-
PDF
- Split View
-
Views
-
Cite
Cite
Michael Taleski, Kelly Chapman, Nijat Imin, Michael A. Djordjevic, Michael Groszmann, The Peptide Hormone Receptor CEPR1 Functions in the Reproductive Tissue to Control Seed Size and Yield , Plant Physiology, Volume 183, Issue 2, June 2020, Pages 620–636, https://doi.org/10.1104/pp.20.00172
- Share Icon Share
Abstract
The interaction of C-TERMINALLY ENCODED PEPTIDES (CEPs) with CEP RECEPTOR1 (CEPR1) controls root growth and development, as well as nitrate uptake, but has no known role in determining yield. We used physiological, microscopic, molecular, and grafting approaches to demonstrate a reproductive tissue-specific role for CEPR1 in controlling yield and seed size. Independent Arabidopsis (Arabidopsis thaliana) cepr1 null mutants showed disproportionately large reductions in yield and seed size relative to their decreased vegetative growth. These yield defects correlated with compromised reproductive development predominantly in female tissues, as well as chlorosis, and the accumulation of anthocyanins in cepr1 reproductive tissues. The thinning of competing reproductive organs to improve source-to-sink ratios in cepr1, along with reciprocal bolt-grafting experiments, demonstrated that CEPR1 acts locally in the reproductive bolt to control yield and seed size. CEPR1 is expressed throughout the vasculature of reproductive organs, including in the chalazal seed coat, but not in other seed tissues. This expression pattern implies that CEPR1 controls yield and seed size from the maternal tissue. The complementation of cepr1 mutants with transgenic CEPR1 rescued the yield and other phenotypes. Transcriptional analyses of cepr1 bolts showed alterations in the expression levels of several genes of the CEP-CEPR1 and nitrogen homeostasis pathways. This transcriptional profile was consistent with cepr1 bolts being nitrogen deficient and with a reproductive tissue-specific function for CEP-CEPR1 signaling. The results reveal a local role for CEPR1 in the maternal reproductive tissue in determining seed size and yield, likely via the control of nitrogen delivery to the reproductive sinks.
Leucine-rich repeat receptor-like kinases (LRR-RLKs) are one of the largest gene families in plants, comprising more than 220 members in Arabidopsis (Arabidopsis thaliana; Gou et al., 2010). Research over the past decade has implicated LRR-RLKs and their selective interactions with secreted peptide hormones in myriad developmental processes, including reproduction, chemotropism, biotic and abiotic stress tolerances, symbiosis, root architecture, regulation of organ number, stomatal function and development, abscission, and general interactions with the environment (Czyzewicz et al., 2013; Delay et al., 2013a; Djordjevic et al., 2015; Santiago et al., 2016; Shabala et al., 2016; Shinohara et al., 2016; Imin et al., 2018; Roy et al., 2018; Chapman et al., 2020).
A conserved LRR-RLK with a growing list of important roles is C-TERMINALLY ENCODED PEPTIDE RECEPTOR1 (CEPR1) in Arabidopsis and its functional ortholog COMPACT ROOT ARCHITECTURE2 (CRA2) in Medicago truncatula. In Arabidopsis, CEPR1’s ectodomain specifically interacts with peptide hormones of the C-TERMINALLY ENCODED PEPTIDE (CEP) family (Tabata et al., 2014). Arabidopsis has 12 canonical CEP genes, each encoding one or more conserved 15-amino acid CEP domains from which the secreted, mature CEP peptide hormones are derived (Ogilvie et al., 2014). Since the identification of CEPR1 as a CEP receptor (Tabata et al., 2014), its developmental and physiological role has been defined primarily in the context of roots. For example, CEP-CEPR1/CRA2 signaling controls the extent of root growth and development, root nodule number in legumes, and nitrate uptake in roots (Tabata et al., 2014; Mohd-Radzman et al., 2016; Roberts et al., 2016; Taleski et al., 2016, 2018; Chapman et al., 2019, 2020; Delay et al., 2019). Grafting and split-root studies show that CEPR1 influences nitrate uptake in Arabidopsis via a systemic mechanism and that the rate of nitrate uptake is reduced in cepr1-1 (Tabata et al., 2014). The identification of mature CEPs in the xylem streams of various plants also supports the existence of systemic mechanisms (Tabata et al., 2014; Okamoto et al., 2015; Patel et al., 2018). The interaction of the root-derived CEPs with CEPR1 in the shoot vasculature triggers the up-regulation of phloem-mobile shoot-to-root signals, namely the glutaredoxins CEP DOWNSTREAM1 (CEPD1) and CEPD2, that up-regulate the level of nitrate transporter expression in roots selectively exposed to high nitrate (Ohkubo et al., 2017). Recently, we demonstrated that local and systemic CEP-CEPR1 signaling curtails the expenditure of resources to control lateral root growth in response to elevated shoot-derived carbon (Chapman et al., 2019) and that systemic CEP-CEPR1 signaling controls aspects of root system architecture in soil (Chapman et al., 2020). CEP-CEPR1 signaling also controls main root growth in Arabidopsis (Delay et al., 2013b, 2019). In M. truncatula, CRA2 controls root nodulation systemically from the shoot; however, a local interaction of CEPs with CRA2 controls the growth of lateral roots (Huault et al., 2014; Mohd-Radzman et al., 2015; Laffont et al., 2019).
Whether CEP-CEPR1 signaling plays a role in the growth of shoots has not been thoroughly explored. Aboveground, the cepr1-1 mutant has been described as dwarfed, producing smaller rosettes with pale green leaves and a shorter floral stem that overaccumulates anthocyanins (Bryan et al., 2012; Tabata et al., 2014). Since these traits are typical responses to nitrogen deficiency (Vidal and Gutiérrez, 2008; Takatani et al., 2014), it could be reasonable to dismiss any cepr1 aboveground defects as simply the result of reduced nitrate acquisition by the cepr1 roots. Our anecdotal observations of two cepr1 null mutants, however, indicated that their yield was reduced much more dramatically than expected based on their modest reduction in vegetative growth. In addition, we observed that the cepr1 mutants produced smaller seeds. These phenotypes appear inconsistent with an effect of CEPR1 on nitrate uptake alone, given that wild-type plants grown at low nitrogen, or mutants with impaired nitrate uptake, produce normally sized but fewer seeds such that their yield losses are proportional to the decreases in vegetative growth (Schulze et al., 1994; Masclaux-Daubresse and Chardon, 2011).
In contrast to plants with impaired root nitrate uptake, an impairment in the remobilization of assimilated nitrogen from vegetative to reproductive tissues leads to a reduction in both seed size and yield (Guan et al., 2015; Li et al., 2015; Di Berardino et al., 2018; Moison et al., 2018). Smaller seeds also result from knocking out particular USUALLY MULTIPLE ACIDS MOVE IN AND OUT TRANSPORTERS (UMAMIT) genes, which encode transporters required for delivering assimilated nitrogen to seeds (Müller et al., 2015). It is not known if CEPR1 signaling affects nitrogen mobilization and delivery to reproductive sinks; however, the phenotypic similarities of cepr1 with mutants impaired in these processes hint at this possibility and suggest that CEPR1’s control over seed size and yield extends beyond its influence over root nitrate uptake. Supporting this hypothesis, we noted that several Arabidopsis CEPs are expressed in reproductive tissues (Roberts et al., 2013) and that rice (Oryza sativa) CEPs OsCEP5 and OsCEP6 are specifically expressed at defined stages of reproductive development (Ogilvie et al., 2014; Sui et al., 2016). CEPR1 is expressed throughout the shoot and root vasculature (Bryan et al., 2012; Huault et al., 2014; Tabata et al., 2014), suggesting roles in multiple tissues.
To explore a potential role for CEP-CEPR1 signaling in reproduction, we addressed several questions. First, what is the physiological basis for yield reduction in cepr1 mutants? Second, can yield losses in cepr1 be restored by complementation with transgenic CEPR1 or by manipulating nutrient allocation from the vegetative tissues? Third, is CEPR1 expressed in reproductive tissues, and is its effect on yield controlled systemically via vegetative tissues or locally in reproductive tissues? Finally, does CEPR1 regulate genes involved in nitrogen homeostasis/nutrient mobilization in the reproductive tissues?
In this study, we demonstrate that CEPR1 has a specific role in reproductive tissues in the promotion of fecundity, seed yield, and size. The two cepr1 knockout mutants showed yield reductions of between 88% and 98%, which were associated with the production of smaller seeds and a diminished number of reproductive units. These yield defects correlated with poorly developed reproductive tissues as well as chlorosis and the accumulation of anthocyanins in cepr1 reproductive tissues, all of which could be restored by transgenic complementation with CEPR1. Bolt grafting and manipulation of nutrient allocation to reproductive sinks showed that local CEP-CEPR1 signaling underpins the poor fecundity of the cepr1 mutants. We found that CEPR1 expression in the reproductive organs occurred specifically in the vasculature. Notably, CEPR1 expression in the seed was restricted to the chalazal seed coat, the site where nutrients for seed filling are unloaded from the terminating maternal vasculature (Müller et al., 2015). This result supported a local role for CEPR1 in the control of seed size and yield through activity in the mother tissue. Finally, we used transcriptional profiling of key marker genes to demonstrate a perturbation of nitrogen status in cepr1 bolts. Collectively, the results reveal a role for CEP-CEPR1 signaling that involves local activity in the bolt to control nitrogen mobilization and delivery to reproductive sinks.
RESULTS
CEPR1 Controls Vegetative Growth, Reproductive Development, and Seed Yield
We explored whether CEPR1 plays a role in vegetative and reproductive development by examining two independent knockout mutant alleles in the Nossen (No-0) and Columbia (Col-0) backgrounds (cepr1-1 and cepr1-3, respectively; Tabata et al., 2014; Chapman et al., 2019). Both cepr1 knockout mutants displayed an ∼30% retardation in rosette growth (Fig. 1, A–C). Since vegetative leaves mobilize resources to the bolt and other reproductive tissues, we examined whether the loss of CEPR1 function affected yield. The cepr1 mutants displayed a reduction in the growth of reproductive tissues (Fig. 1, D and E), and the number of reproductive units (siliques, flowers, and buds) on the main inflorescence was reduced by ∼10% in cepr1-1 and ∼40% in cepr1-3 (Supplemental Fig. S1). The diminished reproductive capacity correlated with a substantial reduction in the total seed yield per plant of ∼88% and ∼98% in cepr1-1 and cepr1-3, respectively (Fig. 1, F and G). The reduced seed yield resulted from a lower seed number and a 12% to 25% reduction in seed weight (Fig. 1, H and I). In general, the limited seeds produced by the cepr1 mutants were smaller and more diverse in size compared with wild-type seeds (Fig. 1, J and K). These results demonstrate that CEPR1 knockout reduces vegetative growth as well as seed size and yield.
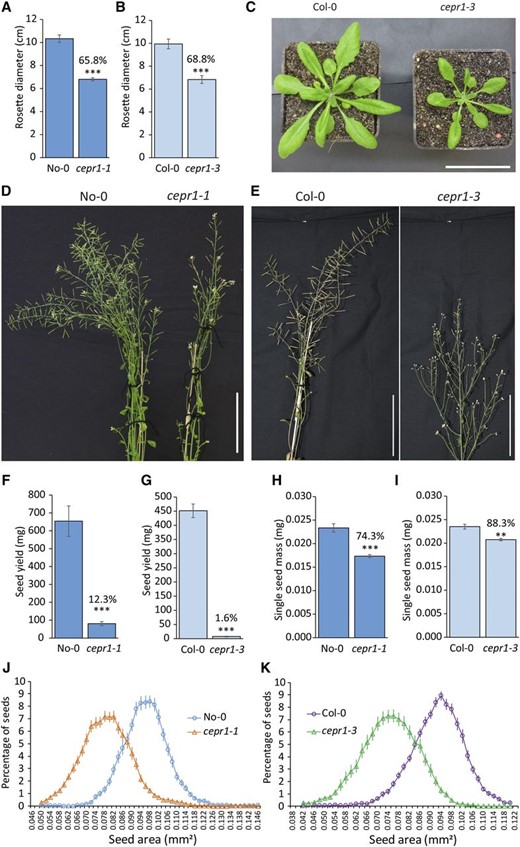
CEPR1 affects aboveground plant growth, yield, and seed size. A to C, CEPR1 mutants show decreased vegetative leaf growth. A, Rosette diameter at 30 d post germination (dpg) for No-0 and cepr1-1. n = 10. B, Rosette diameter at 31 dpg for Col-0 and cepr1-3. n = 4 to 8. C, Representative images of plants in B. Note that cepr1-3 rosette leaves displayed no obvious chlorosis. Bar = 5 cm. D to I, Loss of CEPR1 function results in impaired yield. D, Inflorescence stems of No-0 and cepr1-1 at 50 dpg. E, Inflorescence stems of Col-0 and cepr1-3 at 59 dpg. Bars in D and E = 100 mm. F and G, CEPR1 mutants show reduced yield. Total seed yield per plant is shown for No-0 and cepr1-1 (n = 6–10; F) and for Col-0 and cepr1-3 (n = 4–8; G). H and I, CEPR1 mutants have reduced seed size. Mass of one seed for No-0 and cepr1-1 (n = 3 plants; H) and for Col-0 and cepr1-3 lines (n = 4 plants; I) was determined from ∼100 seeds per plant. Percentages above bars indicate means as a percentage of the wild type. Significant differences were determined by a two-sample Student’s t test: **P < 0.01 and ***P < 0.001. Error bars indicate se. J and K, Distribution of seed size for the wild type and cepr1 in the No-0 (J) and Col-0 (K) backgrounds. n = 4 to 7 plants, 60 to 120 seeds per plant.
CEPR1 Activity Controls the Proper Development of Reproductive Organs
The severe reductions in cepr1 seed yield (∼88%–98%) seemed disproportionate to the decrease in vegetative growth (∼30%) and the reduced number of reproductive units per main inflorescence (∼10%–40%). Therefore, we examined if CEPR1 knockout mutants had additional effects on reproductive development. An examination of floral development in the cepr1 mutants (Fig. 2) revealed chlorosis in the sepals of the developing buds and those of the open flowers (Fig. 2, A–H) and anthocyanin accumulation in the apical region of the main stem, pedicle, and valves of the siliques, especially in cepr1-3 (Fig. 2, E–H). This anthocyanin accumulated in the cepr1 silique valves shortly after fertilization (stage 14; Smyth et al., 1990), intensified during silique elongation (stages 15–17), and subsided as the silique reached full length (late stage 17; Fig. 2, E–H).
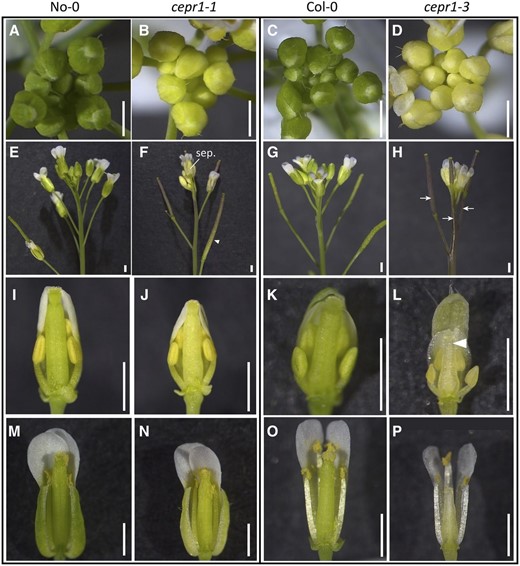
CEPR1 affects the development of reproductive organs. Representative images show floral and reproductive organ phenotypes of wild-type and cepr1 mutant plants in the No-0 and Col-0 accessions. A to D, Inflorescences of the wild type and cepr1 mutants. The developing buds of CEPR1 knockout mutants are chlorotic. E to H, Side views of the inflorescences showing chlorotic sepals (sep.) and anthocyanin accumulation in the siliques, pedicle, and main stem of cepr1 (arrows). The arrowhead in F indicates the fading of anthocyanin in older siliques. I to P, Medial views of dissected wild-type and cepr1 flowers showing the aberrant floral development associated with CEPR1 knockout. I to L, Preanthesis stage 12 wild-type and cepr1 flowers. The arrowhead in L indicates the poorly developed distal end of a cepr1-3 gynoecium. M and N, Postanthesis stage 13 flowers of wild-type No-0 and cepr1-1. O and P, Postanthesis stage 14 flowers of wild-type Col-0 and cepr1-3. Bars = 1 mm.
We examined the preanthesis floral structure in dissected stage 12 flowers of wild-type No-0 and cepr1-1 (Fig. 2, I and J). The cepr1-1 flowers were smaller than wild-type No-0 but retained the relative dimensional ratios between the different floral organs. There was a clear differentiation of the substructures of the cepr1-1 gynoecium (i.e. stigma, style, valves, and replum), but, like the sepals, these tissues were chlorotic compared with wild-type No-0 (Fig. 2J). Postanthesis cepr1-1 flowers (stage 13) were smaller and chlorotic compared with wild-type No-0, but the anthers still elongated and deposited pollen on the stigma similarly to wild-type No-0 (Fig. 2, M and N).
Floral development in the Col-0 cepr1-3 mutant was impaired more severely than in the No-0 mutant, and it was not possible to determine floral stage by the conventional landmark developmental events (Smyth et al., 1990). Instead, we determined floral buds equivalent to wild-type Col-0 stage 12, based on the relative position of the bud within the inflorescence from the most recent anthesed flowers. Although the cepr1-3 sepals were of similar size to those of Col-0, there was a severe underdevelopment of the organs in the inner whorls (Fig. 2, K and L). The cepr1-3 gynoecium was typically pear shaped and stunted, with a translucent appearance (Fig. 2L). In cepr1-3, the petals expanded and the anthers elongated and dehisced; however, the stunting of the gynoecium resulted in a state of near hercogamy (i.e. reduced self-pollination; Fig. 2, O and P). We observed only occasional deposition of cepr1-3 pollen from the shorter medial stamens onto the poorly developed stigma. Collectively, these results show that loss of CEPR1 activity perturbs floral and reproductive organ development, with the impact being more severe in Col-0 cepr1-3 compared with No-0 cepr1-1. This suggests that background genetic differences between the Col-0 and No-0 accessions modify the severity of the reproductive development defects resulting from a CEPR1 knockout.
CEPR1 Positively Influences Seed Yield on a per Silique Basis
Since the loss of CEPR1 function affected flower development, we investigated whether there were effects on yield per silique in cepr1 (Fig. 3). First, we assessed siliques of self-pollinated plants and observed that cepr1 mutants had a higher incidence of unfertilized ovules and of seeds that had aborted at various stages of development (Fig. 3, A and B). The reduction in seed set per silique was particularly severe in cepr1-3 and worsened acropetally. Therefore, we quantified seed set across silique positions (Fig. 3, C and D). In wild-type No-0, seed set steadily improved with increasing silique positions up the stem, reaching a maximum at approximately silique 14 (Fig. 3C). The cepr1-1 mutant also showed an improving seed set with increasing silique position up the main shoot, albeit at a decreased rate compared with wild-type No-0. Maximum seed set in cepr1-1 was lower compared with wild-type No-0 (Fig. 3C). Seed set in wild-type Col-0 was minimal in the first silique and increased to a maximum at approximately silique 6 (Fig. 3D). In contrast to wild-type Col-0, cepr1-3 had maximal seed set in the first silique, which decreased with increasing silique position (Fig. 3D). We observed nil seed set from silique number 12 onward in cepr1-3. For siliques with nonzero fecundity, the average seed set was significantly lower in cepr1-1 and cepr1-3 compared with their respective wild-type lines (Fig. 3, E and F). Moreover, the frequency of clearly fertilized and then aborted seeds (i.e. late-aborting seed) was higher in both cepr1 mutants compared with their respective wild-type lines, and this phenotype was particularly severe in cepr1-3 (Fig. 3, G and H).
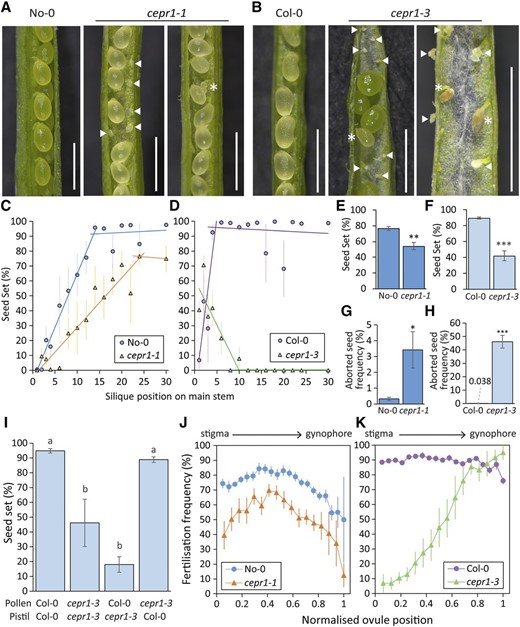
Loss of CEPR1 function results in decreased seed set and a higher frequency of seed abortion. A and B, Representative images of dissected siliques from self-pollinated wild-type and cepr1 lines in the No-0 (A) and Col-0 (B) backgrounds. Arrowheads indicate unfertilized ovules. Asterisks indicate aborted seeds. Bars = 1 mm. C and D, Seed set at silique positions on the main stem (position 1 = first silique produced) for self-pollinated wild-type and cepr1 lines in the No-0 (n = 4–6; C) and Col-0 (n = 3–6; D) backgrounds. Error bars show se. For each plant line, two separate linear trend lines were applied to the distinct subset of silique positions before and after a plateau in seed set. E and F, Average seed set determined from siliques with nonzero seed set irrespective of silique position for self-pollinated wild-type and cepr1 plants in the No-0 background (n = 6 plants, seven to 14 siliques per plant; E) and the Col-0 background (n = 5–6 plants, two to 15 siliques per plant; F). G and H, Frequency of aborted seeds observed at fertilized ovule positions for self-pollinated wild-type and cepr1 plants in the No-0 background (n = 6 plants, seven to 14 siliques per plant; G) and the Col-0 background (n = 5–6 plants, two to 15 siliques per plant; H). Significant differences in E to H were determined by a two-sample Student’s t test: *P < 0.05; **P < 0.01; and ***P < 0.001. Error bars show se. I, Percentage seed set for controlled pollination between Col-0 and cepr1-3 plants. Preanthesis flowers were emasculated prior to deposition of donor pollen onto recipient pistils. Significant differences were determined by ANOVA followed by Tukey’s honestly significant difference (HSD) test (α = 0.05). n = 4 to 6 siliques from three plants. Error bars show se. J and K, Fertilization frequency at ovule positions for self-pollinated wild-type and cepr1 plants in the No-0 background (n = 6 plants, seven to 14 siliques per plant; J) and the Col-0 background (n = 5–6 plants, two to 15 siliques per plant; K). Each position is represented as a fraction of the highest position observed in at least three plants per genotype (0 = stigma end; 1 = gynophore end). Fertilization frequency was determined from siliques with nonzero seed set.
We conducted reciprocal crosses to determine whether the more severe seed set reduction in cepr1-3 was due to a male and/or female reproductive defect (Fig. 3I). Pollen from cepr1-3 fertilized wild-type pistils without impairment, which suggested that there was no appreciable decrease in cepr1-3 pollen viability. The reduced fecundity of the cepr1-3 pistil compared with the wild type held true regardless of pollen genotype. As mechanical pollination would overcome pollen deposition defects resulting from asynchronous anther-gynoecium development in cepr1-3 (Fig. 2, O and P), we reasoned that a female reproductive defect limits cepr1-3 seed set.
To further explore this female fertility defect, we examined fertilization frequency depending on ovule position in the pistil. Such analysis helps distinguish between problems with pollen transmission and ovule-specific defects (Kay et al., 2013; Groszmann et al., 2020). As cepr1 mutants in both backgrounds had fewer ovules per pistil than the wild type (Supplemental Fig. S2), we assessed seed set at normalized ovule positions within the carpels, from position 0 (closest to the stigma) to position 1 (closest to the gynophore; Fig. 3, J and K). Compared with wild-type No-0, cepr1-1 showed a similar, albeit more accentuated, position-dependent fertilization pattern in the pistil (Fig. 3J). As expected, ovules of wild-type Col-0 pistils showed a high and mostly position-neutral fertilization frequency (Fig. 3K). In stark contrast, the fertilization rate in cepr1-3 was very low at the stigma end and gradually increased toward the proximal region of the pistil, eventually reaching wild-type Col-0 levels (Fig. 3K). The high fertilization rate of ovules farthest from the stigma in cepr1-3 demonstrates that pollen tubes can traverse the entire distance of the transmitting tract tissue. This indicates that the defect responsible for the reduced ovule fertility is not in the female pollen-transmitting tissues (e.g. stigma and transmitting tract) but rather is specific to the ovules. This fertilization pattern of cepr1-3 correlated spatially with the poor development of the distal region of the gynoecium (Fig. 2, L and P).
CEPR1 Activity Controls Seed Filling
One component of the yield decrease in cepr1 is a reduction in mature seeds per silique due to a reduced ovule number, a lower fertility rate, and an increased incidence of seed abortion. In addition, cepr1 seeds that successfully progress to maturity are smaller than wild-type seeds, further contributing to the lower yield. Normally, plants with a reduced seed number tend to compensate by having larger seeds, which is due to a greater proportional allocation of the available nutrients being remobilized from the rosette (Bennett et al., 2012). Therefore, the small mature seeds of cepr1 may reflect a deficiency in nutrient supply to the seeds. This may arise due to its smaller source rosette and, hence, a proportionately lower per seed availability of nutrients, or alternatively, due to a reduced capacity of cepr1 plants to deliver nutrients from the source rosette to the seed. To test these possibilities, we manipulated nutrient allocation from the rosette (source) by thinning the number of competing reproductive organs (sinks) to improve the source-to-sink ratio in favor of larger seeds in the siliques that remained (Bennett et al., 2012). As expected, we found that seed size increased in response to the thinning of wild-type plants; however, an increase in seed size did not occur in cepr1-1 despite improving the source-to-sink ratio (Fig. 4). This result suggests that CEPR1 is required for the redistribution and/or delivery of resources for seed filling and, in doing so, controls seed size.
![CEPR1 determines the extent of seed filling. The resource availability per silique was increased by thinning the number of reproductive sinks. A, Images of No-0 (wild type [WT]) and cepr1-1 before (left) and after (right) thinning. Three to four open flowers or early-stage siliques were left at the apex of the main stem. B, Relative seed area for thinned and untreated plants. Significant differences were determined by ANOVA followed by Tukey’s HSD test (α = 0.05). n = 3 plants, 100 to 200 seeds per plant. Error bars show se.](https://oup.silverchair-cdn.com/oup/backfile/Content_public/Journal/plphys/183/2/10.1104_pp.20.00172/3/m_plphys_v183_2_620_f4.jpeg?Expires=1750222680&Signature=kK3ddIL9W7hLVF95U53DnQET12kKUPz40Lv8o2m7X3L6JBk7gP-OcyNKA4DFF3Kn9yY3PN6w9wyurn1KjIIOG4QBsIRuMYmiWD2lORi49satdAWIfP37dYpat-5zusGqhud146CgDinpvlX3xi4KAoafjinrqw25LP2ApTJJGnW8iiiWV1AbyvmjgdGtPrvMTp6GtnGv-9pdgFOhOAOzDT73Kuksbb0yehQAjmOhOZemO3jpC18G9tPtRaCGYFJsEEMqGYaCFnjyyBUD0SQAkqoaim9~48giln7J3a-4~qtBrCK-Uzg2q8WhcIjtsiQWOITVHLHwIdqLu3MtMLOmaw__&Key-Pair-Id=APKAIE5G5CRDK6RD3PGA)
CEPR1 determines the extent of seed filling. The resource availability per silique was increased by thinning the number of reproductive sinks. A, Images of No-0 (wild type [WT]) and cepr1-1 before (left) and after (right) thinning. Three to four open flowers or early-stage siliques were left at the apex of the main stem. B, Relative seed area for thinned and untreated plants. Significant differences were determined by ANOVA followed by Tukey’s HSD test (α = 0.05). n = 3 plants, 100 to 200 seeds per plant. Error bars show se.
CEPR1 Control of Seed Size Depends on CEPR1 Activity in the Reproductive Bolt
We undertook reciprocal bolt grafting between the wild type and cepr1-1 to elucidate if CEPR1 activity in the vegetative tissues or reproductive tissues determined seed size (Fig. 5, A and B; Supplemental Fig. S3). Early observations of established grafted bolts revealed that both the chlorosis and the accumulation of anthocyanins in the siliques and inflorescence stem persisted in cepr1 bolts even when grafted onto wild-type stock (Fig. 5C). This result suggested that juvenile cepr1 bolts derived little or no additional nutritional benefit from the wild-type stock. At maturity, we harvested the seeds and other dry bolt materials (i.e. stem, cauline leaves, and floral and silique material) to assess the effect of graft combination on seed size and yield. We found that the smaller size of seeds produced by cepr1 bolts could not be rescued by grafting to a wild-type stock (Fig. 5D). In contrast, there was no penalty to the seed size of wild-type bolts when grafted to cepr1-1 stock (Fig. 5D). Furthermore, the distribution of seed size for wild-type bolts was more uniform than for cepr1-1 bolts regardless of the stock (Fig. 5E). This suggests that local CEPR1 activity in the bolt controls seed size and its uniformity. A weak, compensatory effect of vegetative CEPR1 activity was observed for cepr1-1 bolts grafted onto a wild-type stock (i.e. cepr1-1/wild type), with mild improvements in seed size uniformity compared with cepr1-1 homografts (Fig. 5E).
![Seed size depends upon CEPR1 activity in the bolt. Young No-0 (wild-type [WT]) and cepr1-1 bolts were excised approximately 2 cm from the base and grafted to a recipient stock, which provided vegetative rosette and root tissues to the transplanted reproductive tissue. Secondary branches that formed from the stock after grafting were continuously removed so that the final reproductive organs were derived from the donor bolt only. The bolt and stock genotypes are labeled above and below the horizontal line, respectively. A and B, Reciprocal grafting of wild-type and cepr1-1 plants. Representative images show plants 0 (A) and 26 (B) d after grafting (bolt/stock genotype). Arrowheads in A indicate the graft junction. Bars = 5 cm. C, Representative images of inflorescences from heterografted plants. Note the accumulation of anthocyanin in the siliques of cepr1-1 bolt grafts. D, Quantification of single seed mass for grafted plants. Statistically significant differences were determined by ANOVA followed by Tukey’s HSD test (α = 0.05). n = 6 to 9. E, Distribution of seed size. n = 6 to 9 plants, 100 to 200 seeds per plant. Error bars show se.](https://oup.silverchair-cdn.com/oup/backfile/Content_public/Journal/plphys/183/2/10.1104_pp.20.00172/3/m_plphys_v183_2_620_f5.jpeg?Expires=1750222680&Signature=PYJpKhnZOszfQSgTopI4JAdQlDJZv2GUL0AarRDLtB~dTjjdI6zTzQioux4g6oDwXLbe9K6YmI3OhVK7HZRVyCTPqTlKVp1uwPLHc6jHBAz6I1YXh2BvDWFFFx9vpsXMCal1rYLeD9cgZ1lpunATq2VXMfeA1glCtS8624VK1aTV3MP5l2cBPznNH9FKujp0Zv9Q~srMNZKCxj9lFVu49elM8oaihX41d~opEX3qTGa9M2qEtm3U9B~2Y1nVOX82zKNotJ9ej7cc3U8rvN4Ttw3gCwdxOD47Ks0tRboakpzDcTVK4rowrztJOi4I86psjxXwCVF~9QsJTeb0DJvxrg__&Key-Pair-Id=APKAIE5G5CRDK6RD3PGA)
Seed size depends upon CEPR1 activity in the bolt. Young No-0 (wild-type [WT]) and cepr1-1 bolts were excised approximately 2 cm from the base and grafted to a recipient stock, which provided vegetative rosette and root tissues to the transplanted reproductive tissue. Secondary branches that formed from the stock after grafting were continuously removed so that the final reproductive organs were derived from the donor bolt only. The bolt and stock genotypes are labeled above and below the horizontal line, respectively. A and B, Reciprocal grafting of wild-type and cepr1-1 plants. Representative images show plants 0 (A) and 26 (B) d after grafting (bolt/stock genotype). Arrowheads in A indicate the graft junction. Bars = 5 cm. C, Representative images of inflorescences from heterografted plants. Note the accumulation of anthocyanin in the siliques of cepr1-1 bolt grafts. D, Quantification of single seed mass for grafted plants. Statistically significant differences were determined by ANOVA followed by Tukey’s HSD test (α = 0.05). n = 6 to 9. E, Distribution of seed size. n = 6 to 9 plants, 100 to 200 seeds per plant. Error bars show se.
CEPR1 Activity Controls Total Seed Yield Primarily in the Reproductive Bolt
Since the decrease in total seed yield of the cepr1-1 bolt could not be rescued by grafting to a wild-type stock (Fig. 6A), a lack of CEPR1 activity in the bolt most likely limits yield in cepr1-1 plants. Moreover, there was no yield penalty to a wild-type bolt when grafted to a cepr1 stock but, rather, an apparent increase in total seed yield relative to the wild-type homografts (Fig. 6A). An examination of the dry mass of harvested bolt material (excluding seed) revealed that wild type-on-cepr1-1 grafts had significantly greater bolt dry mass than the other graft combinations (Fig. 6B), consistent with the greater bolt growth observed for this graft combination at 26 d (Fig. 5B). To account for differences in bolt growth, we calculated the ratio of seed yield to total bolt biomass (bolt harvest index; Fig. 6C). The bolt harvest index of wild type-on-cepr1-1 grafts was not different from that of wild-type homografts. Compared with the cepr1-1 homografts, the bolt harvest index of cepr1-1 slightly improved when grafted to a wild-type stock (increasing from ∼37% to ∼50% of wild-type homografts). Therefore, while a wild-type stock could partially compensate for a lack of CEPR1 in the bolt, the bolt harvest index was primarily determined by CEPR1 activity in the bolt. These results, together with the seed size data, show that CEPR1 bolt activity controls reproductive development, seed size, and subsequently total yield.
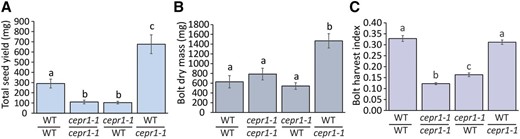
Yield is primarily determined by CEPR1 activity in the reproductive bolt. Analysis of yield is shown on a per plant basis for reciprocal wild-type (WT) and cepr1-1 bolt-grafted plants (bolt/stock genotype). Quantification of total seed yield (A), bolt dry mass (minus seed; B), and bolt harvest index (the ratio of seed yield to total bolt biomass; C) are shown. Significant differences were determined by ANOVA followed by Tukey’s HSD test (α = 0.05). Error bars show se. n = 6 to 9 plants.
CEPR1 Is Expressed in the Vasculature of Floral and Reproductive Organs
To help elucidate how CEPR1 influences reproductive development and fecundity, we examined CEPR1 expression in reproductive organs using a 2-kb CEPR1 promoter-GUS reporter (pCEPR1:GUS). pCEPR1:GUS was expressed in the vasculature of the inflorescence stem, the base of floral buds, as well as floral and reproductive organs (Fig. 7, A and B). In flowers, expression was detected in the vasculature of well-developed sepals and petals, elongated stamen filaments (Fig. 7, A, C, and D), developing gynoecium prior to fertilization (Fig. 7E), mature gynoecium (Fig. 7F), and the growing silique (Fig. 7G). CEPR1 expression in the maternal reproductive tissues appeared throughout the entire vasculature network, including the medial and lateral vascular strands, the terminating vascular bundles in the style, and the vasculature of the funiculus right to the point of termination within the chalazal seed coat (Fig. 7, F–H). We observed CEPR1 expression within the funiculus and chalazal seed coat only after fertilization (Fig. 7, E and F), and it persisted throughout seed development (Fig. 7, H and I). We detected no expression of CEPR1 in the endosperm or embryo.
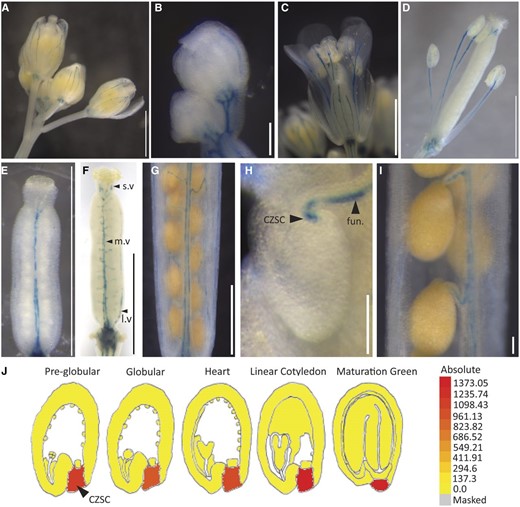
CEPR1 expresses in the vasculature of reproductive organs. A to I, pCEPR1:GUS expression in the reproductive organs. Shown are whole inflorescence (A), buds (B), stage 14 flower (C), stage 15 gynoecium and stamens (D), stage 12 gynoecium (E), stage 14 gynoecium (F; l.v, lateral vasculature; m.v, medial vasculature; s.v, style vasculature), mature silique (G), funiculus (fun.) and chalazal seed coat (CZSC; H), and mature silique funiculus (I). Staining was carried out for 18 h (A, D, G, and I), 24 h (H), or 72 h (B, C, E, and F). Bars = 1 mm (A and C–G) and 0.1 mm (B, H, and I). J, Microarray expression of CEPR1 in the developing seed from the Arabidopsis eFP browser (https://bar.utoronto.ca/efp/cgi-bin/efpWeb.cgi; Belmonte et al., 2013).
The restriction of seed pCEPR1:GUS expression to the chalazal seed coat is consistent with publicly available expression data (Fig. 7J; Belmonte et al., 2013; Arabidopsis eFP browser [https://bar.utoronto.ca/efp/cgi-bin/efpWeb.cgi]). CEPR1 expression in the chalazal seed coat is important since it represents the final delivery point of nutrients via the vasculature from the mother tissue prior to their uptake via a variety of transporters to the symplasmically isolated filial tissues (Stadler et al., 2005; Chen et al., 2015; Müller et al., 2015; Hoai et al., 2020). The lack of pCEPR1:GUS or CEPR1 mRNA expression in the endosperm or embryo tissue indicates that CEPR1 control of seed size occurs strictly through activity in the mother tissue. Consistent with this, restoring wild-type CEPR1 in only the filial tissue of the cepr1-3 mutant via crossing (i.e. cepr1-3 ♀ × wild-type Col-0 ♂) did not improve seed size (Supplemental Fig. S4).
Transgenic CEPR1 Rescues cepr1 Reproductive and Yield Defects
We complemented both cepr1 mutants using a transgene containing CEPR1 driven by the same 2-kb upstream sequence used in our GUS reporter expression analysis (Fig. 8). The examination of multiple complemented lines revealed that the transgene rescued several obvious cepr1 developmental defects (e.g. anthocyanin accumulation in stem and silique, smaller chlorotic flowers, and aberrant floral organ morphology; Fig. 8, A and B). Further analysis of two independent complemented lines of each cepr1 allele demonstrated full or substantial rescue of ovule number (Fig. 8, C and G), seed set (Fig. 8, D and H), seed abortion (Fig. 8, E and I), and seed size (Fig. 8, F and J). These results indicate that the 2 kb of sequence upstream of CEPR1 is sufficient for native CEPR1 function and imply that a loss of CEPR1 expression in the vasculature is the causal factor leading to the developmental, reproductive, and yield defects associated with the cepr1 mutants.
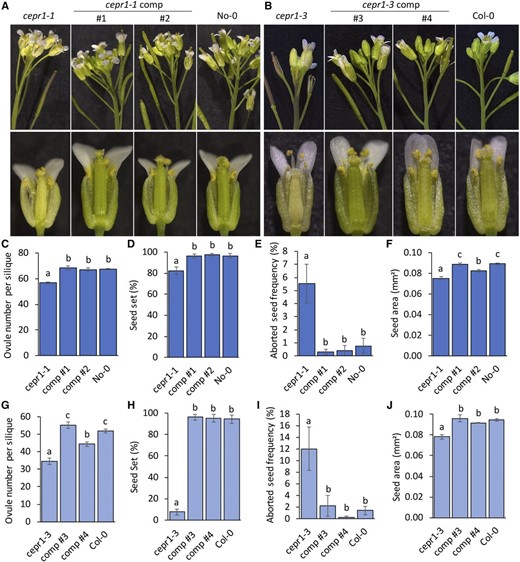
Complementation of cepr1 mutants using a CEPR1 genomic fragment. Reproductive development phenotypes were assessed for independent complementation (comp) lines in the No-0 cepr1-1 (1 and 2) and Col-0 cepr1-3 (3 and 4) backgrounds compared with their respective cepr1 mutant and wild-type lines. A and B, Representative images showing side views of the inflorescence (top row) and dissected postanthesis flowers (bottom row) for lines in the No-0 (A) and Col-0 (B) ecotypes. C and G, Quantification of ovule number for siliques with nonzero fecundity for No-0 (n = 4–10, two siliques per plant; C) and Col-0 (n = 3–11, one to four siliques per plant; G) ecotype lines. D and H, Measurement of seed set for No-0 (n = 4–10; D) and Col-0 (n = 3–11; H) ecotype lines. Two siliques were examined per plant from positions on the stem corresponding to the phase of wild-type maximal seed set (Fig. 3, C and D). E and I, Frequency of aborted seeds observed at fertilized ovule positions for lines in the No-0 (n = 4–10, two siliques per plant; E), and Col-0 (n = 3–11, one to four siliques per plant; I) ecotypes. F and J, Seed area for No-0 (n = 6–11 plants; F) and Col-0 (n = 3–9 plants; J) ecotype lines determined from 60 to 120 seeds per plant. Significant differences were determined by ANOVA followed by Fisher’s lsd test (α = 0.05). Error bars show se.
CEPR1 Regulates the Expression of Genes Involved in Nitrogen Homeostasis in the Reproductive Bolt
Anthocyanin accumulation and chlorosis are signs of imbalances in nitrogen and carbon, especially where nitrogen is low and carbon is in proportional excess (Takatani et al., 2014). Therefore, nutritional limitation may account for the cepr1 defects in floral morphology, lower fecundity, high incidence of seed abortion, and smaller, more variable seed sizes. To investigate this, we harvested bolt and inflorescence tissues and surveyed the expression of several genes involved in the CEP-CEPR1 signaling pathway along with several genes involved in nitrogen and carbon homeostasis (Fig. 9). CEPD1, which is positively regulated by CEP-CEPR1 signaling in shoots (Ohkubo et al., 2017), was down-regulated approximately 8-fold in the cepr1 bolt tissue (Fig. 9A). Three out of four CEP ligand-encoding genes known to be expressed in the bolt (Roberts et al., 2013; Col-0 accession) were also differentially expressed in the cepr1 mutants. CEP5 and CEP9 were strongly up-regulated (∼32-fold), CEP2 was down-regulated in cepr1-3 (approximately 3-fold) and undetectable in the No-0 background, and CEP1 expression was not significantly altered (Fig. 9A). The cepr1 mutants had an ∼60% reduction in the expression of GLUTAMINE SYNTHETASE1;2 (GLN1;2; Fig. 9B), which encodes the main isozyme contributing to Gln synthetase activity in the shoot (Guan et al., 2016) and is known to be involved in nitrogen mobilization and yield formation (Diaz et al., 2008; Guan et al., 2016; Moison et al., 2018). The expression of the nitrate reductase gene NITRATE REDUCTASE1 (NIA1), also involved in nitrogen metabolism (Wilkinson and Crawford, 1993), was not significantly different. The expression of UMAMIT14, an amino acid transporter gene linked to seed filling (Müller et al., 2015), was strongly down-regulated in the cepr1 mutants (greater than 80% reduction; Fig. 9B). In contrast, UMAMIT11 and UMAMIT18/SILIQUES ARE RED1 (SIAR1), which also supply assimilated nitrogen as amino acids to reproductive tissues (Ladwig et al., 2012; Müller et al., 2015), were not differentially expressed in the cepr1 mutants (Fig. 9B). Consistent with the observed anthocyanin accumulation in cepr1 stems, there was a substantial up-regulation in the cepr1 mutants of one or both of the low-nitrogen-induced MYB transcription factors PRODUCTION OF ANTHOCYANIN PIGMENT1 (PAP1) and PAP2, which are involved in anthocyanin production when nitrogen is limited (Scheible et al., 2004; Lea et al., 2007; Rubin et al., 2009; Fig. 9B).
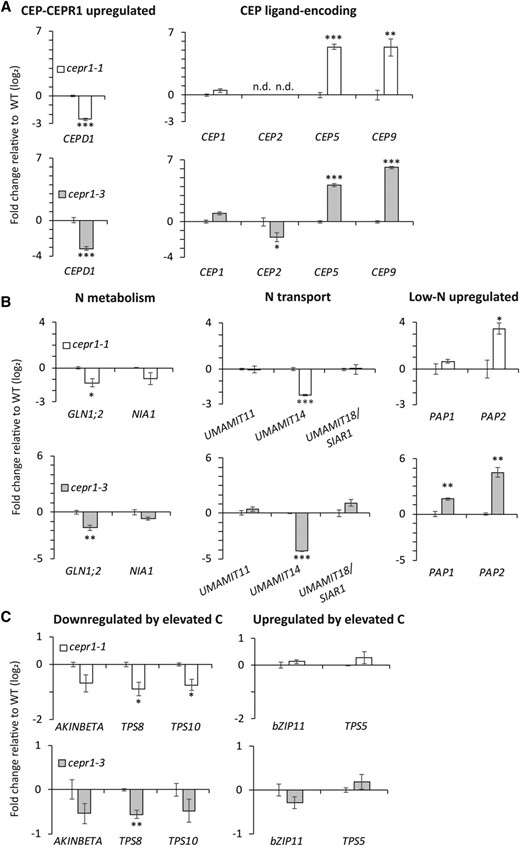
Loss of CEPR1 function affects the expression of genes involved in nitrogen homeostasis in bolt tissue. For reverse transcription quantitative PCR (RT-qPCR) analyses, the primary inflorescence tissue was harvested from wild-type and cepr1 plants upon first flower opening. The fold change in expression (log2) was determined for cepr1-1 and cepr1-3 relative to the wild-type (WT; No-0 and Col-0, respectively) for a selection of CEP-CEPR1 pathway genes (A), genes related to nitrogen (N) homeostasis (B), and genes related to carbon (C) homeostasis (C). Significant differences were determined by a two-sample Student’s t test: *P < 0.05; **P < 0.01; and ***P < 0.001. Error bars show se. n = 3 biological replicates each consisting of two pooled plants. n.d., Not detected.
Unlike some of the nitrogen-associated genes, marker genes responding to elevated carbon (Nunes et al., 2013; Cookson et al., 2016) were not substantially altered in the cepr1 mutants. Specifically, AKINBETA1 and the putative trehalose-6-phosphate synthase genes, TREHALOSE PHOSPHATE SYNTHASE8 (TPS8) and TPS10, which are normally down-regulated in response to elevated carbon levels, were either mildly down-regulated or unaltered in the cepr1 mutants (Fig. 9C). The Arabidopsis BASIC LEUCINE-ZIPPER11 (bZIP11) and TPS5 genes, which positively respond to elevated carbon levels, remained unchanged (Fig. 9C). Together with the nitrogen-associated transcripts, the expression profiles of cepr1 bolts imply a state of nitrogen limitation with minimal or no perturbations to carbon status.
DISCUSSION
In this study, we demonstrated a function for CEPR1 in controlling seed yield via a local circuit in the reproductive tissues of Arabidopsis. The severely decreased yield of cepr1 mutants is due to a loss of vasculature-expressed CEPR1, which compromises bolt growth, female reproductive development, seed set, and mature seed size and, in addition, causes chlorosis and anthocyanin accumulation in inflorescence tissues. The complementation of cepr1 mutants using a CEPR1 genomic fragment rescues these diverse phenotypes. Underpinning these phenotypes of the cepr1 mutants is the apparent common theme of an inability to mobilize nutrients, specifically nitrogen, either from the vegetative tissues to the young bolts or from within bolt tissues to the developing floral organs and seeds. Several lines of evidence support this view.
First, the cepr1 reproductive tissues showed chlorosis in the bolt tissues and floral organs and anthocyanin accumulation in the inflorescence stems and siliques. The grafting of young cepr1 bolts onto wild-type vegetative stocks did not remedy these well-documented phenotypes of nutrient limitation stress, suggesting a compromise of nutrient delivery from the vegetative tissue to the young cepr1 bolt. Concordantly, young wild-type bolts did not develop chlorosis or accumulate anthocyanins when grafted onto cepr1 vegetative stocks.
The grafting of cepr1 bolts onto wild-type vegetative stocks did not restore yield or a normal seed size distribution, whereas wild-type bolts had no reduction in yield or seed size when grafted to cepr1 vegetative stocks. In agreement with a role for CEPR1 in seed filling through the control of nitrogen mobilization and delivery, we found that both GLN1;2 and UMAMIT14 were down-regulated in the cepr1 reproductive bolt. The cytosolic Gln synthetase encoded by the vasculature-expressed GLN1;2 contributes to nitrogen remobilization from source tissues for seed filling (Moison et al., 2018), with gln1;2 loss-of-function mutants displaying reduced seed size and yield similar to cepr1 (Guan et al., 2015). The importance of cytosolic Gln synthetase in seed filling appears conserved across diverse species, including maize (Zea mays; Martin et al., 2006). The UMAMIT14 amino acid transporter is also expressed throughout the plant vasculature, including the chalazal seed coat, where it is required for the unloading of amino acids transported from source tissues to support seed filling. Consistent with the decreased UMAMIT14 expression in the cepr1 mutants, the umamit14 null mutants produce smaller seeds (Müller et al., 2015).
Improving nutrient allocation by thinning the number of reproductive units resulted in the expected increased seed size in wild-type plants but not in cepr1 mutants. The differential effect of thinning between the wild type and cepr1 is not due to the smaller cepr1 rosettes, because grafting showed that the cepr1 rosettes support the flourishing of wild-type bolts. Moreover, this grafting result implies that the cepr1 source rosettes are still able to sufficiently load nutrients such as amino acids into the phloem for export to the developing bolts (Santiago and Tegeder, 2016). Therefore, the seeds of cepr1 plants that were thinned appear unable to receive the expected allocation of surplus nutrient that clearly benefits the seeds of thinned wild-type plants.
CEPR1 expression was detected throughout the vasculature of reproductive tissues, consistent with a function in nutrient mobilization/delivery. The specific expression of CEPR1 in the chalazal seed coat, but not in any other seed tissues, is pertinent to the cepr1 seed size defect, since this tissue is the final delivery point of nutrients from the mother tissue via the vasculature prior to their uptake via transporters to the symplasmically isolated filial tissues (Müller et al., 2015). This localization points to CEPR1 playing an important role in nutrient unloading at the seed and indicates that it controls seed development and size strictly through activity in the maternal tissue. An impairment of nutrient delivery to the seed would be consistent with the more variable seed size and higher rates of seed abortion observed in the cepr1 mutants, with seed abortion occurring in instances where early nutrient demands of the embryo are not met (Di Berardino et al., 2018) and with the more variable mature seed sizes reflecting inconsistency in nutrient delivery.
Our RT-qPCR data indicate that the nutrient stress caused by a loss of CEPR1 function is related to impaired nitrogen status of the reproductive bolt. Consistent with a low nitrogen state and anthocyanin accumulation, cepr1 bolts displayed a strong up-regulation of one or both of the MYB transcription factors PAP1 and PAP2. These PAP genes are highly up-regulated in response to nitrogen starvation and positively regulate anthocyanin biosynthesis (Lea et al., 2007; Rubin et al., 2009). The down-regulation of the Gln synthetase gene GLN1;2 and UMAMIT14 would be consistent with decreased levels of assimilated nitrogen compounds (Patterson et al., 2010; Besnard et al., 2016). By contrast, the mild down-regulation of carbon-repressed TPS genes and the unchanged expression of carbon-induced transcripts in cepr1 bolts imply a sufficient carbon supply. A decreased nitrogen state but steady carbon state in cepr1 mutants is further supported by their accumulation of anthocyanins, which in general is not just a symptom of nitrogen limitation but rather a symptom of excess levels of carbon relative to nitrogen (Takatani et al., 2014). Moreover, several CEP-CEPR1 pathway genes both upstream (i.e. several CEP genes) and downstream (i.e. CEPD1) of CEPR1 displayed altered expression in cepr1 bolts, consistent with a locally perturbed CEPR1 signaling status involving both feedforward and feedback loops. Similar transcriptional perturbations occur to CEP-CEPR1 pathway genes locally in cepr1 root tissues (Chapman et al., 2019). These transcriptional responses, together with CEPR1 expression in the vasculature of reproductive tissues and the dependence of seed size and yield on local CEPR1 activity, demonstrate that CEPR1 specifically acts in bolt tissues to control aspects of nitrogen mobilization and delivery to reproductive sinks.
Finally, an impairment in the nitrogen economy of the bolt can also explain the cepr1 fecundity and silique defects. Nutrient deficiency is consistent with the diminished growth of the bolt and the reduced number of reproductive units produced (i.e. flowers and siliques; Perchlik and Tegeder, 2018). The aberrant and asynchronous development of the cepr1 gynoecium and stamens, contributing to the reduced seed number on a per silique basis, is comparable with the miscoordination of reproductive development occurring in rice florets when nitrogen is limited by loss-of-function mutations in arginase (OsARG) or ornithine δ-aminotransferase (OsOAT; Ma et al., 2013; Liu et al., 2018). Similar to cepr1, these rice mutants display reduced seed set and smaller seed/grain. The accumulation of anthocyanins observed in cepr1 developing siliques is identical to the phenotype of mutants in the amino acid transporter gene UMAMIT18/SIAR1 and is likely a stress symptom of inadequate nitrogen provision during the nutrient-demanding stages of seed development (Ladwig et al., 2012). The miscoordination of reproductive development along with the altered expression of some nitrogen-related genes in the cepr1 bolt are consistent with CEPR1 acting as one node of a complex network regulating nitrogen homeostasis (Castaings et al., 2011; Tegeder and Masclaux-Daubresse, 2018).
CONCLUSION
In this article, we show that CEPR1 activity in the reproductive tissue is critical for both reproductive development and yield. Our data highlight the importance of CEPR1 activity in the reproductive bolt for the delivery of nutrients for yield formation. The apparent perturbation of nitrogen status in cepr1 bolts suggests that CEPR1 plays a broader role in controlling nitrogen homeostasis at the whole-plant level beyond roles previously identified in root nitrogen acquisition, root system architecture, and nodulation (Huault et al., 2014; Tabata et al., 2014; Mohd-Radzman et al., 2016; Ohkubo et al., 2017; Chapman et al., 2020). The manipulation of CEPR1-dependent outputs could provide a new avenue to improve nutrient delivery from source tissues to reproductive sinks, with the aim of improving traits such as seed yield and nitrogen use efficiency in plants.
MATERIALS AND METHODS
Plant Materials and Growth Conditions
The previously described Arabidopsis (Arabidopsis thaliana) No-0 cepr1-1 (RATM11-2459 [RIKEN]; Bryan et al., 2012; Tabata et al., 2014) and Col-0 cepr1-3 (467C01 [GABI-Kat]; Kleinboelting et al., 2012; Chapman et al., 2019) mutant lines were used. Sterilized seedlings were grown on solidified medium (1% [w/v] type M agar) containing one-half-strength Murashige and Skoog basal salts (Sigma) at pH 5.7 for 7 to 10 d until seedlings were transferred to soil (seed-raising mix; Debco) supplemented with Osmocote Exact fertilizer and grown in chambers at 22°C. For the grafting experiment, plants were grown with 200 µmol m−2 s−1 light and an 8-h photoperiod. For all other experiments, plants were grown with 100 µmol m−2 s−1 light and a 16-h photoperiod.
Determination of Seed Size, Seed Set, and Yield Parameters
Inflorescences were covered with microperforated plastic bags to collect seeds. To determine single seed mass, aliquots were weighed and seed number per aliquot was counted. Seed area was determined using ImageJ (https://imagej.nih.gov/ij/) using the particle analysis function with a consistently applied threshold on all microscope images of seeds taken with identical magnification and exposure time within experiments (Herridge et al., 2011). For seed size distributions, the percentage of seeds within bins at 0.002-mm2 intervals was determined and a running average over six bins was plotted. For controlled pollination, preanthesis flowers (Smyth et al., 1990; stage 12) were emasculated using forceps. One day after emasculation, the pollen from the donor genotype was applied to the stigma of the recipient genotype. Seed development and seed set were determined by dissecting developing siliques and dehisced siliques, respectively (Groszmann et al., 2008; Kay et al., 2013). For the grafting experiment, the bolt harvest index was determined as the seed mass as a proportion of total bolt biomass (seed mass/total bolt dry mass including seed).
Vector Construction
To generate the pCEPR1:GUS reporter, the 2 kb of sequence upstream of the start codon of CEPR1 was amplified from Col-0 gDNA, cloned into pENTR/D-TOPO, and then transferred into the pHGWFS7 destination vector (Karimi et al., 2002) by LR recombination. To generate the CEPR1 complementation construct, the kanamycin marker for plant selection in the pBI121 vector was first removed by digestion with PmeI and ApaI and was replaced using Gibson Assembly (New England Biolabs) with a Basta selection marker amplified from the MIGS2.1 vector (de Felippes et al., 2012). Using Gibson Assembly, the Col-0 CEPR1 genomic fragment and 2 kb of upstream sequence was cloned upstream of a NOS terminator in the modified pBI121 vector cut with SacI and ClaI. Primer sequences used for cloning are listed in Supplemental Table S1.
Plant Transformation and Introgression of Constructs
Plants were transformed by floral dipping (Clough and Bent, 1998) with the Agrobacterium tumefaciens strain LBA4404 harboring the described vectors to create the pCEPR1:GUS reporter line (No-0 background) and the cepr1-1 complementation lines (comp 1 and comp 2). Due to the severely impaired female fertility in cepr1-3, which prevented the direct transformation of this mutant, the complementation lines in the cepr1-3 background (comp 3 and comp 4) were generated by crossing with wild-type Col-0 transformed with the complementation construct. Flowers from independent Col-0 lines harboring the CEPR1 complementation construct were emasculated and then pollinated using cepr1-3 pollen. F2 plants homozygous for the cepr1-3 allele (i.e. no endogenous wild-type CEPR1) and carrying the CEPR1 transgene were identified using PCR genotyping (for primers, see Supplemental Table S1).
Thinning Experiment
Six-week-old No-0 (wild-type) and cepr1-1 plants were thinned by removal of siliques and flowers in addition to secondary and lateral apexes so that only three or four open flowers or very-early-stage siliques remained on the primary stem. Seeds from thinned plants were compared with those from unpruned controls.
Inflorescence Stem (Bolt) Grafting
Bolt grafting was carried out as described by Nisar et al. (2012). Briefly, young No-0 (wild-type) and cepr1-1 bolts (∼80 mm long) were excised ∼20 mm from the base. The end of the donor bolt was cut into a wedge, which was inserted into a vertical incision made in the remaining basal stem section of the recipient stock. The graft junction was stabilized with silicon tubing of 2 to 2.5 mm (internal diameter) and covered with Parafilm to retain moisture. Plants were kept in a humid environment using a plastic covering until grafted bolts had reestablished turgor and growth. Bolts that did not take, or that grew poorly as determined by the appearance of necrotic cauline leaves, were discarded. Secondary branches that formed from the stock after grafting were continuously removed so that the final reproductive organs were derived from the donor bolt only.
Promoter-GUS Reporter Analysis
GUS staining and analysis were performed essentially as previously described (Groszmann et al., 2010, 2011). Briefly, samples were harvested into cold phosphate buffer (pH 7) with 4% (v/v) formaldehyde, washed in cold phosphate buffer, transferred into 2 mm 5-bromo-4-chloro-3-indolyl-β-D-glucuronic acid staining solution, incubated at 37°C for specified durations, and then cleared for 24 h in 70% (v/v) ethanol, fixed for 24 h in 70% (v/v) ethanol plus FAA (3.7% [v/v] formaldehyde and 5% [v/v] acetic acid), washed again, and stored in 70% (v/v) ethanol ready for visualization.
Microscopy
Microscopic imaging was carried out with an M205 FA stereomicroscope with a DFC550 camera (Leica).
Complementation Analyses
T2 complementation lines in the cepr1-1 background (comp 1 and comp 2) were grown alongside wild-type No-0 and cepr1-1. PCR genotyping identified cepr1-1 plants harboring the complementation transgene and null (azygous) segregants. Azygous segregants were pooled with the cepr1-1 plants grown in parallel for the analysis.
For complementation lines in the cepr1-3 background (comp 3 and comp 4), segregating F2 plants were grown alongside wild-type Col-0 and cepr1-3. PCR genotyping identified homozygous cepr1-3 plants harboring the complementation CEPR1 transgene. For the analysis, wild-type Col-0 and cepr1-3 segregants identified from the F2 populations were pooled with the wild-type Col-0 and cepr1-3 plants grown in parallel, respectively. See Supplemental Table S1 for genotyping primers used.
RT-qPCR Analyses
Wild-type and cepr1 primary inflorescence tissue (including stem, inflorescence meristem, buds, and flowers) was harvested for analyses upon opening of the first flower. The lateral organs (cauline leaves, developing branches, and buds) were removed from the harvested tissue before they were snap frozen in liquid nitrogen. Three biological samples per genotype, each containing two bolts, were used, and total RNA was isolated by a modified Trizol extraction method using columns from the RNeasy Plant Mini Kit (Qiagen; Delay et al., 2013b). cDNA synthesis was carried out using oligo(dT)12–18 primers and SuperScript III reverse transcriptase (Invitrogen) and was followed by treatment with RNase H (Invitrogen). For RT-qPCR, Fast SYBR Green fluorescent dye (Applied Biosystems) was used, and samples were run on a ViiA 7 Real-Time PCR System (Applied Biosystems) following the manufacturer’s specifications. Data were analyzed using the ƊƊCT method (Livak and Schmittgen, 2001), with EF1α (At1g07920) expression used for normalization (Czechowski et al., 2005). RT-qPCR primers are listed in Supplemental Table S1.
Accession Numbers
The Arabidopsis Genome Initiative locus codes for the genes discussed in this study are as follows: CEPR1 (AT5G49660), CEPD1 (AT1G06830), CEPD2 (AT2G47880), CEP1 (AT1G47485), CEP2 (AT1G59835), CEP5 (AT5G66815), CEP9 (AT3G50610), UMAMIT11 (AT2G40900), UMAMIT14 (AT2G39510), UMAMIT18/SIAR1 (AT1G44800), PAP1 (AT1G56650), PAP2 (AT1G66390), NIA1 (AT1G77760), GLN1;2 (AT1G66200), AKINBETA1 (AT5G21170), TPS5 (AT4G17770), TPS8 (AT1G70290), TPS10 (AT1G60140), and bZIP11 (AT4G34590).
Supplemental Data
The following supplemental materials are available.
Supplemental Figure S1. CEPR1 mutants have reduced reproductive units per main stem.
Supplemental Figure S2. CEPR1 knockout results in a reduced number of ovules per silique
Supplemental Figure S3. Rosette diameter of plants grown under a short-day (8-h) photoperiod for bolt grafting.
Supplemental Figure S4. CEPR1 controls seed size via activity in the maternal tissue.
Supplemental Table S1. List of oligonucleotides used.
ACKNOWLEDGMENTS
We thank Katia Taylor (Australian National University) and the Grossniklaus lab (University of Zurich) for helpful discussions as well as Nazia Nisar (Australian National University) for bolt grafting technical advice. We thank Bernd Weisshaar (Max Planck Institute for Plant Breeding Research) for the T-DNA mutant line 467C01 and RIKEN for providing the RATM11-2459 line. We thank Aleu Mani George and Baxter Massey (Australian National University) for technical assistance. We acknowledge the National Collaborative Research Infrastructure Strategy of the Australian Government for supporting the Australian National University with the growth facilities utilized as part of the Australian Plant Phenomics Facility.
LITERATURE CITED
Author notes
This work was supported by Australian Research Council (grant nos. DP150104050 and LP150100826 to M.A.D. and grant no. CE140100015 to M.G.). M.T. and K.C. were supported by Australian Government Research Training Program.
Articles can be viewed without a subscription.
Senior author.
The author responsible for distribution of materials integral to the findings presented in this article in accordance with the policy described in the Instructions for Authors (www.plantphysiol.org) is: Michael A. Djordjevic ([email protected]).
M.G., M.A.D., N.I., and M.T. conceived and designed experiments; M.G., M.A.D., and M.T. coordinated the project; M.T performed most of the experiments with assistance from M.G., and K.C. contributed some data to Figure 1; M.T., M.G., and M.A.D. analyzed the data and wrote the article, with contributions from N.I. and K.C.