-
PDF
- Split View
-
Views
-
Cite
Cite
Quan-Yan Zhang, Kai-Di Gu, Lailiang Cheng, Jia-Hui Wang, Jian-Qiang Yu, Xiao-Fei Wang, Chun-Xiang You, Da-Gang Hu, Yu-Jin Hao, BTB-TAZ Domain Protein MdBT2 Modulates Malate Accumulation and Vacuolar Acidification in Response to Nitrate , Plant Physiology, Volume 183, Issue 2, June 2020, Pages 750–764, https://doi.org/10.1104/pp.20.00208
- Share Icon Share
Abstract
Excessive application of nitrate, an essential macronutrient and a signal regulating diverse physiological processes, decreases malate accumulation in apple (Malus domestica) fruit, but the underlying mechanism remains poorly understood. Here, we show that an apple BTB/TAZ protein, MdBT2, is involved in regulating malate accumulation and vacuolar pH in response to nitrate. In vitro and in vivo assays indicate that MdBT2 interacts directly with and ubiquitinates a bHLH transcription factor, MdCIbHLH1, via the ubiquitin/26S proteasome pathway in response to nitrate. This ubiquitination results in the degradation of MdCIbHLH1 protein and reduces the transcription of MdCIbHLH1-targeted genes involved in malate accumulation and vacuolar acidification, including MdVHA-A, which encodes a vacuolar H+-ATPase, and MdVHP1, which encodes a vacuolar H+-pyrophosphatase, as well as MdALMT9, which encodes an aluminum-activated malate transporter. A series of transgenic analyses in apple materials including fruits, plantlets, and calli demonstrate that MdBT2 controls nitrate-mediated malate accumulation and vacuolar pH at least partially, if not completely, via regulating the MdCIbHLH1 protein level. Taken together, these findings reveal that MdBT2 regulates the stability of MdCIbHLH1 via ubiquitination in response to nitrate, which in succession transcriptionally reduces the expression of malate-associated genes, thereby controlling malate accumulation and vacuolar acidification in apples under high nitrate supply.
Malate, as a key metabolite, plays a vital role in plant metabolism, pH homeostasis, nutrient uptake, osmotic adjustment, and abiotic stress resistance (Fernie and Martinoia, 2009; Finkemeier and Sweetlove, 2009; Bai et al., 2015; Hu et al., 2017). Cellular malate accumulation also largely determines the acidity and perception of sweetness of fleshy fruits and their processed products (Yao et al., 2007; Ye et al., 2017; Butelli et al., 2019; Ma et al., 2019). The majority of malate in the parenchyma cells of fleshy fruits is in the vacuole. Malate accumulation is a complex process involving synthesis, degradation, and transport. Although malate metabolism can alter fruit malate levels (Sweetman et al., 2009; Centeno et al., 2011), it appears that transport of malate from the cytosol into the vacuole is the step that largely controls malate accumulation (Etienne et al., 2013; Hu et al., 2016a; Ma et al., 2019).
Vacuolar proton pumps and malate transporters are crucial for malate transport into the vacuole. Vacuolar proton pumps, such as V-ATPase and V-PPase, acidify the vacuoles by pumping protons across the tonoplast. The resulting low pH protonates any malate that crosses the tonoplast from the cytosol, effectively trapping malate in the acid form. This “acid trap” mechanism maintains the malate concentration gradient across the tonoplast for its facilitated diffusion (Martinoia et al., 2007; Etienne et al., 2013; Eisenach et al., 2014; Hu et al., 2016a). Aluminum-activated malate transporter/channel (ALMT) as well as tonoplast dicarboxylate transporter enables the transport of malate across the tonoplast (Emmerlich et al., 2003; Kovermann et al., 2007; Ma et al., 2015; Ye et al., 2017). ALMT9 underlies a major malic acid locus, Ma, in apple (Malus domestica; Bai et al., 2012, 2015; Khan et al., 2013) and a major QTL for malate levels in tomato (Solanum lycopersicum; Ye et al., 2017).
Upstream of these proton pumps and transporters are various transcription factors (TFs) that regulate their expression. For example, SlWRKY42 directly binds to the promoter and affects transcription of SlAMT9 to negatively regulate malate content in tomato fruit (Ye et al., 2017). Furthermore, the MYB TFs together with bHLH TFs and WD40 proteins form MBW complexes to transcriptionally activate the expression of genes encoding vacuolar proton pumps and malate transporters, thereby promoting malate accumulation in the vacuole (Xie et al., 2012; Hu et al., 2016a, 2017). In apple, MdMYB73 binds directly to the promoters of MdVHA-A, MdVHP1, and MdALMT9 to regulate malate accumulation (Hu et al., 2017). Cold-induced bHLH1 (MdCIbHLH1) interacts with MdMYB73 and enhances its effects on downstream target genes to regulate malate accumulation in apple (Feng et al., 2012; Hu et al., 2017).
Malate accumulation in fleshy fruits is also affected by environmental factors and management practices such as nutrient availability, salinity, temperature, and irrigation (Wu et al., 2002; Burdon et al., 2007; Thakur and Singh, 2012; Etienne et al., 2013). In response to environmental stresses such as low temperature and salinity, plants accumulate malate as a metabolite in coping with these stresses, consequently altering fruit acidity levels (Ruffner et al., 1976; Friemert et al., 1988; Hu et al., 2016b). Nitrate is an essential macronutrient for plant growth and development, and also serves as a signal regulating many physiological processes (Liu et al., 2017; Maeda et al., 2018). High nitrate supply has been reported to reduce malate accumulation (Spironello et al., 2004), but how nitrate specifically affects malate levels remains poorly understood.
The BTB-TAZ proteins respond to diverse environmental stimuli such as nutrients and stress, and internal signals such as hormones in plants (Mandadi et al., 2009). We recently found that BT2, a BTB-TAZ protein, responds to nitrate in modulating anthocyanin synthesis and accumulation in apple (Wang et al., 2018). In this work, we demonstrate that MdBT2 protein regulates malate accumulation in response to nitrate. A yeast two-hybrid (Y2H) screening library allowed for identification of MdCIbHLH1 as a candidate protein for MdBT2 interaction. Subsequently, we uncovered that MdBT2 regulates the stability of MdCIbHLH1 via ubiquitination in response to nitrate, which in succession transcriptionally reduces the expression of several malate-associated genes, thereby altering malate accumulation in apple.
RESULTS
Exogenous Application of Nitrate Reduces Malate Accumulation and Increases Vacuolar pH
To determine if nitrate has any effect on malate accumulation in apple, we provided apple calli and plantlets with different nitrate concentrations (0–5 mm) and measured malate content in various tissues. High concentrations of exogenous nitrate were found to significantly reduce tissue malate accumulation (Supplemental Fig. S1).
To confirm the effect of nitrate on malate accumulation in fruits, we treated ‘Royal Gala’ apple fruits with exogenous nitrate from 0 to 5 mm for further analysis (Fig. 1A). Nitrate content was measured to confirm the effects of exogenous nitrate (Supplemental Fig. S2). Nitrate application led to a significant reduction in fruit malate levels (Fig. 1C). The expression levels of malate-related genes, including vacuolar proton pumps MdVHA-A, MdVHA-Bs, and MdVHP1, the vacuolar malate channel MdALMT9, and the malate-associated MYB TF MdMYB73, were significantly decreased when nitrate concentration increased from 0 to 5 mm (Fig. 1B). Subsequently, we measured the hydrolytic and proton-pumping activities of V-ATPase and V-PPase. V-ATPase hydrolytic activity and proton-pumping activity and V-PPase activity were decreased to 49%, 56%, and 60% of the 0 mm N control, respectively, in the 5 mm N treatment (Fig. 1, D–F). Furthermore, measurements of vacuolar pH with the ratiometric fluorescent pH indicator 2′,7′-bis-(2-carboxyethyl)-5-(6)-carboxyfluorescein (BCECF) showed that the average vacuolar pH was increased from 3.6 in 0 mm N to 3.7 and 4.1, respectively, in the 0.5 and 5 mm N treatments (Fig. 1, G and H). These results suggest that high nitrate levels reduce vacuolar acidification by decreasing the hydrolytic and proton-pumping activities of both V-ATPase and V-PPase in apple.
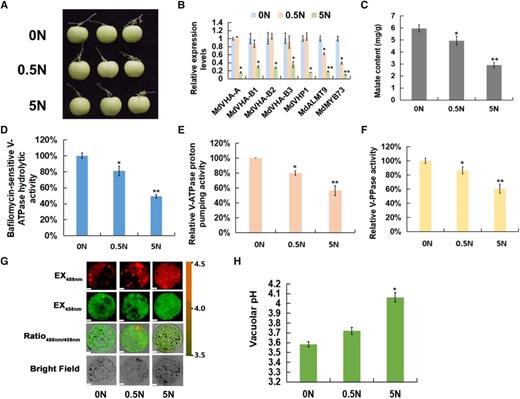
Exogenous application of nitrate reduces malate accumulation and increases the vacuolar pH. A, ‘Royal Gala’ apple fruits were treated with different concentrations of exogenous nitrate (including 0, 0.5, and 5 mm nitrate). B, Reverse transcription quantitative PCR (RT-qPCR) expression analysis of malate-related genes under different concentrations of exogenous nitrate. The genes include vacuolar proton pumps MdVHA-A, MdVHA-Bs, and MdVHP1, the vacuolar malate channel MdALMT9, and the malate-associated MYB TF MdMYB73. C, Malate content under different concentrations of exogenous nitrate. D, Bafilomycin A1-sensitive ATP hydrolytic activity of V-ATPase under different concentrations of exogenous nitrate. E, Proton-pumping activity of V-ATPase under different concentrations of exogenous nitrate was measured by tracking the ATP-dependent quenching of acridine orange using a fluorescence spectrometer with excitation at 493 nm and emission at 545 nm. F, V-PPase activity under different concentrations of exogenous nitrate. G, Emission intensities of protoplast vacuoles under different concentrations of exogenous nitrate loaded with BCECF at 488 nm (first column) and 458 nm (second column). Scale bars = 10 μm. H, Quantification of the pH in vacuoles under different concentrations of exogenous nitrate. For C to F and H, error bars represent the sd from three biological replicates with three technical repetitions. Significant differences were detected by t test (*P < 0.05, **P < 0.01).
BTB/TAZ Protein MdBT2 Controls Malate Accumulation and Vacuolar pH in Response to Nitrate
Considering that the BTB-TAZ protein BT2 responds to nitrate in plants (Mandadi et al., 2009; Wang et al., 2018), we explored the potential role of BT2 in nitrate-modulated malate accumulation. We first looked at the expression of MdBT2 in response to nitrate and found that nitrate application increased its transcript level (Supplemental Fig. S3). We then generated 35S::anti-MdBT2 RNA interference transgenic apple calli (35S::anti-MdBT2; Supplemental Fig. S3), and detected higher malate levels in the antiMdBT2 calli.
To confirm the role of MdBT2 in malate accumulation in response to nitrate, transgenic apple plantlets overexpressing MdBT2 (MdBT2-OX) were treated with 0 mm N (0 N-MdBT2-OX), while antiMdBT2 transgenic apple plantlets (antiMdBT2) were treated with 5 mm N (5N-antiMdBT2), along with wild-type control plantlets under 0 or 5 mm nitrate. Firstly, the nitrate contents were also measured to confirm the effects of exogenous nitrate (Supplemental Fig.e S4). As shown in Figure 2A, 5 mm of nitrate decreased malate accumulation in wild-type plantlets compared with 0 mm nitrate; MdBT2-OX reduced the malate level compared with the wild-type control at 0 mm nitrate, whereas antiMdBT2 increased the malate level compared with the wild-type control at 5 mm nitrate (Fig. 2A).
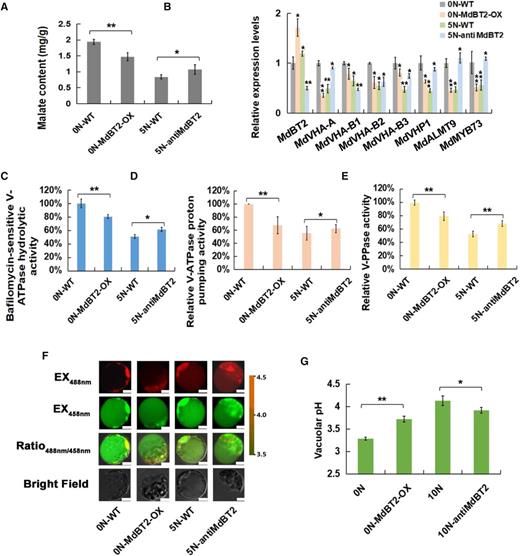
BTB/TAZ protein MdBT2 controls malate accumulation and vacuolar pH in response to nitrate. A, Malate content in wild-type (WT) and transgenic apple plantlets under different concentrations of exogenous nitrate. ‘GL-3’ plantlets were treated with 0 N (0 N-WT). MdBT2 overexpression transgenic apple plantlets were treated with 0 N (0 N-MdBT2-OX). The anti-MdBT2 transgenic apple plantlets were treated with 5 N (5 N-antiMdBT2). B, RT-qPCR expression of MdVHA-A, MdVHP1, and MdALMT9 in wild-type and transgenic apple plantlets under different concentrations of exogenous nitrate. C to E, Hydrolytic and proton-pumping activities of V-ATPase and V-PPase in wild-type and transgenic apple plantlets under different concentrations of exogenous nitrate. F, Emission intensities of protoplast vacuoles loaded with BCECF at 488 and 458 nm. Scale bars = 10 µm. G, Quantification of the pH in vacuoles. For A, C to E, and G, error bars represent the sd from three biological replicates with three technical repetitions. Significant differences were detected by t test (*P < 0.05, **P < 0.01).
A quantity of 5 mm nitrate inhibited the expression of malate-associated genes including MdVHA-A, MdVHA-Bs, MdVHP1, and MdALMT9, and V-ATPase hydrolytic and proton-pumping activities as well as V-PPase activity (Fig. 2, B–E). Overexpression of MdBT2 decreased the expression of these genes and activities of related enzymes and increased vacuolar pH at 0 mm nitrate, whereas antisense repression of MdBT2 increased the expression of the genes and activities of related enzymes and decreased vacuolar pH at 5 mm nitrate (Fig. 2, B–G).
Taken together, these results demonstrate that BTB/TAZ protein MdBT2 modulates malate accumulation and vacuolar pH in response to nitrate in apple.
MdBT2 Physically Interacts with MdCIbHLH1 via the Conserved BTB/BACK Domain
To explore the regulatory mechanism by which MdBT2 is involved in malate accumulation in apple, possible MdBT2-interacting proteins were screened using MdBT2 as a bait through a Y2H complementary DNA (cDNA) library. As a result, the gene MDP0000662999 was chosen because it was a likely candidate for interaction with MdBT2. Previous studies have shown that MDP0000662999, also known as MdCIbHLH1, is involved in malate accumulation (Feng et al., 2012; Hu et al., 2017). To determine whether MdBT2 interacts with MdCIbHLH1 protein, Y2H assays were performed. As MdBT2 protein contains three conserved domains, namely BTB, BACK, and ZnF_TAZ, we divided the full-length cDNA of the MdBT2 gene into four fragments, i.e. MdBT2BTB/BACK, and MdBT2BTB, as well as MdBT2BACK and MdBT2BACK/ZnF_TAZ. Subsequently, the full-length cDNA and four truncated mutants of the MdBT2 gene were inserted into the pGBT9 vector, independently, as bait vectors. Meanwhile, the full-length MdCIbHLH1 cDNA was inserted into pGAD424 as the prey vector. The different combinations of bait and prey vectors were transformed into yeast for Y2H assays. The full-length MdBT2 interacted with the full-length MdCIbHLH1 protein (Fig. 3A). Moreover, MdCIbHLH1 interacted with the truncated mutant MdBT2BTB/BACK, but not with the others (Fig. 3A). These results suggest that MdBT2 in vitro interacts with MdCIbHLH1 via the conserved BTB/BACK domain. In addition, a GST pull-down assay showed that GST-tagged MdBT2 physically interacted with His-tagged MdCIbHLH1 in vitro (Fig. 3B).
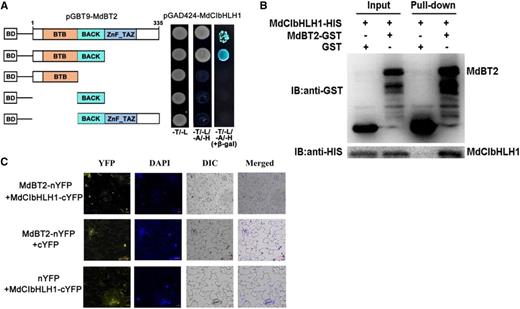
MdBT2 physically interacts with MdCIbHLH1 via the conserved BTB/BACK domain. A, MdBT2 interacted with MdCIbHLH1 in a Y2H assay. The full-length and the BTB/BACK domain of MdBT2 interacted with the MdCIbHLH1 protein. B, Pull-down assays demonstrating the interaction between MdBT2 and MdCIbHLH1. MdBT2-GST was expressed in Escherichia coli, resulting in the precipitation of MdCIbHLH1-HIS using anti-GST and anti-HIS antibodies, respectively. GST alone was used as the control. C, BiFC assay showing the interaction between MdBT2 and MdCIbHLH1 using N. benthamiana leaf cells. MdBT2-nYFP and MdCIbHLH1-cYFP interacted in the nucleus of N. benthamiana leaf cells. Scale bars = 20 μm.
To further confirm the interaction between MdBT2 and MdCIbHLH1, an in vivo bimolecular fluorescence complementation (BiFC) assay using Nicotiana benthamiana leaf cells was conducted. MdBT2 and MdCIbHLH1 were ligated to the N-terminus and C-terminus of yellow fluorescent protein (YFP), respectively, to generate the constructs MdBT2-nYFP and MdCIbHLH1-cYFP. Subsequently, these constructs including empty vectors with pairwise combinations were transiently transformed into leaf cells by the Agrobacterium-mediated method. A strong YFP signal was observed in the nucleus of cells with the combination of MdBT2-nYFP and MdCIbHLH1-cYFP constructs, and no signal was detected in the other two combinations (MdBT2-nYFP+cYFP and nYFP+MdCIbHLH1-cYFP) as negative controls (Fig. 3C). These results indicate that MdBT2 physically interacts with the MdCIbHLH1 protein.
MdCIbHLH1 Is Involved in Regulating Malate Accumulation and Vacuolar pH
It is known that MdCIbHLH1 interacts with MdMYB73 to modulate malate accumulation by directly activating vacuolar transporter genes including MdALMT9, MdVHA-A, and MdVHP1 in apple (Hu et al., 2017). To further verify the functions of MdCIbHLH1 in the regulation of malate accumulation in apple fruits, the 35S::MdCIbHLH1 transgenic apple plantlets (MdCIbHLH1-OVXs) obtained by Feng et al. (2012) were grafted onto rootstock, and transgenic apple fruits were harvested at different stages during fruit development in the third growing season. Determination of malate content revealed that fruits of the three MdCIbHLH1 transgenic lines (MdCIbHLH1-OVXs) had much higher malate levels than the wild-type control (cv GALA) throughout fruit development, particularly at 40 d after flowering (DAF; Fig. 4A). Subsequently, 40-DAF apple fruits were used for further analysis (Fig. 4B).
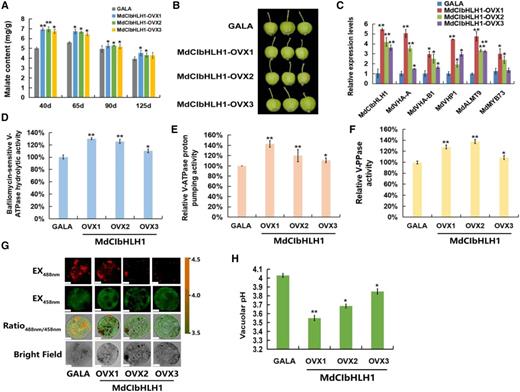
MdCIbHLH1 regulates malate accumulation and vacuolar pH. A, Malate content in ‘GALA’ and MdCIbHLH1-OVXs transgenic apple fruits (MdCIbHLH1-OVXs) in the whole fruit developmental stages. B, ‘GALA’ and MdCIbHLH1-OVXs lines at 40 DAF. C, Expression of MdVHA-A, MdVHA-B1, MdVHP1, MdALMT9, and MdMYB73 in ‘GALA’ and MdCIbHLH1-OVXs. D to F, Hydrolytic and proton-pumping activities of V-ATPase and V-PPase in ‘GALA’ and MdCIbHLH1-OVXs. G, Emission intensities of protoplast vacuoles in ‘GALA’ and MdCIbHLH1-OVXs with BCECF at 488 and 458 nm. Scale bars = 10 µm. H, Quantification of the pH in vacuoles in ‘GALA’ and MdCIbHLH1-OVXs. For A, C, D to F, and H, error bars represent the sd from three biological replicates with three technical repetitions. Significant differences were detected by t test (*P < 0.05, **P < 0.01).
Transcript levels of MdVHA-A, MdVHA-B1, and MdVHP1, as well as MdALMT9 and MdMYB73, were significantly higher in the three independent MdCIbHLH1-OVXs than in cv GALA control fruits (Fig. 4C). V-ATPase and V-PPase activities were 1.2- to 1.7-fold greater in MdCIbHLH1-OVXs than in cv GALA fruits (Fig. 4, D–F). Furthermore, we used BCECF to illustrate the effects of V-ATPase and V-PPase activities on vacuolar pH. As shown in Figure 4, G and H, the average vacuolar pH in cv GALA fruits was 4.03 while those of the three MdCIbHLH1-OVXs were 3.55, 3.69, and 3.85, respectively. Hence, higher vacuolar H+ concentrations in MdCIbHLH1-expressing fruits, indicated by the lower pH values, further supports the conclusion that MdCIbHLH1 regulates malate accumulation by altering tonoplast V-ATPase and V-PPase activities.
MdBT2 Affects the Stability of MdCIbHLH1 Protein
Earlier work showed that the ubiquitination-related MdBT2 scaffold protein targets MYB and bHLH TFs for degradation, thereby regulating plant senescence and anthocyanin biosynthesis (Wang et al., 2018; An et al., 2019). Considering the interaction between MdBT2 and MdCIbHLH1, we predicted that MdBT2 probably affects the stability of MdCIbHLH1 protein. To verify this, cell-free degradation assays with purified recombinant MdCIbHLH1-HIS fusion proteins were conducted using protein samples extracted from wild type, 35S::MdBT2 transgenic apple calli (35S::MdBT2), and 35S::anti-MdBT2. The MdCIbHLH1-HIS protein was more rapidly degraded in the protein extract of 35S::MdBT2 than in that of the wild type (Fig. 5A), whereas the protein was more stable in the protein extract of 35S::anti-MdBT2 compared to the wild type (Fig. 5A). These results suggest that MdBT2 promotes the degradation of the MdCIbHLH1 protein.

MdBT2 affects the stability of MdCIbHLH1 protein. A, Cell-free degradation assays of purified recombinant MdCIbHLH1-HIS fusion proteins using protein samples extracted from WL, 35S::MdBT2, and 35S::anti-MdBT2. WL, ‘Orin’ calli. B, Three types of transgenic calli, 35S::MdCIbHLH1-GFP, 35S::MdBT2-MYC + 35S::MdCIbHLH1-GFP, and 35S::anti-MdBT2 + 35S::MdCIbHLH1-GFP, were obtained and used for immunoblotting assays with an anti-GFP antibody. C, Cell-free degradation assays were also performed using 35S::MdCIbHLH1-GFP and 35S::MdBT2-MYC + 35S::MdCIbHLH1-GFP. Total proteins were analyzed by immunoblotting using the anti-GFP antibody. ACTIN was used as the loading control.
To further examine whether MdBT2 influences the degradation of the MdCIbHLH1 protein in vivo, three types of transgenic calli, 35S::MdCIbHLH1-GFP, 35S::MdBT2-MYC + 35S::MdCIbHLH1-GFP, and 35S::anti-MdBT2 + 35S::MdCIbHLH1-GFP, were obtained and used for immunoblotting assays with an anti-GFP antibody. The abundance of MdCIbHLH1 was lower in 35S::MdBT2-MYC + 35S::MdCIbHLH1-GFP, but higher in 35S::anti-MdBT2 + 35S::MdCIbHLH1-GFP, than in the wild type (Fig. 5B). Meanwhile, cell-free degradation assays were also performed using 35S::MdCIbHLH1-GFP and 35S::MdBT2-MYC + 35S::MdCIbHLH1-GFP. As shown in Figure 5C, the MdCIbHLH1-GFP protein in 35S::MdBT2-MYC + 35S::MdCIbHLH1-GFP was degraded to a much greater extent than in 35S::MdCIbHLH1-GFP, just as they did in vitro.
MdBT2 Is Involved in the Ubiquitination and Degradation of MdCIbHLH1 Proteins
To examine whether MdCIbHLH1 is degraded through a 26S proteasomal pathway, 35S::MdCIbHLH1-GFP treated with the translational inhibitor cycloheximide (CHX) and the proteasomal inhibitor MG132 were used for immunoblotting assays with an anti-GFP antibody. The results showed that MdCIbHLH1 protein accumulation was almost completely inhibited by CHX in the 35S::MdCIbHLH1-GFP (Fig. 6A). By contrast, MdCIbHLH1 protein highly accumulated in the transgenic calli treated with MG132 (Fig. 6A), suggesting that the MdCIbHLH1 protein is degraded in a 26S proteasome-dependent manner.
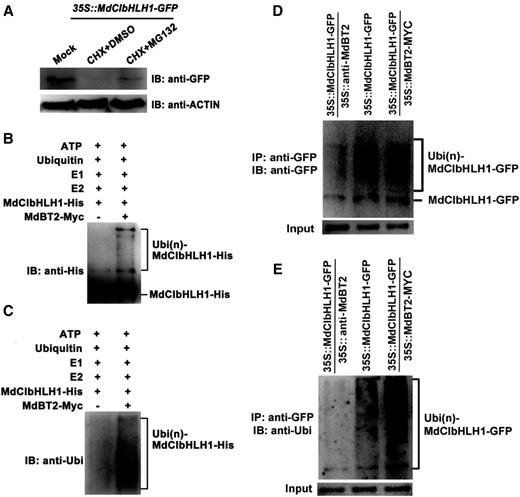
MdBT2 is involved in the ubiquitination and degradation of MdCIbHLH1 proteins. A, 35S::MdCIbHLH1-GFP plants were treated with CHX and MG132 for 6 h, after which MdCIbHLH1-GFP protein levels were measured using the anti-GFP antibody. B to E, MdBT2 promoted the ubiquitination of MdCIbHLH1 protein in vitro and in vivo. B and C, The MdBT2-MYC active protein extracted from 35S::MdBT2-MYC was coincubated with purified recombinant MdCIbHLH1-HIS protein, E1, E2, and ubi in vitro for immunoprecipitation using anti-HIS and anti-ubi antibodies. D and E, In vivo ubiquitination was measured using 35S::MdCIbHLH1-GFP, 35S::MdCIbHLH1-GFP + 35S::MdBT2-MYC, and 35S::MdCIbHLH1-GFP + 35S::anti-MdBT2. The anti-GFP and anti-ubi antibodies were used to detect MdCIbHLH1-GFP protein accumulation. IP, Immunoprecipitated; IB, immunoblotted.
26S proteasome-mediated protein degradation is generally associated with ubiquitination modifications. To examine if MdBT2 participates in the ubiquitination of the MdCIbHLH1 protein, the MdBT2-MYC active protein extracted from 35S::MdBT2-MYC was coincubated with purified recombinant MdCIbHLH1-HIS protein, E1, E2, and ubiquitin (ubi) in vitro for immunoprecipitation using anti-HIS and anti-ubi antibodies. A higher amount of high-molecular mass forms of MdCIbHLH1, that is, polyubiquitinated MdCIbHLH1 (Ubi[n]-MdCIbHLH1–HIS), was detected when supplemented with MdBT2-MYC active protein (Fig. 6, B and C). Therefore, it seems that MdBT2 is essential for the ubiquitination of MdCIbHLH1 proteins.
Subsequently, in vivo ubiquitination assays were conducted using 35S::MdCIbHLH1-GFP, 35S::MdCIbHLH1-GFP + 35S::MdBT2-MYC, and 35S::MdCIbHLH1-GFP + 35S::anti-MdBT2 (Fig. 6, D and E). The anti-GFP antibody and anti-ubi antibodies were used to detect MdCIbHLH1-GFP protein accumulation. The abundance of polyubiquitinated MdCIbHLH1-GFP was greater in 35S::MdCIbHLH1-GFP + 35S::MdBT2-MYC than in 35S::MdCIbHLH1-GFP, but lower in 35S::MdCIbHLH1-GFP + 35S::anti-MdBT2 than in 35S::MdCIbHLH1-GFP (Fig. 6, D and E). These results suggest that the ubiquitination and degradation of the MdCIbHLH1 protein was mediated by MdBT2.
Nitrate Participates in the Regulation of MdBT2-Mediated MdCIbHLH1 Protein Stability
To determine whether nitrate is involved in the regulation of MdBT2-mediated MdCIbHLH1 protein stability, 35S::MdCIbHLH1-GFP calli were provided with nitrate (+N) or without nitrate (−N). The total proteins extracted from these calli samples were then used for immunoblotting assays with anti-GFP antibody. Degradation of the MdCIbHLH1 protein was accelerated in the presence of nitrate (Fig. 7A). In contrast, the MdCIbHLH1 proteins gradually accumulated in the absence of nitrate (Fig. 7A). These results suggest that nitrate facilitates the degradation of MdCIbHLH1.
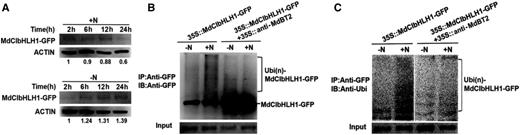
Nitrate participates in the regulation of MdBT2-mediated MdCIbHLH1 protein stability. A, Protein stability of MdCIbHLH1 in response to nitrate. 35S:: MdCIbHLH1-GFP calli were incubated without nitrate (−N) and with nitrate (+N), respectively. The total proteins extracted from these calli samples were then used for immunoblotting (IB) assays using the anti-GFP antibody. B and C, IB assays were conducted using 35S::MdCIbHLH1-GFP treated with or without nitrate. IP, Immunoprecipitated.
To examine the effect of nitrate on MdCIbHLH1 protein ubiquitination, immunoblotting assays were conducted using 35S::MdCIbHLH1-GFP treated with or without nitrate. Exogenous nitrate treatment accelerated the ubiquitination of MdCIbHLH1 in 35S::MdCIbHLH1-GFP, while MdCIbHLH1 protein ubiquitination was alleviated in 35S::MdCIbHLH1-GFP + 35S::anti-MdBT2 (Fig. 7B). These results suggest that nitrate is involved in the regulation of MdBT2-mediated MdCIbHLH1 protein ubiquitination.
In addition, the level of ubiquitinated MdCIbHLH1 protein was also assessed using an anti-ubi antibody that recognizes only ubiquitinated MdCIbHLH1 protein. Similarly, the ubiquitin signal was substantially enhanced in 35S::MdCIbHLH1-GFP treated with nitrate compared with the absence of nitrate, whereas the ubiquitin signal was reduced in 35S::MdCIbHLH1-GFP + 35S::anti-MdBT2 (Fig. 7C).
Overall, these results suggest that nitrate acting as a signal regulates MdBT2-mediated MdCIbHLH1 protein stability.
MdCIbHLH1 Is Required for MdBT2 To Modulate Malate Accumulation
To investigate whether MdBT2 and MdCIbHLH1 regulate malate accumulation in apple, a viral vector-based method was applied to alter their expression using vector TRV for suppression. Two viral constructs, including MdBT2-TRV and MdCIbHLH1-TRV, were obtained. These constructs including MdBT2-TRV and MdCIbHLH1-TRV, as well as the double combination MdBT2-TRV + MdCIbHLH1-TRV were used for fruit infiltration, with the empty vectors as controls (Fig. 8A). RT-qPCR assays showed that the transcript levels of MdBT2 and MdCIbHLH1 genes were significantly decreased after being infiltrated with MdBT2-TRV and MdCIbHLH1-TRV (Fig. 8B).
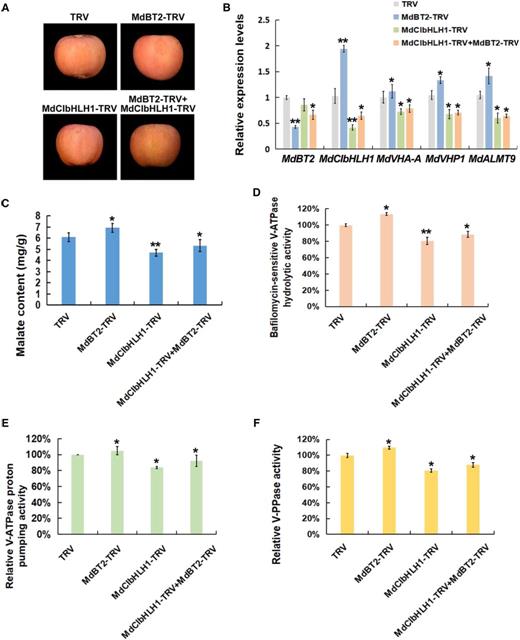
MdCIbHLH1 is required for MdBT2 to modulate malate accumulation. A, Viral vector-based method was applied to the apple injection assays. The TRV1 vector was used as an auxiliary plasmid. MdBT2-TRV and MdCIbHLH1-TRV were obtained by cloning the coding sequences of MdBT2 and MdCIbHLH1 into the TRV2 vector, respectively, and mixed with TRV1. The TRV vector (TRV1 + TRV2) was used as control. B, RT-qPCR expression analysis of MdVHA-A, MdVHP1, and MdALMT9. C to F, Malate content and hydrolytic and proton-pumping activities of V-ATPase and V-PPase were measured in apple fruit tissues around the sites infiltrated with the different viral constructs. Error bars represent the sd from three biological replicates with three technical repetitions. Significant differences were detected by t test (*P < 0.05, **P < 0.01).
In response to suppression of MdCIbHLH1, transcript levels of MdCIbHLH1-targeted genes involved in malate accumulation including MdVHA-A, MdVHP1, and MdALMT9, were decreased. By contrast, the transcripts of these genes were increased in MdBT2-TRV injected apple fruits (Fig. 8B). Subsequently, malate levels, hydrolytic and proton-pumping activities of V-ATPase, and V-PPase activity were measured in apple fruit tissues. MdCIbHLH1-TRV injected apple fruits had lower malate levels and lower proton-pumping activities than the TRV control, whereas MdBT2-TRV injected apple fruits produced higher malate levels and proton-pumping activities than the control (Fig. 8, C–F). In the MdBT2-TRV + MdCIbHLH1-TRV double-injected apple fruits, malate levels and proton-pumping activities were obviously decreased compared with the TRV control, consistent with MdCIbHLH1-TRV. MdCIbHLH1 suppression abolished the effect of MdBT2-TRV infiltration on malate accumulation and proton-pumping activities, indicating that MdBT2 controls malate accumulation at least partially, if not entirely, via MdCIbHLH1 in apple. Similar results were obtained in MdBT2 and MdCIbHLH1 transgenic apple plantlets (Supplemental Fig. S5).
In earlier work, we showed that MdCIbHLH1 interacts with and activates MdMYB73 to modulate malate accumulation and vacuolar acidification by directly activating MdALMT9 in apples (Hu et al., 2017). Because MdBT2 mediates the ubiquitination and degradation of the MdCIbHLH1 protein, it is reasonable to predict that MdBT2 influences the transcription level of the MdMYB73-downstream gene MdALMT9. To verify this hypothesis, we fused the GUS coding region to the MdALMT9 promoter, and performed GUS assays to determine the effect of MdBT2 on the transcriptional activity of MdALMT9 (Supplemental Fig. S6). The MdBT2-MdCIbHLH1 regulatory module negatively regulates GUS transcription of MdMYB73-downstream gene MdALMT9, which is driven by the MdALMT9 promoter.
Further, a viral vector-based method was also applied to show that the MdBT2-MdCIbHLH1 regulatory module controls malate accumulation through influencing the MdMYB73-downstream gene MdALMT9 (Supplemental Fig. S7). These constructs including TRV, MdALMT9-TRV, MdCIbHLH1-TRV + MdALMT9-TRV, and the triple combination MdCIbHLH1-TRV + MdALMT9-TRV + MdBT2-TRV were used for fruit infiltration. Compared with MdALMT9-TRV, malate levels and activities of V-ATPase and V-PPase were decreased as expected in MdCIbHLH1-TRV + MdALMT9-TRV. In MdCIbHLH1-TRV + MdALMT9-TRV + MdBT2-TRV injected apple fruits, malate levels and activities of V-ATPase and V-PPase were increased compared to MdCIbHLH1-TRV + MdALMT9-TRV (Supplemental Fig. S7), indicating that the MdBT2-MdCIbHLH1 regulatory module controls malate accumulation through influencing the MdMYB73-downstream gene MdALMT9 in apple.
Taken together, these results further demonstrate that MdCIbHLH1 is required for MdBT2 to modulate malate accumulation.
DISCUSSION
Malate is a crucial metabolite regulated by various signaling pathways that integrate responses of gene expression to environmental factors such as nutrient availability. Physiological studies have demonstrated that malate and nitrate have intricate regulation mechanisms during plant growth and development (Stitt, 1999; Etienne et al., 2013). Although a connection between nitrate and malate has long been speculated, the exact molecular mechanism has remained unclear. Some reports suggest that nitrogen positively regulates fruit acidity (Reitz and Koo, 1960; Ruhl, 1989; Jia et al., 1999; Radi et al., 2003), while others have shown a negative relationship between nitrate level and fruit acidity (Spironello et al., 2004; Wang et al., 2010). This study reveals that high nitrate inhibits malate accumulation in apple (Fig. 1; Supplemental Fig. S1). MdBT2, a nitrate-responsive protein, which was shown to be involved in regulating anthocyanin accumulation through interacting with MdMYB1 in our earlier work (Wang et al., 2018), plays a key role in modulating malate accumulation in response to nitrate. MdBT2 interacts with and ubiquitinates MdCIbHLH1 in response to nitrate (Figs. 2–8), providing the molecular link between nitrate availability and malate accumulation.
BT2 is a scaffold protein that recruits CUL3 protein to form the E3 ligase complex or bridges an unknown ubiquitin E3 ligase to ubiquitinate and degrade the target proteins (Robert et al., 2009; Zhao et al., 2016; Wang et al., 2018). MdCUL3 protein is required for MdBT2-mediated ubiquitination and degradation of MdbHLH104 for iron homeostasis in apple, with the TAZ domain of MdBT2 being essential for the interaction (Zhao et al., 2016). However, MdBT2 promotes the ubiquitination and degradation of MdMYB1 in regulating anthocyanin biosynthesis through an MdCUL3-independent pathway, while the BACK domain of MdBT2 is indispensable for the interaction (Wang et al., 2018). In this study, both BTB domain and BACK domain are required for the interaction between MdBT2 and MdCIbHLH1 (Fig. 3). Based on these findings, we speculate that MdBT2 ubiquitinates MdCIbHLH1 through an MdCUL3-independent pathway.
In apple, MdCIbHLH1, an ICE1-like protein, regulates cold tolerance in a CBF-dependent manner (Feng et al., 2012). The ICE1-CBF-COR cold response pathway is one of the dominating cold signaling modules that mediates the cold tolerance response in Arabidopsis (Arabidopsis thaliana; Chinnusamy et al., 2003; Shi et al., 2018). It has been known that malate accumulation is affected by temperature. High temperature reduces malate accumulation in fruit (Buttrose et al., 1971; Lobit et al., 2006; Etienne et al., 2013), whereas low temperature facilitates vacuolar malate accumulation probably by affecting proton pump transport activity and membrane fluidity (Murata and Los, 1997; Terrier et al., 2001; Etienne et al., 2013). As malate serves as an osmoticum for plant resistance to low temperature (Ruffner et al., 1976; Friemert et al., 1988; Kovermann et al., 2007) and salinity (Hu et al., 2016b), our previous (Hu et al., 2017) and current work strongly suggest that MdCIbHLH1 regulates plant cold tolerance via more than one pathway. In addition to the CBF-dependent pathway, MdCIbHLH1 controls cold tolerance via regulating malate accumulation by the MdCIbHLH1-MdMYB73 pathway, which was described in our previous study Hu et al. (2017).
Vacuolar proton pumps could acidify the vacuoles by pumping protons across the tonoplast and the resultant low vacuolar pH condition protonates any malate that is transported into the vacuole. Moreover, malate transporters (such as MdALMT9) could transport malate from the cytosol into the vacuole (Hu et al., 2016a; Li et al., 2020). Thus, vacuolar proton pumps and malate transporters are crucial for influencing malate accumulation in the vacuole and vacuolar acidification. We believe that MdBT2 regulates malate accumulation, V-ATPase activities, and vacuolar pH through malate transporter and vacuolar proton pumps. Because MdBT2 controls malate accumulation, the activities of vacuolar proton pumps, and vacuolar pH (Fig. 2; Supplemental Fig. S3), the GUS assays in Supplemental Figure S6, and viral vector-based fruit infiltration in Supplemental Figure S7 show that the MdBT2-MdCIbHLH1 regulatory module controls the transport of malate into the vacuole through at least partially influencing the MdALMT9 gene. Moreover, our previous studies showed that MdCIbHLH1 interacts with MdMYB73 to modulate malate accumulation and vacuolar pH by regulating the activities of vacuolar proton pumps (Hu et al., 2017). In this study, MdBT2 interacted with MdCIbHLH1 (Fig. 3). Therefore, MdBT2 controls the activities of vacuolar proton pumps via the MdCIbHLH1-MdMYB73 regulatory module to influence malate accumulation and vacuolar pH. Taken together, our findings show that MdBT2 regulates malate accumulation and vacuolar pH through malate transporter and vacuolar proton pumps, but we cannot rule out that vacuolar acidification could affect MdALMT9.
Malate accumulation involves transcriptional regulation of genes encoding vacuolar proton pumps and malate transporters (Xie et al., 2012; Hu et al., 2017; Li et al., 2017). It has been reported that MdbHLH3 plays a central role in the accumulation of both malate and anthocyanins by interacting with MdMYB1 and binding to the promoter of MdMYB1 (Xie et al., 2012; Hu et al., 2016a). MdCIbHLH1 interacts with MdMYB73 and enhances its transcriptional activity on the downstream target genes to control malate accumulation (Figs. 4 and 8; Supplemental Fig. S6; Hu et al., 2017). In both cases, it is proposed that the MBW complex is recruited to regulate malate accumulation. However, the target proteins of MdbHLH3 and MdCIbHLH1 differ in their functions in regulating malate versus anthocyanin accumulation. In this study, it was found that MdBT2 interacted with MdCIbHLH1 to modulate V-ATPase activities and malate accumulation (Figs. 3 and 8; Supplemental Figs. S5–S7). Meanwhile, MdBT2 also interacts with MdMYB1 to regulate V-ATPase activities and malate accumulation (Hu et al., 2016a; Wang et al., 2018). Therefore, these findings suggest that MdBT2 regulates V-ATPase activities and malate accumulation by regulating MdCIbHLH1, MdMYB1, and possibly some other unknown TFs. Thus, MdBT2 is proposed to control V-ATPase activities and malate accumulation by regulating several TFs, especially components of the MBW complex. Additionally, recent studies found that Noemi, encoding a bHLH transcription factor, controls two coevolved traits in citrus, fruit acidity, and anthocyanin levels (Butelli et al., 2019; Zhu et al., 2019). Our findings here suggest that Noemi might regulate fruit acidity and anthocyanin levels via a pathway similar to MdbHLH3-MdMYB1 (Xie et al., 2012; Hu et al., 2016a).
Based on the findings presented in this work and elsewhere (Hu et al., 2017), we propose a working model for the regulation of malate accumulation by nitrate (Fig. 9). In this model, when not ubiquitinated under nitrate deficiency, MdCIbHLH1 enhances the activity of MdMYB73, which maintains the transcription of malate-associated genes at a high level, resulting in high malate accumulation. However, MdBT2 ubiquitinates and degrades MdCIbHLH1 in response to high nitrate, which reduces the transcription of malate-associated genes by decreasing the activity of MYB73, leading to lower malate accumulation. Our findings provide valuable insights into the molecular mechanism by which nitrate affects malate accumulation and fruit quality, providing an important link between two key metabolites in plant metabolism. These findings not only help us understand the complex regulatory network of malate accumulation but also are of great significance in guiding molecular breeding programs as well as traditional apple breeding programs for fruit quality improvement.
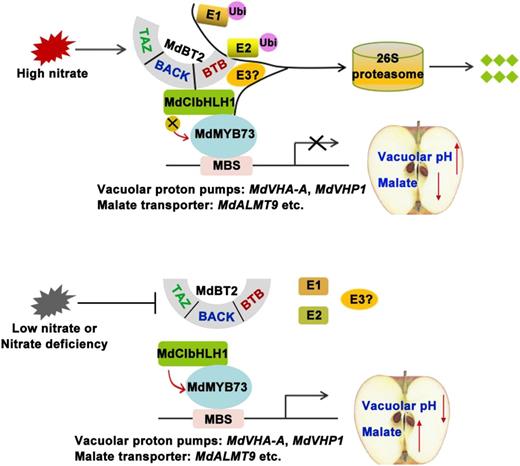
Working model demonstrating that MdBT2 modulates malate accumulation and vacuolar acidification in response to nitrate. Bottom, Low nitrate or nitrate deficiency; MdCIbHLH1 enhances the activity of MdMYB73, which maintains the transcription of malate-associated genes at a high level, resulting in high malate accumulation and lower vacuolar pH. Top, MdBT2 ubiquitinates and degrades MdCIbHLH1 in response to high nitrate, which reduces the transcription of malate-associated genes by decreasing the activity of MYB73, leading to lower malate accumulation and high vacuolar pH. MBS, MYB-binding cis-elements.
MATERIALS AND METHODS
Plant Materials and Treatments
‘GL-3’ (Malus domestica ‘Royal Gala’) apple plantlets, ‘Royal Gala’ apple fruits, and ‘Orin’ calli were used. The ‘GL3’ and transgenic apple plantlets were grown on Murashige-Skoog (MS) medium containing 0.2 mg L−1 of naphthylacetic acid, 0.2 mg L−1 of gibberellin acid, and 0.6 mg L−1 of 6-benzylaminopurine at 25°C under long-day conditions (16-h light/8-h dark). ‘Orin’ apple calli were grown on MS medium with 1.5 mg L−1 of 2,4-dichlorophenoxyacetic acidrev, and 0.4 mg L−1 of 6-benzylaminopurine at 25°C in the dark. For phenotypic analyses of the nitrate-deficiency response, the ‘GL-3’ cultures and ‘Orin’ apple calli were transferred from nitrate-sufficient medium (MS medium with the indicated amount of KNO3, 5 mm) to nitrate-deficient medium (MS medium without nitrogen, followed by 5 mm of KCl as control), and then grown for 3 d.
Three MdCIbHLH1 overexpression lines, MdCIbHLH1-OVX1, MdCIbHLH1-OVX2, MdCIbHLH1-OVX3, and wild-type control trees were planted in the field at an experimental farm in April 2012. These apple trees started to bear fruits from 2015. At least 30 apple fruits were taken from each of the three MdCIbHLH1 overexpression lines and wild-type control trees for phenotypic characterization and other determination.
Plasmid Construction and Genetic Transformation
To construct the MdCIbHLH1 overexpression vector, the full-length cDNA of MdCIbHLH1 was isolated from ‘Gala’ apples using RT-PCR. The cDNA was inserted into T-system vector pCXCN-GFP and pCXCN-Myc, respectively. For transformation of apple tissue cultures, the recombinant plasmids 35S::MdCIbHLH1-GFP and 35S::MdCIbHLH1-Myc were introduced into ‘Gala’ apple tissue cultures and apple calli, respectively, according to the method described by Horsch et al. (1985) and Hu et al. (2019). The amplification primers are listed in Supplemental Table S1. For plasmids, construction of MdBT2-OVX and antiMdBT2, and their transformation into apple calli, have been described by An et al. (2018).
Measurement of Malate Contents
Samples were extracted with 95% (v/v) ice-cold methanol first and then 80% (v/v) ice-cold methanol after being frozen and ground in liquid nitrogen. The extracts were evaporated to dryness, dissolved in deionized water, and then centrifuged at 4,000g. The supernatants were filtered through a 0.45-μm membrane filter and then analyzed by high performance liquid chromatography as described by Hu et al. (2016a).
Activity Assays of V-ATPase and V-PPase
Isolation of tonoplast membranes was performed as described by Terrier et al. (2001). The bafilomycin A1-sensitive ATP hydrolytic activity and V-ATPase H+ transport activity, as well as V-PPase activity, were measured as described by Hu et al. (2016a, 2017).
Protoplasts Isolation and Measurement of Vacuolar pH
The isolation of apple protoplasts was performed as described by Sun et al. (2017). The resultant protoplasts were then measured by vacuolar pH by using BCECF-acetoxymethyl ester (Molecular Probes) as described by Tang et al. (2012) and Hu et al. (2016a) with some optimized modifications. When cells were grown at too low pH, BCECF dye could not be encapsulated into the vacuoles and provoked an accumulation in the cytoplasm. Therefore, on the one hand, we increased the dye incubation time up to 6 h; on the other hand, we adjusted the osmotic potential (upon exposure to hypotonic medium [10 mm of EGTA, 0.8 m of Mannitol, 1 m of MgCl2, 0.3 m of MES, and 10 μm of BCECF-acetoxymethyl ester adjusted to 200 mOsmol kg−1 with sorbitol]) of the solution. After incubation, the protoplasts were washed two times with W5 solution (5 m of NaCl, 1 m of CaCl2, 1 m of KCl, and 0.3 m of MES). These protoplasts were then kept in WI solution (0.8 m of Mannitol, 1 m of MgCl2, and 0.3 m of MES), and used for vacuolar pH determination. The vacuolar pH was quantified by the ratio of 488-nm and 458-nm excitation wavelengths. The ion concentration tool of the LSM Confocal Microscope software (Zeiss) was used to generate the ratio images.
RT-qPCR Analysis
Total RNA was extracted from apple flesh, plantlets, and calli using RNA Plant Plus Reagent (Tiangen) and RT-qPCR analysis was performed as described in Hu et al. (2019). The primers used for RT-qPCR assays are listed in Supplemental Table S1.
Y2H Assay
Y2H assays were performed as described by Wang et al. (2018). The full-length cDNA of MdCIbHLH1 was amplified and inserted into pGAD424, while MdBT2 cDNAs and truncated sequences (amino acids 1–335, 1–195, 1–128, 129–195, and 129–335) were amplified and inserted into pGBT9. The plasmids of pGAD424-MdCIbHLH1 and pGBT9-MdBT2s were cotransformed into Y2H Gold (Clontech). The yeast strains were grown on -l/-T selection medium for the transformation control and on -L/-T/-H/-A selection medium with or without X-gal for the interaction analysis.
Pull-Down Assays
The full-length cDNA of MdCIbHLH1 was amplified by PCR and inserted into pET-32a to generate HIS-tagged recombinant protein (MdCIbHLH1-HIS). The full-length cDNA of MdBT2 was also amplified by PCR and cloned into pGEX-4T to produce GST-tagged fusion protein (MdBT2-GST). The fusion proteins of MdCIbHLH1-HIS, MdBT2-GST, and empty GST were used for the pull-down assays according to Hu et al. (2019).
BiFC Assays
The full-length cDNA of MdBT2 was inserted into the vector 35S::pSPYNE-nYFP (MdBT2-nYFP) and MdCIbHLH1 was inserted into the vector 35S::pSPYCE-cYFP (MdCIbHLH1-cYFP). Solutions of Agrobacterium harboring recombinant plasmids (MdBT2-nYFP + MdCIbHLH1-cYFP) were injected into Nicotiana benthamiana leaves. The empty vectors (MdBT2-nYFP + cYFP and nYFP + MdCIbHLH1-cYFP) were used as negative controls. Imaging of YFP fluorescence in tobacco cells was conducted under a laser scanning confocal microscope (model no. LSM510; Zeiss). The excitation wavelength used for YFP was 488 nm, and the emission filter wavelength was 520 to 550 nm. For each experiment, at least eight different samples were examined under the laser scanning microscope. Experiments were repeated three times.
Protein Degradation and Ubiquitination Assays
For the degradation assays of the MdCIbHLH1 protein in vitro, apple calli (35S::MdBT2, ‘Orin’ calli [WL], and 35S::anti-MdBT2) and the MdCIbHLH1-HIS fusion protein were prepared. The extraction buffer contained 25 mm of Tris at pH 7.5, 5 mm of dithiothreitol, 10 mm of NaCl, 10 mm of MgCl2, 4 mm of phenylmethanesulfonyl fluoride, and 10 mm of ATP. The MdCIbHLH1-HIS fusion protein and apple calli were extracted and incubated at 22°C. Samples were collected at the indicated time (0, 1, 2, 4, and 6 h) and examined using an anti-HIS antibody. For the proteasome inhibitor experiments, 50 μm of MG132 was added. For the degradation assays of the MdCIbHLH1 protein in vivo, two types of apple calli (35S::MdCIbHLH1-GFP and 35S::MdCIbHLH1-GFP + 35S::MdBT2-MYC) were prepared. Total proteins were monitored at the indicated times (0, 1, 2, 4, and 6 h) when 250 mm of CHX was added, and then the anti-GFP antibody was used for immunoblotting assays.
For the ubiquitination assays in vivo, three types of apple calli (35S::MdCIbHLH1-GFP, 35S::MdCIbHLH1-GFP + 35S::MdBT2-MYC, and 35S::MdCIbHLH1-GFP + 35S::anti-MdBT2) were prepared and treated with 50 µm of MG132 for 10 h before extraction. The protein extracts were immunoprecipitated using a Pierce Classic Protein A IP Kit (Thermo Fisher Scientific) with anti-GFP and anti-ubi antibodies. For the ubiquitination assays in vitro, 35S::MdBT2-MYC apple calli were treated with 50 μm of MG132 for 10 h to obtain the MdBT2-MYC active protein using Pierce Classic Protein A IP Kit. The incubation buffer contained 50 mm of Tris at pH 7.5, 2 mm of dithiothreitol, 50 mm of MgCl2, 2 mm of ATP, 100 ng of rabbit E1, 100 ng of human E2, and 1 μg of ubi. The buffer and MdCIbHLH1-HIS protein with or without MdBT2-MYC active protein in vivo were coincubated at 30°C for 24 h. Protein ubiquitination was detected using anti-HIS and anti-ubi antibodies.
Apple Injection Assays
Fruit injection assays were carried out as described in Hu et al. (2016a). The coding sequences of MdBT2 and MdCIbHLH1 were amplified and inserted into viral vector to obtain MdBT2-TRV (TRV1 + MdBT2-TRV2), MdCIbHLH1-TRV (TRV1 + MdCIbHLH1-TRV2), and MdBT2-TRV + MdCIbHLH1-TRV (TRV1 + MdBT2-TRV2 + MdCIbHLH1-TRV2), with the TRV vector (TRV1 + TRV2) used as control. The mixture of vectors and the Agrobacterium tumefaciens solutions were injected into apple fruit.
Statistical Analysis
The data were performed for three biological replicates and three technical repetitions. Significant difference was detected by t test using Graphpad PRISM 6.02 software (*P < 0.05; **P < 0.01).
Accession Numbers
Sequence data from this article can be found in the Genome Database for Rosaceae (GDR; https://www.rosaceae.org) data libraries under accession numbers MdBT2 (MDP0000643281), MdCIbHLH1 (MDP0000662999), MdMYB73 (MDP0000894463), MdVHA-A (MDP0000248012), MdVHA-B1 (MDP0000945182), MdVHA-B2 (MDP0000631168), MdVHA-B3 (MDP0000205940), and MdVHP1 (MDP0000688191).
Supplemental Data
The following supplemental materials are available.
Supplemental Figure S1. Malate content under different concentrations of exogenous nitrate in apple.
Supplemental Figure S2. Nitrate content under different concentrations of exogenous nitrate in apple fruits.
Supplemental Figure S3. BTB/TAZ protein MdBT2 controls malate accumulation and vacuolar pH in response to nitrate.
Supplemental Figure S4. Nitrate content under transgenic apple plantlets treated with different nitrate concentrations.
Supplemental Figure S5. MdCIbHLH1 is required for MdBT2 to modulate malate accumulation in response to nitrate.
Supplemental Figure S6. The MdBT2-MdCIbHLH1 regulatory module negatively regulates MdMYB73-downstream gene MdALMT9.
Supplemental Figure S7. The MdBT2-MdCIbHLH1 regulatory module controls malate accumulation through influencing the MdMYB73-downstream gene MdALMT9.
Supplemental Table S1. The primers used in this study.
ACKNOWLEDGMENTS
We thank Takaya Moriguchi (National Institute of Fruit Tree Science, Japan) for ‘Orin’ apple calli.
LITERATURE CITED
Author notes
This work was supported by the National Key Research and Development Program of China (no. 2018YFD1000200), the National Natural Science Foundation of China (nos. 31972375 and 31772288), the Ministry of Agriculture of China (no. CARS–27), Shandong Province (no. SDAIT–06–03), and Nanjing Agricultural University (no. ZW201805).
Articles can be viewed without a subscription.
Senior authors.
The author responsible for distribution of materials integral to the findings presented in this article in accordance with the policy described in the Instructions for Authors (www.plantphysiol.org) is: Yu-Jin Hao ([email protected]).
Y.-J.H. and D.-G.H. planned and designed the research; Q.-Y.Z., K.-D.G., J.-H.W., J.-Q.Y., X.-F.W., and C.-X.Y. performed experiments, conducted fieldwork, and analyzed data; Q.-Y.Z., D.-G.H., Y.-J.H., and L.C. wrote the article.