-
PDF
- Split View
-
Views
-
Cite
Cite
Md Asraful Jahan, Brianna Harris, Matthew Lowery, Aniello M. Infante, Ryan J. Percifield, Nik Kovinich, Glyceollin Transcription Factor GmMYB29A2 Regulates Soybean Resistance to Phytophthora sojae , Plant Physiology, Volume 183, Issue 2, June 2020, Pages 530–546, https://doi.org/10.1104/pp.19.01293
- Share Icon Share
Abstract
Glyceollin isomers I, II, and III are the major pathogen-elicited secondary metabolites (i.e. phytoalexins) of soybean (Glycine max) that, collectively with other 5-deoxyisoflavonoids, provide race-specific resistance to Phytophthora sojae. The NAC-family transcription factor (TF) GmNAC42-1 is an essential regulator of some but not all glyceollin biosynthesis genes, indicating other essential TF(s) of the glyceollin gene regulatory network remain to be identified. Here, we conducted comparative transcriptomics on soybean hairy roots of the variety Williams 82 and imbibing seeds of Harosoy 63 upon treatment with wall glucan elicitor from P. sojae and identified two homologous R2R3-type MYB TF genes, GmMYB29A1 and GmMYB29A2, up-regulated during the times of peak glyceollin biosynthesis. Overexpression and RNA interference silencing of GmMYB29A2 increased and decreased expression of GmNAC42-1, GmMYB29A1, and glyceollin biosynthesis genes and metabolites, respectively, in response to wall glucan elicitor. By contrast, overexpressing or silencing GmMYB29A1 decreased glyceollin I accumulation with marginal or no effects on the expressions of glyceollin synthesis genes, suggesting a preferential role in promoting glyceollin turnover and/or competing biosynthetic pathways. GmMYB29A2 interacted with the promoters of two glyceollin I biosynthesis genes in vitro and in vivo. Silencing GmMYB29A2 in Williams 82, a soybean variety that encodes the resistance gene Rps1k, rendered it compatible with race 1 P. sojae, whereas overexpressing GmMYB29A2 rendered the susceptible Williams variety incompatible. Compatibility and incompatibility coincided with reduced and enhanced accumulations of glyceollin I but not other 5-deoxyisoflavonoids. Thus, GmMYB29A2 is essential for accumulation of glyceollin I and expression of Phytophthora resistance.
Secondary metabolites have important roles in mediating plant-microbe interactions. These include signaling, establishment, and regulation of rhizobial endosymbiosis, and providing resistance to pathogens (Zernova et al., 2014; Cheng et al., 2015). Metabolites that provide resistance to pathogens generally have antibiotic activity. The antibiotic metabolites can be biosynthesized during plant development, independent of the perception of a pathogen (phytoanticipins) or induced by stress or pathogen attack (phytoalexins). Generally, a plant species produces only a few biosynthetically related phytoalexins that putatively provide broad-spectrum activity against bacterial, fungal, and oomycete pathogens. Elegant biochemical studies have correlated phytoalexin accumulation with resistance to pathogens, and more recent genetics studies have supported their antibiotic roles, often narrowing down the identity of the antibiotic metabolite to a few molecules (Graham et al., 2007). Compelling evidence of the role of phytoalexins as important antibiotic molecules in plant defense comes from their toxicity in vitro and the fact that detoxification genes have evolved in pathogens to degrade phytoalexin molecules (Lygin et al., 2010). Further, some pathogens have evolved effectors that directly inhibit their biosynthesis (Zhou et al., 2011). Phytoalexin biosynthesis has been studied in numerous plant species and generally provides a lesion-limiting role to mediate incompatibility (Hammerschmidt, 1999; Großkinsky et al., 2012; Jeandet et al., 2014).
One of the most highly characterized systems for understanding the molecular basis of the phytoalexin resistance against pathogens is the soybean (Glycine max)–Phytophthora sojae interaction. Most genes and enzymes for the biosynthesis of soybean’s major isoflavonoid phytoalexins, the glyceollins, have been well-elucidated (Akashi et al., 2009; Sukumaran et al., 2018), and the organization of enzymes into metabolons is beginning to be understood (Dastmalchi et al., 2016; Mameda et al., 2018). Further, it was in this system that one of the first pathogen-associated molecular pattern elicitors, the cell wall glucan elicitor (WGE) from P. sojae was discovered (Ayers et al., 1976). Putative soybean receptors of WGE have been identified (Fliegmann et al., 2004), and various developmental and environmental parameters that affect the elicitation of glyceollins have been characterized (Bhattacharyya and Ward, 1986; Farrell et al., 2017; Jahan et al., 2019). In a landmark study, Yoshikawa et al. (1978) demonstrated that a critical feature that distinguished resistant versus susceptible soybean genotypes was their ability to rapidly elicit the biosynthesis of three glyceollin. This work provided the foundation for later cellular studies that demonstrated that resistant soybean varieties activated the local biosynthesis of glyceollins more rapidly than susceptible nearly isogenic genotypes (Hahn et al., 1985). More recent studies found that glyceollins can be biosynthesized via two mechanisms that may not be mutually exclusive. The de novo biosynthesis mechanism refers to the up-regulation of genes spanning from PHE AMMONIA LYASE (PAL) to the late-stage glyceollin I-specific biosynthesis gene GLYCINOL 4-DIMETHYLALLYLTRANSFERASE (G4DT; Fig. 1; Moy et al., 2004; Akashi et al., 2009). By contrast, the ‘conversion’ mechanism refers to the hydrolysis of the isoflavone conjugate 6′′-O-malonyldaidzin, that is constitutively biosynthesized, to provide the intermediate daidzein for glyceollin biosynthesis (Fig. 1; Graham et al., 1990; Farrell et al., 2017).
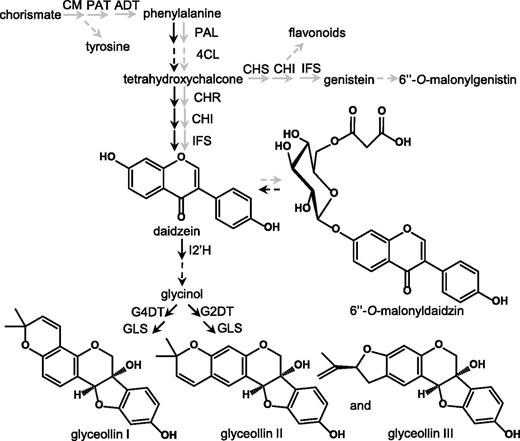
Pathogen-inducible and constitutive isoflavonoid biosynthesis pathways. Elicitors of glyceollin biosynthesis up-regulate transcripts encoding enzymes for glyceollin biosynthesis and/or the hydrolysis of 6′′-O-malonyldaidzin (black arrows). Transcription factors have been identified that regulate the biosynthesis of the constitutively accumulating isoflavone conjugates 6′′-O-malonyldaidzin and 6′′-O-malonylgenistin (gray arrows). CM, chorismatemutase; PAT, prephenate aminotransferase; ADT, arogenate dehydratase; PAL, Phe ammonia lyase; 4CL, 4-coumaric acid: coenzyme A ligase; CHS, chalcone synthase; CHI, chalcone isomerase; CHR, chalcone reductase; IFS, isoflavone synthase; I2′H, isoflavone 2′-hydroxylase; G4DT, glycinol 4-dimethylallyl transferase; G2DT, glycinol 2-dimethylallyl transferase; GLS, glyceollin synthase.
Despite intensive research on glyceollins for decades, the transcription factors (TFs) that regulate their biosynthesis have remained elusive until recently. Several TF genes that regulate the biosynthesis of diverse phytoalexins have been identified but none were homologous among plant species, leading to the notion that phytoalexin TFs were evolutionarily as diverse as the biosynthetic pathways that they regulate. For example, the first identified phytoalexin TF gene GaWRKY1 that regulates gossypol biosynthesis in cotton (Gossypium arboretum) was not homologous to WRKY33, a regulator of the indole alkaloid phytoalexin camalexin from Arabidopsis (Arabidopsis thaliana; Xu et al., 2004; Qiu et al., 2008). The R2R3-type MYB TF genes VvMYB14 and VvMYB15 that regulate stilbene biosynthesis in grapevine (Vitis vinifera) were not homologous to the Arabidopsis camalexin regulators AtMYB34, AtMYB51, and AtMYB122, or to yellow seed (y1) that regulates 3-deoxyanthocyanidin biosynthesis in sorghum (Sorghum bicolor; Höll et al., 2013; Frerigmann et al., 2015; Ibraheem et al., 2015). By comparing the transcriptomes of soybean plants responding to a novel glyceollin biosynthesis elicitor, namely acidity stress, and the suppressor dehydration, we identified the NAC (NAM/ATAF1/2/CUC2)-family TF gene GmNAC42-1. GmNAC42-1 is up- and down-regulated with the glyceollin elicitor and repressor, respectively (Jahan et al., 2019). The GmNAC42-1 protein directly binds the promoter of the glyceollin-specific biosynthetic gene G4DT. GmNAC42-1 is the soybean homolog of the Arabidopsis camalexin biosynthesis regulator ANAC042 (Saga et al., 2012). Thus, GmNAC42-1 and ANAC042 represent the first conserved TF genes found to regulate diverse phytoalexin biosynthesis pathways in plants. Yet, silencing and overexpressing GmNAC42-1 does not affect the expression of all glyceollin biosynthesis genes. Also, overexpressing GmNAC42-1 in the absence of an elicitor does not fully activate glyceollin biosynthesis. This strongly suggests that at least one additional TF is required to fully activate glyceollin biosynthesis (Jahan et al., 2019).
Glyceollins are purified from soybean and have potent anticancer and neuroprotective properties (Bamji and Corbitt, 2017; Seo et al., 2018; Pham et al., 2019). Yet, they may also have important roles in agriculture. P. sojae is a major pathogen of soybean that causes 200 million and 1 to 2 billion dollars in soybean yield loss per year in the United States and worldwide, respectively. The major source of resistance relied upon by breeders has been the resistance to P. sojae (Rps) genes that primarily encode nucleotide binding site Leu-rich repeat (NBS-LRR) receptor proteins that recognize on a gene-for-gene basis the effector proteins encoded by P. sojae avirulence (Avr) genes (Gao et al., 2005; Song et al., 2013). Rps1k has been the most widely relied upon Rps gene by breeders in the major soybean-producing regions of the United States for the last two decades because it has provided resistance to several of the most commonly encountered races of P. sojae (Gao et al., 2005). Soybean genotypes encoding Rps1k, such as Williams 82 (W82) and Harosoy 63 (H63), activate the biosynthesis of glyceollins more rapidly when encountering race 1 P. sojae than other nearly isogenic soybean genotypes that lack Rps1k (Yoshikawa et al., 1978; Hahn et al., 1985). RNA interference (RNAi) silencing of the CHALCONE REDUCTASE (CHR) gene (Fig. 1) has provided genetic evidence that daidzein and/or the glyceollins mediate Rps1k and Rps1c resistance to race 1 P. sojae (Graham et al., 2007). Further, overexpressing the biosynthesis genes 4-COUMARIC ACID: COENZYME A LIGASE (4CL) or ISOFLAVONE REDUCTASE (IFR) that increased the levels of 5-deoxyisoflavonoids enhanced resistance to P. sojae (Cheng et al., 2015; Chen et al., 2019). However, similar to many other plant-pathogen interactions, the exact metabolite(s) responsible for providing resistance have remained unknown. Here, we have identified two TF genes that have roles in regulating the biosynthesis of glyceollin I. We also provide evidence that glyceollin I is the 5-deoxyisoflavonoid responsible for providing resistance to race 1 P. sojae.
RESULTS
WGE Elicits the de Novo and Conversion Mechanisms of Glyceollin Biosynthesis in Different Soybean Tissues
We hypothesized that glyceollin TF candidates could be identified from the transcriptomes of WGE-elicited soybean tissues undergoing the de novo mechanism of glyceollin biosynthesis. We chose to analyze W82 hairy roots since W82 is the source of the reference genome sequence (Schmutz et al., 2010). Also, hairy roots can be readily genetically manipulated to analyze gene function. We also chose imbibing seeds of H63 since our previous experiments suggested that the de novo mechanism was active in this genotype and tissue (Farrell et al., 2017).
W82 hairy roots treated with WGE accumulated glyceollins I, II, and III, whereas no glyceollins were observed from the mock control (Fig. 2A). The amounts of 6′′-O-malonyldaidzin and daidzin dramatically decreased with increasing glyceollin accumulation (Fig. 2B). This indicated that the conversion mechanism was active. Yet, reverse transcription–quantitative PCR (RT-qPCR) demonstrated that GmNAC42-1 and the biosynthesis genes ISOFLAVONE SYNTHASE2 (IFS2) and GLYCINOL 4-DIMETHYLALLYLTRANSFERASE (G4DT) were up-regulated 6.7- to 14.3-fold (Fig. 2C). This suggested that the de novo and the conversion mechanisms were both active in W82 hairy roots. By contrast, glyceollin metabolites and transcripts were up-regulated by WGE in H63 seeds without any decreases in the amounts of daidzein conjugates (Fig. 2, D and E). Thus, both tissues had elevated levels of GmNAC42-1 and biosynthesis gene transcripts and thus fit our criteria to be used to identify TF gene candidates by comparative transcriptomics.
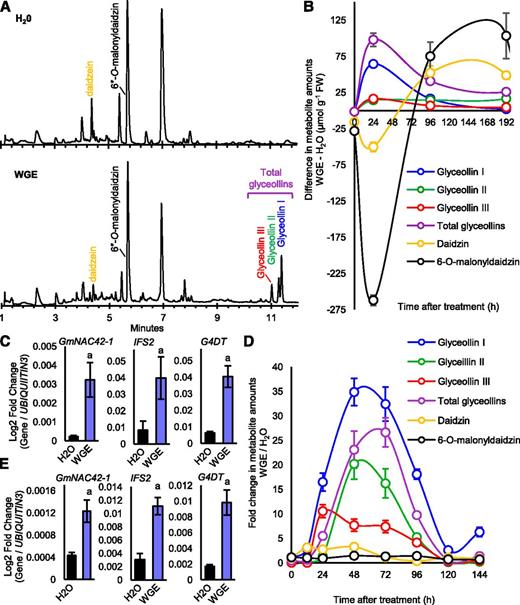
Glyceollin and daidzein-glycoside accumulations in W82 hairy roots and H63 seeds following treatment with P. sojae WGE. A, UPLC-PDA chromatograms at A283 of aqueous ethanolic extracts from W82 hairy roots treated with water or WGE for 24 h. B, Difference in metabolite amounts from W82 hairy roots (the absence of glyceollins in the water control prevented calculation of the fold change). Error bars indicate SE (n = 5 biological replicates). One representative biological experiment out of two is shown. C, Relative gene expression levels in W82 hairy roots 24 h after treatment by RT-qPCR. Lowercase ‘a’ indicates significantly different from water by Student’s t test, P < 0.01. Error bars indicate SE (n = 4 biological replicates). Secondary roots from a single primary root represented one biological replicate since each primary root was derived from a distinct transformation event. D, Fold change of metabolite amounts from H63 seeds. Note, total glyceollin represents the average fold change of the sum of glyceollins I, II, and III in WGE treatment over the mock control. Error bars indicate SE (n = 5 biological replicates). One representative biological experiment out of two is shown. E, Relative gene expression levels in H63 seeds 48 h after treatment. Lowercase ‘a’ indicates significantly different from water by Student’s t test, P < 0.01. Error bars indicate SE (n = 4 biological replicates). One representative biological experiment out of two is shown.
A Distinct Set of TF Genes Is Up-Regulated during Glyceollin Biosynthesis in Response to P. sojae WGE
To identify glyceollin TF candidates, we conducted RNA-sequencing (RNA-seq) on W82 hairy roots and H63 seeds at their respective times of maximum glyceollin accumulation (Fig. 2). We searched for all TF genes that were significantly up-regulated by WGE compared with their respective mock controls (P < 0.05). This identified 5702 and 3852 genes in W82 hairy roots and H63 seeds, respectively, with 1049 genes being commonly up-regulated in both tissues (Fig. 3A; Supplemental Tables S1, S2, and S3). Among the commonly up-regulated genes was the glyceollin TF GmNAC42-1. The transcripts of most biosynthetic genes spanning from the shikimate pathway gene PAT to glyceollin-specific gene G4DT were up-regulated in both tissues (Table 1). A breakdown of the 1049 genes found that ‘Sequence-specific DNA binding TF activity’ was the major gene ontology, comprising 14.3% (Fig. 3B). The myeloblastosis (MYB) family of TFs was the most prevalent in this ontology (14.0%), followed by WRKY family genes (11.3%; Fig. 3C). Among the ten most highly up-regulated TFs were three ETHYLENE RESPONSE FACTOR (ERF) and three MYB family genes (Table 2). Since evidence suggested that ethylene is not a regulator of glyceollin biosynthesis (Paradies et al., 1980), we chose to investigate further the MYB genes.
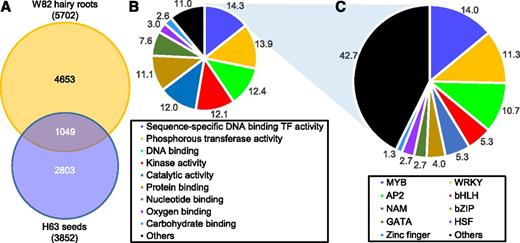
Ontological characterization of TF genes up-regulated by WGE with glyceollins. A, Number of genes significantly up-regulated by P. sojae WGE in H63 germinating seeds and W82 hairy roots determined by RNA-seq (P < 0.05). B, Percentage of genes up-regulated in both tissues in A that were assigned to a particular gene ontology category. Ontology analysis was conducted using the SoyBase.org Gene model Data Mining and Analysis tool. C, Breakdown of genes from the sequence-specific DNA binding TF activity ontology in B into TF gene families, expressed as percentages.
Glyceollin biosynthesis genes up-regulated by WGE in H63 seeds and W82 hairy roots
Pathway . | Gene Symbol . | Enzyme . | Wm82.a2 (Glyma 2.0) . | H63 Seeds . | W82 Hairy Roots . | ||
---|---|---|---|---|---|---|---|
Log2 Fold Change . | P-value . | Log2 Fold Change . | P-value . | ||||
Shikimate | PAT | Prephenate aminotransferase | Glyma.11G238300 | 1.25 | 1.89E−02 | 1.23 | 8.48E−16 |
ADT2 | Arogenate dehydratase | Glyma.12G072500 | 0.64 | 4.67E−03 | 0.34 | 4.44E−02 | |
Phenylpropanoid | PAL | Phe ammonia-lyase | Glyma.10G058200 | 3.17 | 3.79E−33 | 0.75 | 4.89E−05 |
– | – | Glyma.03G181600 | 1.43 | 5.78E−05 | 1.44 | 1.10E−08 | |
– | – | Glyma.03G181700 | 1.32 | 1.10E−02 | 0.72 | 7.07E−05 | |
– | – | Glyma.13G145000 | 1.05 | 1.24E−03 | 0.28 | 3.69E−02 | |
– | – | Glyma.19G182300 | 0.75 | 7.91E−03 | 0.39 | 1.68E−02 | |
C4H | Cinnamic acid 4-hydroxylase | Glyma.20G114200 | 2.66 | 1.20E−07 | 1.17 | 1.00E−22 | |
4CL | 4-Coumarate:coenzyme A | Glyma.11G010500 | 1.38 | 2.76E−02 | 0.86 | 1.08E−08 | |
– | ligase | Glyma.01G232400 | 0.76 | 2.85E−02 | 0.43 | 3.62E−03 | |
Chalcone | CHR | Chalcone reductase | Glyma.02G307300 | 1.56 | 3.05E−02 | 1.81 | 3.00E−23 |
CHI | Chalcone isomerase | Glyma.10G292200 | 2.07 | 1.70E−12 | 0.82 | 1.33E−05 | |
– | – | Glyma.06G143000 | 1.16 | 1.05E−06 | 0.47 | 1.33E−04 | |
– | – | Glyma.04G222400 | 0.81 | 1.45E−06 | 0.32 | 1.99E−02 | |
Isoflavone | IFS1 | Isoflavone synthase | Glyma.07G202300 | 3.78 | 7.06E−45 | 0.30 | 1.75E−03 |
IFS2 | Isoflavone synthase | Glyma.13G173500 | 2.75 | 1.54E−22 | 1.62 | 2.32E−25 | |
HIDH | 2-Hydroxy-isoflavanone dehydratase | Glyma.01G239600 | 1.44 | 1.89E-04 | 0.62 | 1.09E−05 | |
I2'H | Isoflavone 2′-hydroxylase | Glyma.15G156100 | 1.67 | 2.16E−02 | 2.31 | 1.31E−12 | |
IFR | Isoflavone reductase | Glyma.01G211800 | 1.74 | 4.13E−03 | 2.49 | 2.83E−21 | |
– | – | Glyma.11G070200 | 1.78 | 6.42E−03 | 1.80 | 1.18E−26 | |
– | – | Glyma.01G172600 | 1.51 | 1.00E−02 | 0.39 | 3.07E−02 | |
Pterocarpan | P6αH | Dihydroxypterocarpan- 6α-hydroxylase | Glyma.19G144700 | 1.69 | 1.32E−04 | 0.72 | 8.57E−05 |
G4DT | Glycinol 4-dimethylallyl-transferase | Glyma.10G295300 | 1.78 | 1.29E−02 | 2.51 | 2.29E−99 | |
Shikimate | PAT | rephenate aminotransferase | Glyma.11G238300 | 1.25 | 1.89E−02 | 1.23 | 8.48E−16 |
Pathway . | Gene Symbol . | Enzyme . | Wm82.a2 (Glyma 2.0) . | H63 Seeds . | W82 Hairy Roots . | ||
---|---|---|---|---|---|---|---|
Log2 Fold Change . | P-value . | Log2 Fold Change . | P-value . | ||||
Shikimate | PAT | Prephenate aminotransferase | Glyma.11G238300 | 1.25 | 1.89E−02 | 1.23 | 8.48E−16 |
ADT2 | Arogenate dehydratase | Glyma.12G072500 | 0.64 | 4.67E−03 | 0.34 | 4.44E−02 | |
Phenylpropanoid | PAL | Phe ammonia-lyase | Glyma.10G058200 | 3.17 | 3.79E−33 | 0.75 | 4.89E−05 |
– | – | Glyma.03G181600 | 1.43 | 5.78E−05 | 1.44 | 1.10E−08 | |
– | – | Glyma.03G181700 | 1.32 | 1.10E−02 | 0.72 | 7.07E−05 | |
– | – | Glyma.13G145000 | 1.05 | 1.24E−03 | 0.28 | 3.69E−02 | |
– | – | Glyma.19G182300 | 0.75 | 7.91E−03 | 0.39 | 1.68E−02 | |
C4H | Cinnamic acid 4-hydroxylase | Glyma.20G114200 | 2.66 | 1.20E−07 | 1.17 | 1.00E−22 | |
4CL | 4-Coumarate:coenzyme A | Glyma.11G010500 | 1.38 | 2.76E−02 | 0.86 | 1.08E−08 | |
– | ligase | Glyma.01G232400 | 0.76 | 2.85E−02 | 0.43 | 3.62E−03 | |
Chalcone | CHR | Chalcone reductase | Glyma.02G307300 | 1.56 | 3.05E−02 | 1.81 | 3.00E−23 |
CHI | Chalcone isomerase | Glyma.10G292200 | 2.07 | 1.70E−12 | 0.82 | 1.33E−05 | |
– | – | Glyma.06G143000 | 1.16 | 1.05E−06 | 0.47 | 1.33E−04 | |
– | – | Glyma.04G222400 | 0.81 | 1.45E−06 | 0.32 | 1.99E−02 | |
Isoflavone | IFS1 | Isoflavone synthase | Glyma.07G202300 | 3.78 | 7.06E−45 | 0.30 | 1.75E−03 |
IFS2 | Isoflavone synthase | Glyma.13G173500 | 2.75 | 1.54E−22 | 1.62 | 2.32E−25 | |
HIDH | 2-Hydroxy-isoflavanone dehydratase | Glyma.01G239600 | 1.44 | 1.89E-04 | 0.62 | 1.09E−05 | |
I2'H | Isoflavone 2′-hydroxylase | Glyma.15G156100 | 1.67 | 2.16E−02 | 2.31 | 1.31E−12 | |
IFR | Isoflavone reductase | Glyma.01G211800 | 1.74 | 4.13E−03 | 2.49 | 2.83E−21 | |
– | – | Glyma.11G070200 | 1.78 | 6.42E−03 | 1.80 | 1.18E−26 | |
– | – | Glyma.01G172600 | 1.51 | 1.00E−02 | 0.39 | 3.07E−02 | |
Pterocarpan | P6αH | Dihydroxypterocarpan- 6α-hydroxylase | Glyma.19G144700 | 1.69 | 1.32E−04 | 0.72 | 8.57E−05 |
G4DT | Glycinol 4-dimethylallyl-transferase | Glyma.10G295300 | 1.78 | 1.29E−02 | 2.51 | 2.29E−99 | |
Shikimate | PAT | rephenate aminotransferase | Glyma.11G238300 | 1.25 | 1.89E−02 | 1.23 | 8.48E−16 |
Pathway . | Gene Symbol . | Enzyme . | Wm82.a2 (Glyma 2.0) . | H63 Seeds . | W82 Hairy Roots . | ||
---|---|---|---|---|---|---|---|
Log2 Fold Change . | P-value . | Log2 Fold Change . | P-value . | ||||
Shikimate | PAT | Prephenate aminotransferase | Glyma.11G238300 | 1.25 | 1.89E−02 | 1.23 | 8.48E−16 |
ADT2 | Arogenate dehydratase | Glyma.12G072500 | 0.64 | 4.67E−03 | 0.34 | 4.44E−02 | |
Phenylpropanoid | PAL | Phe ammonia-lyase | Glyma.10G058200 | 3.17 | 3.79E−33 | 0.75 | 4.89E−05 |
– | – | Glyma.03G181600 | 1.43 | 5.78E−05 | 1.44 | 1.10E−08 | |
– | – | Glyma.03G181700 | 1.32 | 1.10E−02 | 0.72 | 7.07E−05 | |
– | – | Glyma.13G145000 | 1.05 | 1.24E−03 | 0.28 | 3.69E−02 | |
– | – | Glyma.19G182300 | 0.75 | 7.91E−03 | 0.39 | 1.68E−02 | |
C4H | Cinnamic acid 4-hydroxylase | Glyma.20G114200 | 2.66 | 1.20E−07 | 1.17 | 1.00E−22 | |
4CL | 4-Coumarate:coenzyme A | Glyma.11G010500 | 1.38 | 2.76E−02 | 0.86 | 1.08E−08 | |
– | ligase | Glyma.01G232400 | 0.76 | 2.85E−02 | 0.43 | 3.62E−03 | |
Chalcone | CHR | Chalcone reductase | Glyma.02G307300 | 1.56 | 3.05E−02 | 1.81 | 3.00E−23 |
CHI | Chalcone isomerase | Glyma.10G292200 | 2.07 | 1.70E−12 | 0.82 | 1.33E−05 | |
– | – | Glyma.06G143000 | 1.16 | 1.05E−06 | 0.47 | 1.33E−04 | |
– | – | Glyma.04G222400 | 0.81 | 1.45E−06 | 0.32 | 1.99E−02 | |
Isoflavone | IFS1 | Isoflavone synthase | Glyma.07G202300 | 3.78 | 7.06E−45 | 0.30 | 1.75E−03 |
IFS2 | Isoflavone synthase | Glyma.13G173500 | 2.75 | 1.54E−22 | 1.62 | 2.32E−25 | |
HIDH | 2-Hydroxy-isoflavanone dehydratase | Glyma.01G239600 | 1.44 | 1.89E-04 | 0.62 | 1.09E−05 | |
I2'H | Isoflavone 2′-hydroxylase | Glyma.15G156100 | 1.67 | 2.16E−02 | 2.31 | 1.31E−12 | |
IFR | Isoflavone reductase | Glyma.01G211800 | 1.74 | 4.13E−03 | 2.49 | 2.83E−21 | |
– | – | Glyma.11G070200 | 1.78 | 6.42E−03 | 1.80 | 1.18E−26 | |
– | – | Glyma.01G172600 | 1.51 | 1.00E−02 | 0.39 | 3.07E−02 | |
Pterocarpan | P6αH | Dihydroxypterocarpan- 6α-hydroxylase | Glyma.19G144700 | 1.69 | 1.32E−04 | 0.72 | 8.57E−05 |
G4DT | Glycinol 4-dimethylallyl-transferase | Glyma.10G295300 | 1.78 | 1.29E−02 | 2.51 | 2.29E−99 | |
Shikimate | PAT | rephenate aminotransferase | Glyma.11G238300 | 1.25 | 1.89E−02 | 1.23 | 8.48E−16 |
Pathway . | Gene Symbol . | Enzyme . | Wm82.a2 (Glyma 2.0) . | H63 Seeds . | W82 Hairy Roots . | ||
---|---|---|---|---|---|---|---|
Log2 Fold Change . | P-value . | Log2 Fold Change . | P-value . | ||||
Shikimate | PAT | Prephenate aminotransferase | Glyma.11G238300 | 1.25 | 1.89E−02 | 1.23 | 8.48E−16 |
ADT2 | Arogenate dehydratase | Glyma.12G072500 | 0.64 | 4.67E−03 | 0.34 | 4.44E−02 | |
Phenylpropanoid | PAL | Phe ammonia-lyase | Glyma.10G058200 | 3.17 | 3.79E−33 | 0.75 | 4.89E−05 |
– | – | Glyma.03G181600 | 1.43 | 5.78E−05 | 1.44 | 1.10E−08 | |
– | – | Glyma.03G181700 | 1.32 | 1.10E−02 | 0.72 | 7.07E−05 | |
– | – | Glyma.13G145000 | 1.05 | 1.24E−03 | 0.28 | 3.69E−02 | |
– | – | Glyma.19G182300 | 0.75 | 7.91E−03 | 0.39 | 1.68E−02 | |
C4H | Cinnamic acid 4-hydroxylase | Glyma.20G114200 | 2.66 | 1.20E−07 | 1.17 | 1.00E−22 | |
4CL | 4-Coumarate:coenzyme A | Glyma.11G010500 | 1.38 | 2.76E−02 | 0.86 | 1.08E−08 | |
– | ligase | Glyma.01G232400 | 0.76 | 2.85E−02 | 0.43 | 3.62E−03 | |
Chalcone | CHR | Chalcone reductase | Glyma.02G307300 | 1.56 | 3.05E−02 | 1.81 | 3.00E−23 |
CHI | Chalcone isomerase | Glyma.10G292200 | 2.07 | 1.70E−12 | 0.82 | 1.33E−05 | |
– | – | Glyma.06G143000 | 1.16 | 1.05E−06 | 0.47 | 1.33E−04 | |
– | – | Glyma.04G222400 | 0.81 | 1.45E−06 | 0.32 | 1.99E−02 | |
Isoflavone | IFS1 | Isoflavone synthase | Glyma.07G202300 | 3.78 | 7.06E−45 | 0.30 | 1.75E−03 |
IFS2 | Isoflavone synthase | Glyma.13G173500 | 2.75 | 1.54E−22 | 1.62 | 2.32E−25 | |
HIDH | 2-Hydroxy-isoflavanone dehydratase | Glyma.01G239600 | 1.44 | 1.89E-04 | 0.62 | 1.09E−05 | |
I2'H | Isoflavone 2′-hydroxylase | Glyma.15G156100 | 1.67 | 2.16E−02 | 2.31 | 1.31E−12 | |
IFR | Isoflavone reductase | Glyma.01G211800 | 1.74 | 4.13E−03 | 2.49 | 2.83E−21 | |
– | – | Glyma.11G070200 | 1.78 | 6.42E−03 | 1.80 | 1.18E−26 | |
– | – | Glyma.01G172600 | 1.51 | 1.00E−02 | 0.39 | 3.07E−02 | |
Pterocarpan | P6αH | Dihydroxypterocarpan- 6α-hydroxylase | Glyma.19G144700 | 1.69 | 1.32E−04 | 0.72 | 8.57E−05 |
G4DT | Glycinol 4-dimethylallyl-transferase | Glyma.10G295300 | 1.78 | 1.29E−02 | 2.51 | 2.29E−99 | |
Shikimate | PAT | rephenate aminotransferase | Glyma.11G238300 | 1.25 | 1.89E−02 | 1.23 | 8.48E−16 |
The most highly up-regulated TF genes by P. sojae WGE in H63 germinating seeds and in W82 hairy roots
Gene Symbol . | Gene Family . | Wm82.a2 (Glyma 2.0) . | H63 Seeds . | W82 Hairy Roots . | ||
---|---|---|---|---|---|---|
log2 Fold Change . | P-value . | log2 Fold Change . | P-value . | |||
GmERF15 | ETHYLENE RESPONSE FACTOR | Glyma.11G036500 | 3.523 | 2.18E−10 | 0.648 | 4.91E−03 |
GmMYB29A1 | MYB | Glyma.10G006600 | 3.329 | 2.77E−10 | 2.483 | 1.66E−17 |
GmCRF1-like1 | ETHYLENE RESPONSE FACTOR | Glyma.02G016100 | 3.260 | 2.22E−33 | 0.723 | 9.25E−05 |
GmGBF1-like | G-BOX BINDING FACTOR | Glyma.01G177400 | 3.178 | 5.36E−10 | 1.385 | 3.06E−05 |
GmHBP1b | CAMP-RESPONSE FACTOR | Glyma.05G195200 | 3.163 | 3.18E−09 | 1.177 | 6.58E−03 |
GmHSF2A | HEAT SHOCK FACTOR | Glyma.01G143500 | 2.891 | 2.22E−09 | 1.410 | 4.82E−08 |
GmMYB29A2 | MYB | Glyma.02G005600 | 2.806 | 6.43E−07 | 0.873 | 5.27E−10 |
GmMYB363 | MYB | Glyma.01G224900 | 2.760 | 3.20E−09 | 3.008 | 1.67E−30 |
GmCRF1-like2 | ETHYLENE RESPONSE FACTOR | Glyma.19G213100 | 2.701 | 8.60E−12 | 1.200 | 5.18E−04 |
GmWRKY72 | WRKY | Glyma.17G097900 | 2.516 | 5.13E−07 | 0.389 | 5.86E−03 |
Gene Symbol . | Gene Family . | Wm82.a2 (Glyma 2.0) . | H63 Seeds . | W82 Hairy Roots . | ||
---|---|---|---|---|---|---|
log2 Fold Change . | P-value . | log2 Fold Change . | P-value . | |||
GmERF15 | ETHYLENE RESPONSE FACTOR | Glyma.11G036500 | 3.523 | 2.18E−10 | 0.648 | 4.91E−03 |
GmMYB29A1 | MYB | Glyma.10G006600 | 3.329 | 2.77E−10 | 2.483 | 1.66E−17 |
GmCRF1-like1 | ETHYLENE RESPONSE FACTOR | Glyma.02G016100 | 3.260 | 2.22E−33 | 0.723 | 9.25E−05 |
GmGBF1-like | G-BOX BINDING FACTOR | Glyma.01G177400 | 3.178 | 5.36E−10 | 1.385 | 3.06E−05 |
GmHBP1b | CAMP-RESPONSE FACTOR | Glyma.05G195200 | 3.163 | 3.18E−09 | 1.177 | 6.58E−03 |
GmHSF2A | HEAT SHOCK FACTOR | Glyma.01G143500 | 2.891 | 2.22E−09 | 1.410 | 4.82E−08 |
GmMYB29A2 | MYB | Glyma.02G005600 | 2.806 | 6.43E−07 | 0.873 | 5.27E−10 |
GmMYB363 | MYB | Glyma.01G224900 | 2.760 | 3.20E−09 | 3.008 | 1.67E−30 |
GmCRF1-like2 | ETHYLENE RESPONSE FACTOR | Glyma.19G213100 | 2.701 | 8.60E−12 | 1.200 | 5.18E−04 |
GmWRKY72 | WRKY | Glyma.17G097900 | 2.516 | 5.13E−07 | 0.389 | 5.86E−03 |
Gene Symbol . | Gene Family . | Wm82.a2 (Glyma 2.0) . | H63 Seeds . | W82 Hairy Roots . | ||
---|---|---|---|---|---|---|
log2 Fold Change . | P-value . | log2 Fold Change . | P-value . | |||
GmERF15 | ETHYLENE RESPONSE FACTOR | Glyma.11G036500 | 3.523 | 2.18E−10 | 0.648 | 4.91E−03 |
GmMYB29A1 | MYB | Glyma.10G006600 | 3.329 | 2.77E−10 | 2.483 | 1.66E−17 |
GmCRF1-like1 | ETHYLENE RESPONSE FACTOR | Glyma.02G016100 | 3.260 | 2.22E−33 | 0.723 | 9.25E−05 |
GmGBF1-like | G-BOX BINDING FACTOR | Glyma.01G177400 | 3.178 | 5.36E−10 | 1.385 | 3.06E−05 |
GmHBP1b | CAMP-RESPONSE FACTOR | Glyma.05G195200 | 3.163 | 3.18E−09 | 1.177 | 6.58E−03 |
GmHSF2A | HEAT SHOCK FACTOR | Glyma.01G143500 | 2.891 | 2.22E−09 | 1.410 | 4.82E−08 |
GmMYB29A2 | MYB | Glyma.02G005600 | 2.806 | 6.43E−07 | 0.873 | 5.27E−10 |
GmMYB363 | MYB | Glyma.01G224900 | 2.760 | 3.20E−09 | 3.008 | 1.67E−30 |
GmCRF1-like2 | ETHYLENE RESPONSE FACTOR | Glyma.19G213100 | 2.701 | 8.60E−12 | 1.200 | 5.18E−04 |
GmWRKY72 | WRKY | Glyma.17G097900 | 2.516 | 5.13E−07 | 0.389 | 5.86E−03 |
Gene Symbol . | Gene Family . | Wm82.a2 (Glyma 2.0) . | H63 Seeds . | W82 Hairy Roots . | ||
---|---|---|---|---|---|---|
log2 Fold Change . | P-value . | log2 Fold Change . | P-value . | |||
GmERF15 | ETHYLENE RESPONSE FACTOR | Glyma.11G036500 | 3.523 | 2.18E−10 | 0.648 | 4.91E−03 |
GmMYB29A1 | MYB | Glyma.10G006600 | 3.329 | 2.77E−10 | 2.483 | 1.66E−17 |
GmCRF1-like1 | ETHYLENE RESPONSE FACTOR | Glyma.02G016100 | 3.260 | 2.22E−33 | 0.723 | 9.25E−05 |
GmGBF1-like | G-BOX BINDING FACTOR | Glyma.01G177400 | 3.178 | 5.36E−10 | 1.385 | 3.06E−05 |
GmHBP1b | CAMP-RESPONSE FACTOR | Glyma.05G195200 | 3.163 | 3.18E−09 | 1.177 | 6.58E−03 |
GmHSF2A | HEAT SHOCK FACTOR | Glyma.01G143500 | 2.891 | 2.22E−09 | 1.410 | 4.82E−08 |
GmMYB29A2 | MYB | Glyma.02G005600 | 2.806 | 6.43E−07 | 0.873 | 5.27E−10 |
GmMYB363 | MYB | Glyma.01G224900 | 2.760 | 3.20E−09 | 3.008 | 1.67E−30 |
GmCRF1-like2 | ETHYLENE RESPONSE FACTOR | Glyma.19G213100 | 2.701 | 8.60E−12 | 1.200 | 5.18E−04 |
GmWRKY72 | WRKY | Glyma.17G097900 | 2.516 | 5.13E−07 | 0.389 | 5.86E−03 |
Gene Expression and Phylogeny of Candidate Glyceollin Biosynthesis Regulators GmMYB29A1 and GmMYB29A2
The two most highly up-regulated MYB genes were GmMYB29A1 and GmMYB29A2 (Table 2). WGE increased their expressions 3.33- and 2.81-fold in H63 seeds and 2.48- and 0.87-fold in W82 hairy roots, respectively. RT-qPCR confirmed their up-regulation by WGE treatment in both tissues (Fig. 4A). The G. max RNA-seq Atlas (SoyBase.org) demonstrated that the expression of both genes was not developmentally regulated, like G4DT, and unlike the isoflavone glycoside biosynthesis and TF genes HIDH and GmMYB176 (Fig. 4B). During the course of our study, a closely related soybean MYB gene GmMYB29 was implicated by the genome-wide association study in positively regulating isoflavone glycoside biosynthesis in developing soybean organs (Chu et al., 2017). The RNA-seq Atlas revealed that GmMYB29 expression was developmentally regulated like HIDH and GmMYB176 (Fig. 4B).
![Expression levels and phylogeny of GmMYB29 genes. A, Gene expression in W82 hairy roots 24 h after treatment with WGE measured by RT-qPCR. Lowercase ‘a’ indicates significantly greater than control, paired Student’s t test (P < 0.01). Error bars indicate SE (n = 4 biological replicates). Secondary roots from a single primary root represented one biological replicate since each primary root is derived from a distinct transformation event. B, Gene expressions in developing soybean organs from the RNA-Seq Atlas of G. max (Soybase.org). G4DT is a marker of stress inducible glyceollin biosynthesis, whereas HIDH is a marker of developmentally regulated isoflavone glycoside biosynthesis. C, Unrooted phylogenetic tree of GmMYB29A1 and GmMYB29A2 proteins with representative Arabidopsis and other characterized MYBs. Accession numbers are listed in Supplemental Table S4. D, Amino acid alignment of GmMYB29A1 and GmMYB29A2 by Clustal Omega. R2R3 MYB repeats and SG2 stress-responsive domains are shaded in black and gray, respectively. Only 50% of the residues of the [DE]Lx2[RK]x3Lx6Lx3R motif that mediates interaction with bHLH proteins in other MYB proteins were conserved in GmMYB29A1 and GmMYB29A2 (red boxes). Amino acids that have different charges in GmMYB29A1 compared with GmMYB29A2 are shaded in red. Amino acid 192 of the SG2 domain that differs between GmMYB29A1 and GmMYB29A1 is marked with a black circle. Residues that are fully conserved (*), strongly similar (colon [:]; >0.5 in the GonnetPAM 250 matrix), and weakly similar (period [.]; GonnetPAM <0.5 and >0).](https://oup.silverchair-cdn.com/oup/backfile/Content_public/Journal/plphys/183/2/10.1104_pp.19.01293/2/m_plphys_v183_2_530_f4.jpeg?Expires=1750252539&Signature=p8exvtWM~koa584lOFcDT2yhKJyd5tpsbMRVOsKbxuzTAYa47eKp1oVQm4CogmV9AECGtWdY2V13eJUugOuzK~tFPjTZNeDCGs4jEN0ewgSN8-31M6dWSX6jnkZLEJMYTFLbjcLF-8VXjzJ9FaY-LO9sBbAml7NkDA9H3EFS0SQIKn5lWJpkiEl8p7OOZBUZJNDlkVxSsfL5lc5gvB0q1HKBzKo7Slcb9sXvrkqT0IB2p9ato2KppSoifDg7sFgFQew9d2yqjDOieIa27JfkrOzvYdGogC2ieRr5F9zmmxzTrzwel9jhpx-NTP6Sj-qyTv6QS30lA9qoZ1bVtZ2oIQ__&Key-Pair-Id=APKAIE5G5CRDK6RD3PGA)
Expression levels and phylogeny of GmMYB29 genes. A, Gene expression in W82 hairy roots 24 h after treatment with WGE measured by RT-qPCR. Lowercase ‘a’ indicates significantly greater than control, paired Student’s t test (P < 0.01). Error bars indicate SE (n = 4 biological replicates). Secondary roots from a single primary root represented one biological replicate since each primary root is derived from a distinct transformation event. B, Gene expressions in developing soybean organs from the RNA-Seq Atlas of G. max (Soybase.org). G4DT is a marker of stress inducible glyceollin biosynthesis, whereas HIDH is a marker of developmentally regulated isoflavone glycoside biosynthesis. C, Unrooted phylogenetic tree of GmMYB29A1 and GmMYB29A2 proteins with representative Arabidopsis and other characterized MYBs. Accession numbers are listed in Supplemental Table S4. D, Amino acid alignment of GmMYB29A1 and GmMYB29A2 by Clustal Omega. R2R3 MYB repeats and SG2 stress-responsive domains are shaded in black and gray, respectively. Only 50% of the residues of the [DE]Lx2[RK]x3Lx6Lx3R motif that mediates interaction with bHLH proteins in other MYB proteins were conserved in GmMYB29A1 and GmMYB29A2 (red boxes). Amino acids that have different charges in GmMYB29A1 compared with GmMYB29A2 are shaded in red. Amino acid 192 of the SG2 domain that differs between GmMYB29A1 and GmMYB29A1 is marked with a black circle. Residues that are fully conserved (*), strongly similar (colon [:]; >0.5 in the GonnetPAM 250 matrix), and weakly similar (period [.]; GonnetPAM <0.5 and >0).
A phylogenetic analysis of GmMYB29A1 and GmMYB29A2 with representative proteins from each of the MYB subgroups indicated that they were most closely related to the R2R3-type MYBs AtMYB14 and VvMYB14 (Fig. 4C). Protein accession numbers are listed in Supplemental Table S4. AtMYB14 regulates cold tolerance in Arabidopsis, and VvMYB14 regulates stilbene phytoalexin biosynthesis in grapevine, like VvMYB15 (Chen et al., 2013; Höll et al., 2013). Other more distantly related proteins in this cluster included AtMYB34, AtMYB51, and AtMYB122 that indirectly regulate the biosynthesis of camalexin phytoalexins in Arabidopsis (Frerigmann et al., 2015), AtPAP1/AtMYB75 for anthocyanin biosynthesis (Borevitz et al., 2000), and AtPFG1/AtMYB12, AtPFG2/AtMYB11, and AtPFG3/AtMYB111 for flavonol glycoside biosynthesis (Stracke et al., 2001). An outlier was GmMYB363, whose gene was also highly up-regulated by WGE in both H63 seeds and W82 hairy roots (Table 2). The isoflavonoid regulator GmMYB176 was not found in this cluster.
Amino acid sequence analysis found that GmMYB29A1 and GmMYB29A2 proteins encoded R2R3 DNA binding domains in their N-terminal regions where they were 100% identical to each other and 91% identical to GmMYB29 (Fig. 4D). Some R2R3 MYB TFs such as the anthocyanin regulator AtPAP1/AtMYB75 physically associate into MYB-bHLH-WDR complexes and require physical interaction with a bHLH through the [DE]Lx2[RK]x3Lx6Lx3R motif to bind DNA (Zimmermann et al., 2004; Xu et al., 2015). GmMYB29A1 and GmMYB29A2 only encoded 50% of the residues of this motif. In their C termini, GmMYB29A1 and GmMYB29A2 were 83% identical (86% similar) to each other and 49% identical (59% similar) to GmMYB29 (Fig. 4D). All three proteins contained the SG2 (DxSFW–MxFWFD) motif putatively associated with stress responses (Stracke et al., 2001; Höll et al., 2013). GmMYB29A2 and GmMYB29A1 had only one amino acid difference in their SG2 motif, specifically at amino acid 192 (Fig. 4D). GmMYB29A2 encoded a Ser at this residue like MYB14 and MYB15 TFs from Arabidopsis, grapevine, and Lotus japonicus, whereas GmMYB29A1 encoded a Pro (Supplemental Fig. S1).
Overall, we selected GmMYB29A1 and GmMYB29A2 for functional analysis since they had similar MYB DNA binding domains as GmMYB29 and thus could potentially regulate some of the same isoflavonoid genes.
GmMYB29A2 Is a Positive Regulator of Glyceollin I Biosynthesis
To evaluate whether GmMYB29A1 and/or GmMYB29A2 regulate glyceollin biosynthesis, we first silenced their gene expression in WGE-treated W82 hairy roots. RNAi triggers were designed to target ∼250 bp of their respective 3′-UTRs (Fig. 5A). A 3.2-fold silencing of GmMYB29A1 did not affect the expression of GmMYB29A2, GmNAC42-1, or glyceollin biosynthesis genes with the exception of a 1.5-fold reduction in the expression of G4DT (Fig. 5B). This was accompanied by a 1.6-fold reduction in the levels of glyceollin I metabolites (Fig. 5C). By contrast, a 2.2-fold silencing of GmMYB29A2 reduced the expressions of GmMYB29A1, GmNAC42-1, IFS2, I2’H, and G4DT from 1.7- to 15.2-fold (Fig. 5D). It also resulted in a 3.4-fold reduction in the amounts of glyceollin I and a 1.5- to 4.9-fold increase in the amounts of 6′′-O-malonyldaidzin, daidzein, and 6′′-O-malonylgenistin (Fig. 5E).
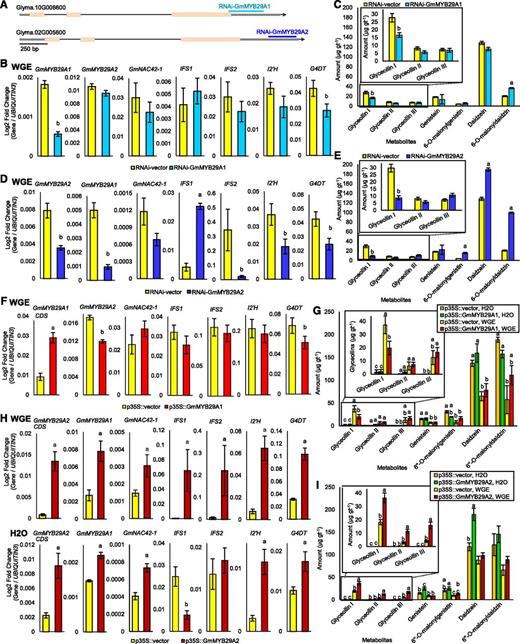
Functional characterization of GmMYB29A1 and GmMYB29A2. A, Schematic diagram of GmMYB29A1 and GmMYB29A2 genes showing the target sites of the RNAi hairpins. B, Gene expression in W82 hairy roots silencing GmMYB29A1 24 h after treatment with WGE or solvent. Lowercase letters indicate significantly greater (a) and significantly less (b) than control, paired Student’s t test (P < 0.01). Error bars indicate SE (n = 4 biological replicates). Secondary roots from a single primary root represented one biological replicate since each primary root is derived from a distinct transformation event. C, Isoflavonoid amounts from W82 RNAi-GmMYB29A1 roots following treatment with P. sojae WGE by UPLC-PDA. Lowercase letters indicate significantly greater (a) and significantly less (b) than control, paired Student’s t test (P < 0.01). Error bars indicate SE (n = 5 biological replicates). D, Gene expression in W82 hairy roots silencing GmMYB29A2 (paired Student’s t test, P < 0.01). Error bars indicate SE (n = 4 biological replicates). E, Isoflavonoid amounts from W82 RNAi-GmMYB29A2 roots (paired Student’s t test, P < 0.01). Error bars indicate SE (n = 5 biological replicates). F, Gene expression in W82 hairy roots overexpressing GmMYB29A1 24 h after treatment with WGE (paired Student’s t test, P < 0.01). Error bars indicate SE (n = 4 biological replicates). Note, due to high similarity between GmMYB29A1 and GmMYB29A2 CDSs, the primers used to measure CDS overexpression could not be designed to be gene specific. However, primers used to amplify native GmMYB29A1 and GmMYB29A2 were gene specific, targeting their unique 3′UTRs, and were used for all measurements with the exception of CDS overexpression. G, Isoflavonoid amounts in W82 p35S::GmMYB29A1 hairy roots 24 h after treatment with WGE or water (solvent) by UPLC-PDA. Different letters show significant differences by single factor ANOVA, Tukey post hoc test (P < 0.05, α = 0.05). Error bars indicate SE (n = 5 biological replicates). H, Gene expression in W82 p35S::GmMYB29A2 hairy roots 24 h after treatment with WGE or water. Lowercase letters indicate significantly greater (a) and significantly less (b) than control, paired Student’s t test (P < 0.01). Error bars indicate SE (n = 4 biological replicates). I, Isoflavonoid amounts in W82 p35S::GmMYB29A2 hairy roots. Different letters show significant differences by single factor ANOVA, Tukey post hoc test (P < 0.05, α = 0.05). Error bars indicate SE (n = 5 biological replicates).
To explore further the functions of GmMYB29A1 and GmMYB29A2, we overexpressed each of their coding sequences (CDSs) in W82 hairy roots using the 35S viral promoter (p35S) to drive transcription. Overexpressing GmMYB29A1 marginally down-regulated GmMYB29A2 and G4DT (1.33- to 1.45-fold) with no other changes in glyceollin gene expressions (Fig. 5F). It also caused a 1.9-fold reduction in glyceollin I metabolites (Fig. 5G). By contrast, the overexpression of GmMYB29A2 led to up-regulation of GmNAC42-1, GmMYB29A1, and all the tested biosynthesis genes in WGE-treated roots (Fig. 5H). In the mock (water)-treated roots, it also up-regulated all genes except for IFS1 and IFS2 (Fig. 5H). It did not result in glyceollins accumulating in water-treated roots, but it enhanced the levels of all glyceollin metabolites 2.0- to 4.2-fold in WGE-treated roots (Fig. 5I). Taken together, these results identify GmMYB29A2 as a positive regulator of GmNAC42-1 that is essential for the full activation of glyceollin I biosynthesis.
GmMYB29A2 Binds the Promoters of Glyceollin Biosynthesis Genes IFS2 and G4DT
To determine if the putative TF GmMYB29A2 is located in nuclei, we cloned its CDS downstream of an N-terminal synthetic GFP tag. We expressed the translational fusion in soybean hairy roots using the constitutively active CaMV-35S promoter (p35S). nGFP-GmMYB29A2 localized predominantly to the nucleus but was also found in the cytosol as shown by colocalization with propidium iodide fluorescence (Supplemental Fig. S2). By contrast, GFP expressed by the empty vector was localized to the cytosol and other extra-nuclear compartments. To determine whether the GmMYB29A2 protein directly binds the promoters of glyceollin biosynthesis genes, we conducted electrophoretic mobility shift assays (EMSAs) using N-terminal human influenza hemagglutinin (HA)-GmMYB29A2 translational fusion proteins purified from Escherichia coli and two labeled oligonucleotide probes encoding highly similar predicted MYB elements from G4DTpro2 and IFS2pro2 promoter regions (Fig. 6A). The HA-GmMYB29A2 protein caused a gel shift for both probes that was completely abrogated by coincubation with a 100-fold excess of unlabeled competitor oligonucleotide (Fig. 6B). Thus, EMSA experiments suggested that GmMYB29A2 can directly bind the promoters of IFS2 and G4DT.
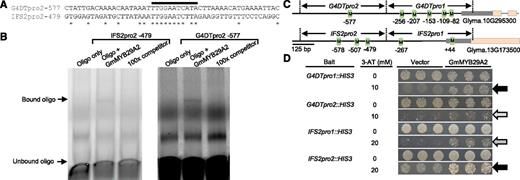
GmMYB29A2 binds glyceollin biosynthesis gene promoters. A, Alignment of 50-nucleotide (nt) oligo sequences that contain the predicted MYB-binding elements G4DTpro2 -577 and IFS2pro2 -479. Stars denote same nucleotide identities. Black bar indicates the predicted MYB-binding elements. B, EMSA of double-stranded G4DTpro2-577 and IFS2pro1-479 oligos with immunoprecipitated (IPed) hemagglutinin (HA)-tagged GmMYB29A2 protein purified from E. coli. Results are representative of two independent experiments. C, Schematic diagram demonstrating G4DT and IFS2 promoter fragments used for yeast one-hybrid assays and predicted MYB binding elements (green boxes). See Supplemental Table S5 for the DNA sequences of MYB elements. D, Y1H assays of YM4271 yeast transformed with GmMYB29A2 or empty vector and containing G4DT or IFS2 promoter segments integrated in the yeast genome upstream of the HIS3 gene. Assays were on SD/-His/-Leu medium containing the listed concentrations of 3-amino-1,2,4-triazole. Gray and black arrows indicate moderate and strong binding, respectively. Results are representative of two to three independent experiments.
To confirm DNA binding, we conducted yeast one-hybrid (Y1H) by expressing a GAL4 activation domain (GAL4AD)-GmMYB29A2 translational fusion in yeast (Saccharomyces cerevisiae) bait strains. The yeast strains contained promoter regions from the isoflavone biosynthesis gene IFS2 or the glyceollin I gene G4DT cloned in the yeast genome upstream of his3-200. An informatics search predicted several MYB recognition elements in the promoter regions (Fig. 6C; Supplemental Table S5). Yeast containing GAL4AD-GmMYB29A2 exhibited more growth than GAL4AD-vector for both G4DT and IFS2 promoter segments (Fig. 6D). Most growth was observed for promoter segments that had the greatest number of predicted MYB recognition elements (compare Fig. 6, C and D). Thus, Y1H indicated that GAL4AD-GmMYB29A2 binds the promoters of G4DT and IFS2.
GmMYB29A2 Mediates Resistance to Race 1 P. sojae
Since 5-deoxyisoflavonoids provide race-specific resistance to P. sojae (Subramanian et al., 2005; Graham et al., 2007), we set out to determine whether the glyceollin regulator GmMYB29A2 has a role in regulating resistance. To do this, we silenced GmMYB29A2 using RNAi in hairy roots of variety W82. W82 has Rps1k-mediated race-specific resistance to race 1 P. sojae. We also overexpressed the GmMYB29A2 CDS in the roots of Williams, a susceptible variety with no known Rps gene (Dorrance et al., 2004; Graham et al., 2007).
A 2.2-fold silencing of GmMYB29A2 in W82 resulted in a 2.4- to 2.7-fold increase in disease progression over a 2- to 4-d period, as measured by the length of water-soaked lesions that spread from the point of inoculation with race 1 P. sojae (Fig. 7A). Water-soaked lesions and mycelium growing out of infected tissues were highly visible in RNAi-GmMYB29A2 roots compared with the empty vector control (Fig. 7B). Metabolite analyses preceding these time points revealed that the control empty vector W82 accumulated 217.5 µg per gram tissue (gt−1) glyceollin I, which is slightly above the effective dose to kill 90% of P. sojae (Yoshikawa et al., 1978), whereas the RNAi-GmMYB29A2 roots accumulated 81.8 µg gt−1 (Fig. 7C), which is slightly below the effective dose to kill 50% (Yoshikawa et al., 1978). No significant reductions in the levels of other 5-deoxyisoflavonoids or genistein-derived isoflavonoids were observed. In dramatic contrast, Williams overexpressing GmMYB29A2 4.1-fold caused a 4.0- to 5.8-fold decrease in disease progression compared with the empty vector control (Fig. 7D). Water-soaked lesions and mycelium growth out of the tissues were readily visible for Williams empty vector roots, but were dramatically reduced in the Williams p35S::GmMYB29A2 roots (Fig. 7E). The amount of glyceollin I in p35S::GmMYB29A2 at 24 h preceding the infection measurements was 110 µg gt−1, which was 2.0-fold higher than the empty vector control (Fig. 7F). No significant increases in the levels of other 5-deoxyisoflavonoids or genistein-derived isoflavonoids were observed. Taken together, these results strongly demonstrate that GmMYB29A2 has a role in regulating glyceollin I biosynthesis and resistance to P. sojae.
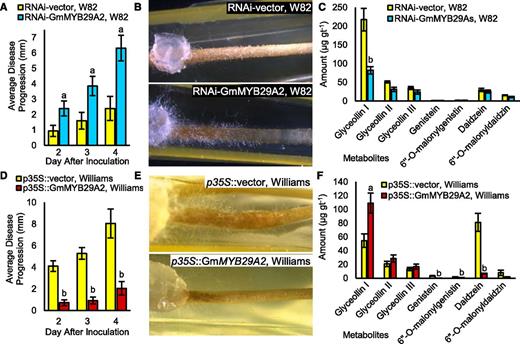
Effects of RNAi silencing or overexpressing GmMYB29A2 on disease progression of race 1 P. sojae in transgenic hairy roots. A, Average lesion length when MYB29A2 gene expression was silenced in hairy roots of W82 (a resistant genotype). Lowercase ‘a’ indicates significantly greater than the p35S::vector control at the same time point, paired Student’s t test (P < 0.01). Error bars indicate SE (n = 4 to 6 biological replicates). Results are representative of two independent experiments. B, Representative images showing disease progression phenotypes. Note the extensive water-soaked lesions and mycelium growth out of root tissues of W82 undergoing RNAi silencing of GmMYB29A2. C, Isoflavonoid amounts from W82 RNAi roots following treatment with race 1 P. sojae for 24 h. Measurements were by UPLC-PDA. Lowercase ‘b’ indicates significantly less than the p35S::vector control, paired Student’s t test (P < 0.01). Error bars indicate SE (n = 6 to 7 biological replicates). D, Average lesion length when MYB29A2 was overexpressed in the susceptible variety Williams. Lowercase ‘b’ indicates significantly less than the p35S::vector control, paired Student’s t test (P < 0.01). Error bars indicate SE (n = 4 to 6 biological replicates). Results are representative of two independent experiments. E, Representative images of (D). Note the massive reduction in water-soaked lesions and mycelium outgrowth in Williams overexpressing GmMYB29A2. F, Isoflavonoid amounts from MYB29A2 overexpressing Williams roots following treatment with race 1 P. sojae for 24 h. Measurements were by UPLC-PDA. Lowercase letters indicate significantly greater (a) and significantly less (b) less than control, paired Student’s t test (P < 0.01). Error bars indicate SE (n = 7 to 8 biological replicates).
DISCUSSION
GmMYB29A2 Is Essential for the Full Elicitation of Glyceollin Biosynthesis
Using RNA-seq we found that transcripts of the R2R3 MYB TF genes GmMYB29A2 and GmMYB29A1 were up-regulated at the time of peak glyceollin biosynthesis upon elicitation with P. sojae WGE in two soybean genotypes and tissues, namely the hairy roots of W82 and in imbibing seeds of H63 (Supplemental Tables S1 and S2). Their up-regulations coincided with those of GmNAC42-1 in both tissues (Supplemental Table S3). GmNAC42-1 is a TF that is essential but insufficient to fully activate glyceollin biosynthesis on its own (Jahan et al., 2019). Silencing either GmMYB29A2 or GmMYB29A1 in W82 hairy roots decreased the accumulations of glyceollin I metabolites (Fig. 5, C and E), but silencing specifically GmMYB29A2 reduced the expression of GmNAC42-1 and most glyceollin biosynthesis genes (Fig. 5D). Further, overexpressing only GmMYB29A2 increased GmNAC42-1 expressions and glyceollin biosynthesis (Fig. 5). Several lines of evidence suggest that GmMYB29A2 regulates specifically the biosynthesis of glyceollin I rather than constitutively biosynthesized isoflavone-glycosides like GmMYB39, GmMYB176, GmMYBJ3, and GmMYB29 (Liu et al., 2013; Chu et al., 2017; Mainali et al., 2017; Zhao et al., 2017). First, GmMYB29A2 expressions were almost undetectable in the absence of an elicitor but were highly up-regulated with glyceollin biosynthesis by WGE (Fig. 4, A and B). Second, overexpressing or silencing its gene expressions in W82 hairy roots increased and decreased, respectively, the levels of glyceollin I metabolites and transcripts of G4DT, a gene that is specific to glyceollin I biosynthesis (Fig. 5, D and H). Third, the GmMYB29A2 protein bound the G4DT promoter in Y1H and EMSA systems (Fig. 6). Finally, overexpressing and silencing GmMYB29A2 in soybean roots inoculated with P. sojae increased and decreased glyceollin I metabolites, respectively, and had no effect on the amounts of isoflavone glycosides (Fig. 7, C and F).
GmMYB29A2 was only highly expressed upon treatment with an elicitor; however, ectopically overexpressing it in the absence of an elicitor provided some insight into its function. Since p35S::GmMYB29A2 up-regulated G4DT in the absence of WGE treatment but did not result in glyceollin biosynthesis, this suggested that it alone cannot up-regulate all genes required to convert daidzein to glyceollins. Genes that may not be up-regulated by GmMYB29A2 include 2-HYDROXYISOFLAVONE REDUCTASE (IFR1/IFR2; Cheng et al., 2015), PTEROCARPAN SYNTHASE (PTS; Uchida et al., 2017), DIHYDROXYPTEROCARPAN 6A-HYDROXYLASE (P6αH/CYP93A1; Schopfer et al., 1998), GLYCINOL 2-DIMETHYLALLYLTRANSFERASE (G2DT; Yoneyama et al., 2016; Sukumaran et al., 2018), and/or GLYCEOLLIN SYNTHASE (GLS; Fig. 1; Welle and Grisebach, 1988). Alternatively, it may not down-regulate the unidentified gene(s) that constitutively degrade glyceollin I (Farrell et al., 2017), or it may not up-regulate genes for the transport of metabolite intermediates between the subcellular compartments where the biosynthetic enzymes are located, including the endoplasmic reticulum (Dastmalchi et al., 2016), plastids (Akashi et al., 2009), and endosomes (Welle and Grisebach, 1988). Our future work will aim to identify all genes that are directly regulated by GmMYB29A2.
Two independent experiments overexpressing GmMYB29A1 in W82 hairy roots demonstrated that GmMYB29A1 slightly down-regulated GmMYB29A2 and G4DT but not other glyceollin biosynthesis genes (Fig. 5). It also reduced glyceollin I metabolite levels. P. sojae elicitation was previously shown by pulse-chase experiments to enhance not only glyceollin I biosynthesis but also its turnover (Bhattacharyya and Ward, 1987). The study suggested that glyceollin I, like several other phytoalexins, was not a pathway 'end product'. Since overexpressing GmMYB29A1 marginally affected the expressions of most glyceollin biosynthetic genes, we suggest that it reduced glyceollin I levels by inducing the expressions of metabolizing enzymes. Thus, the role of GmMYB29A1 may be to limit glyceollin I levels. This could at least in part explain the transient accumulation of glyceollin I upon elicitation (Fig. 2). By contrast, GmMYB29A2 would be required for stimulating glyceollin biosynthesis in part by up-regulating GmNAC42-1.
C-terminal Regions Distinguish the Functions of GmMYB29A2 and GmMYB29A1 in Activating Glyceollin Biosynthesis Genes
GmMYB29A2 and GmMYB29A1 are likely homeologs that have undergone subfunctionalization. Overexpressing GmMYB29A2 and GmMYB29A1 revealed positive and negative effects on glyceollin accumulation (Fig. 5). Since the two proteins are 90% identical (92% similar; Fig. 4D), this raises the question of what structural differences are responsible for their opposite functions.
Various MYBs activate or repress branches of phenylpropanoid biosynthesis by competitively interacting with bHLHs through a [DE]Lx2[RK]x3Lx6Lx3R motif in their N-terminal R3 repeat. This, in turn, is needed for those MYBs to bind DNA (Zimmermann et al., 2004; Ma and Constabel, 2019). Yet, GmMYB29A2 and GmMYB29A1 have identical N-terminal regions (Fig. 4D), and GmMYB29A2 could bind DNA in Y1H and EMSA systems without a bHLH (Fig. 6).
In contrast with their identical N-terminal regions, the C-terminal regions of GmMYB29A1 and GmMYB29A2 were 14% different (86% similar). GmMYB29A1 did not encode either EAR or TLLLFR C-terminal repression motifs that are conserved in other MYBs that repress other branches of phenylpropanoid biosynthesis (Shen et al., 2012; Yoshida et al., 2015; Ma and Constabel, 2019). The SG2 motif was the only conserved motif identified in the C-terminal regions (Fig. 4D). The SG2 motif is associated with inducible phenylpropanoid biosynthesis responses, such as cold- and pathogen-induced lignification in Arabidopsis, pathogen- and UV-C-elicited stilbene biosynthesis in grapevine, and glutathione-induced isoflavan biosynthesis in L. japonicus (Stracke et al., 2001; Shelton et al., 2012; Höll et al., 2013; Chezem et al., 2017). GmMYB29A2 and GmMYB29A1 had only one amino acid difference in their SG2 motif, specifically at amino acid 192 (Fig. 4D). GmMYB29A2 encoded a Ser at this residue like MYB14 and MYB15 TFs from Arabidopsis, grapevine, and L. japonicus, whereas GmMYB29A1 encoded a Pro. The two proteins also had major differences in the charges of 10 amino acids in the C-terminal region outside of the SG2 motif (Fig. 4D). Thus, it is differences in amino acid residues in their C-terminal regions that somehow distinguish the functions of GmMYB29A1 and GmMYB29A2. Since the C-terminal regions of MYBs generally interact with other proteins to activate or repress the transcription of gene targets (Hichri et al., 2011), it is tempting to speculate that the distinct C-terminal regions of GmMYB29A2 and GmMYB29A1 provide different functions by mediating distinct protein-protein interactions. The grapevine homolog VvMYB14 requires VvWRKY03 to fully activate the promoter of STILBENE SYNTHASE (STS; Vannozzi et al., 2018); yet it was not determined whether the proteins physically interact. The soybean homologs of VvWRKY03, namely Glyma.19G094100 and Glyma.16G054400, were up-regulated by WGE in W82 roots, whereas the homolog Glyma.08G011300 was up-regulated in H63 seeds (Supplemental Tables S1 and S2). Our future work will aim to characterize which amino acid(s) distinguish the functions of GmMYB29A2 and GmMYB29A1 and identify interacting proteins.
GmMYB29A2 Mediates Glyceollin I-Based Resistance against P. sojae
Race-specific resistance is broadly relied upon by breeders in plant agriculture to provide incompatibility to some of the most devastating pathogens. Yet R genes trigger numerous defense responses, which makes it difficult to pinpoint the exact cellular component(s) or metabolite(s) responsible for providing resistance. The first reports that glyceollins may provide race-specific resistance to P. sojae came from eloquent biochemical studies that demonstrated that resistant soybean varieties rapidly accumulate toxic levels of glyceollins and that this coincided with the arrest of disease progression (Yoshikawa et al., 1978; Hahn et al., 1985). Yet, these studies did not distinguish individual glyceollin isomers or consider other key metabolites. It was Graham et al. that conclusively narrowed down the metabolites responsible for resistance to the 5-deoxyisoflavonoids daidzein and/or the glyceollins by RNAi silencing the biosynthesis genes CHR and IFS (Fig. 1; Graham et al., 2007). Since then, studies have continued implicating 5-deoxyisoflavonoids in providing race-specific resistance to major soybean pathogens including soybean cyst nematode (SCN; Heterodera glycines), Asian soybean rust (Phakopsora pachyrhizi), and a wide variety of races of P. sojae (Lin et al., 2014; Hossain et al., 2018; Li et al., 2018). Further, overexpressing various 5-deoxyisoflavonoid biosynthesis genes has enhanced resistance to P. sojae races (Sukumaran et al., 2018; Zhou et al., 2018; Chen et al., 2019), yet the metabolite(s) responsible for resistance remained unknown.
Here, we found that silencing the R2R3 MYB TF gene GmMYB29A2 in W82 hairy roots reduced only the amounts of glyceollin I and not the other 5-deoxyisoflavonoids and caused a breakdown of race-specific resistance to P. sojae (Fig. 7, A–C). Conversely, overexpressing GmMYB29A2 increased glyceollin I accumulations in a soybean variety Williams that is universally susceptible to races of P. sojae (Dorrance et al., 2016) and provided resistance to race 1 P. sojae (Fig. 7, D–F). Taken together with Graham et al. (2007), these results strongly suggest that glyceollin I is the 5-deoxyisoflavonoid responsible for W82’s resistance against race 1 P. sojae.
Since overexpressing GmMYB29A2 was sufficient to provide W82-level resistance to Williams hairy roots, it is tempting to speculate that overexpressing GmMYB29A2 can provide nonrace-specific resistance to P. sojae. Glyceollins are toxic to a variety of soybean pathogens in vitro; however, P. sojae is particularly susceptible likely because it lacks the enzyme(s) to catabolize glyceollins (Lygin et al., 2010). Yet, some pathogens such as Pseudomonas syringae have evolved effectors that directly inhibit isoflavonoid biosynthesis enzymes (Zhou et al., 2011). Therefore, the effectiveness of overexpressing GmMYB29A2 to provide crop resistance should be expected to be dependent on the pathogen species. Field trials should be conducted in regions that contain different pathogen microbiomes to test the usefulness of overexpressing GmMYB29A2 in soybean agriculture.
Overexpressing GmMYB29A2 did not result in glyceollin biosynthesis in the absence of P. sojae WGE treatment (Fig. 5I). Thus, it could function by merely priming soybean immunity at the level of up-regulating some but not all glyceollin biosynthesis genes (Fig. 5D). Chemical treatments that prime phytoalexin biosynthesis are commonly used in agriculture to provide resistance to pathogens, and recent research has begun to identify upstream signaling components for genetic priming (Buswell et al., 2018; Schillheim et al., 2018; Van der Ent et al., 2018; Wang et al., 2018; Agostini et al., 2019). Here, our data suggest that overexpressing a downstream direct regulator of phytoalexin biosynthesis (i.e. GmMYB29A2) is an effective strategy to prime pathogen resistance that could be an alternative to using environmentally unfriendly chemical treatments. Further, it could minimize the potential for unintended effects on off-target pathways that are commonly controlled by upstream signaling components. Whether priming immunity by this method would affect yields in a field setting should be topic of future research.
MATERIALS AND METHODS
Chemicals
Stocks of the antibiotics kanamycin, hygromycin-B, and timentin (Gold Biotechnology) were in water. Acetosyringone (Sigma-Aldrich) was 100 mm in dimethyl sulfoxide. (−)-Glyceollin I synthetic standard was purchased from Dr. Paul Erhardt (University of Toledo). Daidzein was from Cayman Chemical, and daidzin, genistin, and genistein were from Indofine. Ultra performance liquid chromatography (UPLC) solvents were liquid chromatography–mass spectrometry grade from Fisher. WGE was extracted from race 1 Phytophthora sojae cultured for 30 to 35 d on lima bean medium as described previously (Farrell et al., 2017).
Maintenance of P. sojae
P. sojae race 1 isolate was a kind gift from Brett Tyler (Oregon State University). The pathogens were maintained on lima bean agar medium. To maintain pathogenicity, P. sojae were grown on lima bean culture plates for 6 to 7 d. Notches were made on the hypocotyl of susceptible soybean (Glycine max) seedlings, which were treated with actively growing P. sojae mycelium. The P. sojae–treated seedlings were wrapped with wet paper, incubated for 24 h, and then transferred to sterile soil. The seedlings were maintained under low intensity fluorescent light for 3 to 4 d, and infected tissue containing P. sojae was transferred to lima bean selection plates containing timentin (500 mg L−1). A mycelial plug (6–7 mm in diameter) was taken from the periphery of a 6- to 7-d-old culture and transferred to a new plate. The cultures were incubated at room temperature for 6 to 7 d under low fluorescent light condition and used for the infection of hairy roots.
Plant Materials and Elicitation
Seeds of Harosoy, H63, Williams, and W82 were obtained from the USDA-GRIN Soybean Germplasm Collection. Bulk seeds of H63 were also a generous gift from Elroy Cober (Agriculture and Agri-Food Canada). For RNA-seq, large, unblemished seeds were surface sterilized and imbibed according to Farrell et al. (2017). The seed coat was carefully removed to avoid damaging the embryo. The distal end (∼2 to 3 mm) of the cotyledons were excised, and a longitudinal incision was made through the axis of the central vein of the cotyledons up to two-thirds of the way toward the hypocotyl-radicle axis. WGE elicitor (20 µg µL−1 in water, 50 μL) or mock solvent was applied to the wound site, and the treated seeds were placed upright on their distal end on sterile water-soaked filter paper in a high petri dish and then incubated for 48 h at 24°C under cool-white T5 fluorescent lights (500 μE m−2 s−1). For hairy roots, following selection on hairy root growth (HRG) medium containing antibiotics (described in the section "Hairy Root Transformation"), the excised 1-cm pieces of secondary roots were placed in petri dishes on HRG medium lacking antibiotics and were overlaid with 80 µL of WGE or water and then incubated for 24 h in the conditions described above. Treated plant materials were used directly for metabolite extractions or were harvested into liquid nitrogen and lyophilized for 3 to 5 d (BenchTop Pro, SP Scientific) before storage at −80°C for RNA extraction.
Isoflavonoid Analyses
Fresh tissues of elicited seeds (150–250 mg) or hairy roots (100 mg) were harvested on ice and immediately extracted with 80% (v/v) ethanol (1 µL mg−1 tissue) according to Farrell et al., 2017. UPLC-PDA-MSn was carried-out using an Accela system (Thermo Scientific) configured with a 1250 pump, Open AS autosampler, and photodiode array (PDA) detector connected to a Q-Exactive–Oribitrap MS containing a HESI. The UPLC-PDA-MSn method and quantification of isoflavonoids by comparison with concentration curves of authentic or in-house purified standards using Xcalibur software (Thermo Scientific) were conducted as previously described (Jahan et al., 2019). Isoflavonoid amounts were measured from five biological replicates in two independent transformation experiments for each overexpression and RNAi silencing of GmMYB29A2 and GmMYB29A1 in roots, and representative results are shown. Secondary roots from a single primary root represented one biological replicate since each primary root is derived from a distinct transformation event. Since the number of hairy roots produced from a cotyledon, their vigor, and the amount of glyceollins elicited by WGE are influenced by the seed age, the hairy roots for all treatments (e.g. 35S::vector and 35s::MYB29A2) were generated from the same batch of seeds. Also, WGE and water treatments were done at the same time for the genotypes being compared.
RNA Extraction and RT-qPCR Analysis
Total RNA from soybean seeds was extracted using the Spectrum Plant Total RNA Kit (Sigma-Aldrich) following the manufacturer’s protocol with some modification as described previously (Farrell et al., 2017). DNase I (Amplification grade, Invitrogen) treatment of the total RNA (500 ng) was done to remove genomic DNA contamination, and complementary DNA (cDNA) was synthesized using SuperScript II Reverse Transcriptase (Invitrogen) following the manufacturers protocol. RT-qPCR of diluted cDNA templates was carried out using IQ SYBR Supermix as described (Farrell et al., 2017). Briefly, reactions (5 µL) consisted of 1 µL of first-strand cDNA (or untreated RNA) diluted one-fifth to one-tenth, 250 nm of forward and reverse primers, and 2.5 µL of the iQ SYBR Green Supermix (BioRad). RT-qPCR was performed on cDNA from four biological replicates or RNA that was not reverse transcribed to measure for genomic DNA contamination using a 7500 Realtime-PCR System (Applied Biosystems). RT-qPCR had an initial denaturation at 95°C for 10 min, followed by 40 cycles of 95°C for 30 s, 58°C for 1 min, and 72°C for 1 min. The primer efficiency was assessed in preliminary experiments with dilutions of plasmids (GmMYB29A1-pDONR221 or -GmMYB29A2) or cDNAs (Farrell et al., 2017; Jahan et al., 2019) and accepted at a value of r 2 ≥ 0.90. Normalized gene expression was calculated based on cycle threshold (Ct) values using the formula expression = 2^-[Ct(gene) - Ct(UBIQUITIN3)]. To verify the specificity of the RT-qPCR reactions, melting curves were determined subsequent to each reaction, and RT-PCR products for each primer set were fractionated on 2% agarose gels. Primers used in this study are listed in Supplemental Table S6.
RNA Sequencing and Analysis
RNA sequencing and analysis was conducted on four biological replicates of WGE- and water-treated tissues of each H63 seeds and W82 hairy roots. Total RNAs from soybean tissues were isolated the using the Spectrum Plant Total RNA Kit as mentioned above. The concentration and purity of extracted total RNA was determined using a NanoDrop 2000 spectrophotometer (Thermo Scientific). The purity of RNA samples was measured by A260/A280 and A260/A230 ratios (>2.0), which indicate lower carbohydrate carryover and protein contamination, respectively. Total RNA samples that exhibited higher quality and purity were selected and sent to the Genomics Core Facility at WV University for library preparation. The quantity of each total RNA sample was more accurately measured using a Qubit fluorometer while RNA quality was ascertained on an Agilent 2100 Bioanalyzer using an RNA Nano 6000 chip. All of the samples used for library prep had RIN (RNA Integrity Number) values >8.0. The mRNA stranded library prep kit from KAPA Biosystems and 750 ng of total RNA were used to build each library following the manufacturer-recommended protocol with nine cycles of PCR. The cDNA libraries were constructed and quantified via the Qubit, pooled in equimolar ratios, and sent to the Genomics Core Facility at Marshall University, where eight libraries were sequenced per lane and 100 bp paired-end reads were generated with the Illumina HiSeq1500 system.
The initial QC checks were conducted upon receiving the raw data from the sequencing facility using FastQC software. rRNA contamination, adapter sequences, and/or low expressed genes were filtered out from the raw high-throughput RNA sequencing data. The clean reads were mapped/aligned to the soybean reference genome (Gmax_275_V2.0.fa; https://phytozome.jgi.doe.gov/pz/portal.html) using STAR RNA-seq Aligner with default parameters based on the current gene annotation. Only the paired mapped reads were considered for further analyses, and the number of reads per gene was calculated using Feature Counts to count only reads, which mapped concordantly. Differentially expressed genes were identified using DESeq2 package, which is based in Negative Binomial Distribution. Multiple hypothesis correction was carried out to obtain an adjusted P value at 0.05 with Benjamini Hochberg procedure, which is a powerful tool to decrease the false discovery rate. Principle component analysis, heatmap, and clustering of the samples were also conducted to check the robustness of the analysis. We chose to not employ a 2.0-fold expression level cutoff to identify up-regulated genes since less than 2% and 10% fit this criterion in W82 hairy roots and H63 seeds, respectively. All the RNA-seq data are available in the Gene Expression Omnibus (GEO) database at the National Center for Biotechnology Information (NCBI) under the accession number GSE131686.
Amino Acid Sequence Comparisons
Amino acid sequences were aligned with Clustal Omega (http://www.clustal.org/omega/).
Cloning
The GmMYB29A2 and GmMYB29A1 CDSs were PCR amplified from the cDNA of H63 seeds treated with WGE (24 h) using the attB Adapter PCR protocol (Invitrogen), the 2X Phusion Master Mix (ThermoFisher Scientific), and primers listed in Supplemental Table S6. The PCR amplicons were cloned into the entry vector pDONR221 using BP clonase (Invitrogen). After sequencing the CDSs, entry vectors were LR recombined downstream of the CaMV 35S promoter in the destination vector pGWB2 for overexpression in hairy roots. The GmMYB29A2 CDS was also LR recombined downstream of the GAL4 activation domain and the HA epitome tag in the pDEST-GADT7 vector for expression in yeast (Saccharomyces cerevisiae) for Y1H assays and Escherichia coli for EMSAs, respectively. For Y1H, G4DT and IFS2 promoter regions 1 and 2 flanked by attL4 and attR1 recombination sites were synthesized and recombined into the destination vector pMW#2 using LR clonase (Invitrogen) as described (Jahan et al., 2019). For RNAi silencing, 180-bp and 253-bp regions corresponding to the 3ʹ-UTRs of GmMYB29A1 and GmMYB29A2, respectively, were PCR amplified from cDNA and BP recombined into pDONR221, and after sequencing were LR subcloned into the RNAi destination vector pANDA35HK. Integrations that would form an RNA hairpin when transcribed were confirmed by sequencing as indicated (Kovinich et al., 2012).
Hairy Root Transformation
Germinating seeds were transformed with Agrobacterium rhizogenes strain K599 according to Jahan et al., 2019. Briefly, unblemished seeds were surface sterilized for 30 s with 70% (v/v) isopropanol and soaked in 10% commercial bleach (6% [v/v] sodium hypochlorite) for 5 min with gentle shaking, then rinsed thrice for 1 min with sterile MilliQ-filtered water. Seeds were transferred to filter paper soaked with germination and cocultivation medium consisting of one-half strength Murashige and Skoog (MS) salts, MS vitamins (Caisson Labs), and 1% Suc (pH 5.8) in a sterile petri dish, incubated in the dark for 3 d, and then transferred to a growth room under 16 h cool-white T5 fluorescent lights (100 µE m−2 s−1) at 24°C for 6 to 7 d.
The day before transformation, A. rhizogenes (strain K599) harboring the vector of interest was streaked onto Luria-Bertani (LB) medium agar plates containing kanamycin and hygromycin (50 mg L−1) and grown at 28°C. A. rhizogenes was scraped from the plate and resuspended in phosphate buffer (0.01 m Na2HPO4 and 0.15 m NaCl, pH 7.5) containing acetosyringone (100 µm) to an optical density at 600 nm of 0.5 to 0.8. Unblemished cotyledons were dissected from meristem and hypocotyl tissues, and several 1-mm-deep cuts were made on the adaxial surface of the cotyledon with a scalpel dipped in the A. rhizogenes suspension. Cotyledons were cocultivated adaxial side down on sterile paper towel soaked with germination and cocultivation medium containing 100 μm acetosyringone for 3 d at 22°C in ∼65 µE light on a 16-h photoperiod. Cotyledons were then transferred adaxial side up onto a HRG medium containing half-strength MS salts, vitamins, 3% Suc (pH5.8), 2.4 g L−1 gelzan (Sigma-Aldrich), and 500 mg L−1 timentin. Primary hairy roots with 2 to 3 cm secondary roots were transferred to selection plates containing HRG medium supplemented with kanamycin and hygromycin (50 mg L−1) to select transgenic hairy roots. After 5 to 7 d, secondary hairy roots that grew to 3 to 6 cm were harvested and cut into 1-cm pieces for WGE treatments. Secondary roots from a single primary root represented one biological replicate since each primary root is derived from a distinct transformation event.
Subcellular Localization
Soybean hairy roots transformed with nGFP-pGWB6 or nGFP-GmMYB29A2-pGWB6 were harvested and stained with propidium iodide according to the manufacturer’s instructions (Sigma-Aldrich). Confocal images were acquired using a Nikon A1R Si confocal laser with N-SIM-E, a TiE inverted research microscope, and two-dimensional automatic deconvolution (NIS Elements) was performed on each image using NIS Elements software. Imaging was performed using an Apo oil 60× objective, plus 1.5× optical zoom, and 6× digital zoom. Excitation and emission spectra were 488 nm and 500 to 550 nm for GFP and 488 nm and 570 to 620 nm for propidium iodide, respectively.
Y1H
Bait strains of YM4271 (MATa, ura3–52, his3–200, lys2–801, ade2–101, ade5, trp1–901, leu2–3, 112, tyr1–501, gal4D, gal80D, ade5::hisG) containing G4DT and IFS2 promoter regions that are integrated into the yeast genome were made as described in Jahan et al., 2019 and were transformed with pDEST-GADT7 (Arabidopsis Biological Resource Center [The Ohio State University, Columbus]) or with GmMYB29A2-pDEST-GADT7. Transformants were selected on media lacking His and Leu (SD-His-Leu) and then confirmed by colony PCR. Autoactivation was tested for and positive DNA-protein interactions were determined by growth in SD-His-Leu medium that contained increasing concentrations (5, 10, 20, 40 and 60 mm) of 3-amino-1,2,4-triazole (Fisher Scientific) as described (Jahan et al., 2019). Three biological replicates are shown, and results were confirmed by two independent experiments.
EMSA
BL21(DE3) pLysS E. coli cells (MilliporeSigma) transformed with pDEST-GADT7 or GmMYB29A2-pDEST-GADT7 were grown overnight in a 37°C shaker at 250 rpm in 3 mL of LB broth containing carbenicillin (100 µg mL–1). The culture was transferred into two sterile 250 mL conical flasks containing 30 mL of LB broth with carbenicillin and incubated in a 37°C shaker at 250 rpm until optical density at 600 nm ∼0.80. Protein expression was induced with 1 mm IPTG at 16°C in a shaking incubator at 250 rpm for 16 h. Following centrifugation (5000g for 10 min at 4°C), the pellet was lysed with B-PER reagent (4 mL g –1; Fisher) containing lysozyme (100 µg mL–1; MP Biomedicals), Thermo Scientific Pierce DNase I (2 µL mL–1 from a 2500U mL−1 stock), and Thermo Scientific Halt protease and phosphatase inhibitor cocktail (10 µL mL–1) for 10 to 15 min at room temperature. Lysates were centrifuged at 15,000g for 5 min to remove insoluble proteins. For immunoprecipitation, 200 µL lysate was mixed with 30 µL of Pierce HA Epitope Tag Agarose Conjugate slurry and incubated overnight at 4°C on a rotary mixer at 40 rpm. The resin was pelleted with a 5 to 10 s pulse at 12,000g and washed three times with prechilled Tris-buffered saline solution containing 0.05% Tween 20. The protein was eluted from resin with 30 µL of 3 m NaSCN. The NaSCN was replaced with 500 µL sterile Tris-buffered saline buffer by centrifugation using a 2 kD cutoff filter (Amicon Ultra Centrifugal Filter UFC500324). This was repeated once. Forty microliters of 80% glycerol were mixed into the 40 µL protein solution to fully resuspend. The concentration and purity of the HA-MYB29A2 protein (32.9 kD) was determined using a BCA kit and Mini-Protean TGX Precast Gel (Bio-Rad), respectively.
For EMSAs, the oligo probe sequences were designed corresponding to predicted GmMYB29A2 binding sites in the G4DT and IFS2 gene promoters (http://plantpan2.itps.ncku.edu.tw/). The forward oligo probes (Fig. 6A) were 5ʹ-end-labeled with IRD700 dye by Integrated DNA Technologies (IDT). Forward oligo probes were annealed at equimolar concentrations to their respective 5ʹ-end-IRD700-labeled reverse complementary oligo probes to 100 µm in Duplex buffer (IDT). Purified full-length GmMYB29A2 protein (1 µg) and IRD-labeled oligonucleotide probes (1 µL of an annealed mixture 500 nm) were incubated with binding solution in 20°C incubator for 30 min. The binding solution was prepared with 20 mm HEPES (pH adjusted to 7.5), 50 mm KCl, 5 mm MgCl2, 3.3 μm ZnSO4, 1 mm dithiothreitol, 0.3 mg mL–1 bovine serum albumin, 0.5 μg poly(dI:dC), 5% glycerol, and 0.5% Triton X-100. The competitors (unlabeled oligos) were added at 100× molar excess. The shifts of the DNA-protein band were resolved by running the binding reaction on 6% DNA Retardation Gel (Invitrogen) using 0.5% TBE running buffer at 200V for 40 min at 4°C. The gels were then imaged using an Odyssey Infrared Imager (LI-COR Biosciences).
Infection of Hairy Roots with P. sojae
Primary hairy roots that had 3- to 4-cm branch roots were cut into apical and basal regions. To identify transgenic roots, the basal region having 4 to 8 branch roots was transferred to selection plates containing the antibiotics kanamycin (50 mg L−1), hygromycin (50 mg L−1), and timentin (500 mg L−1). To measure infection by P. sojae on kanamycin- and hygromycin-free medium, the apical region was transferred to one-half strength Murashige and Skoog media, pH 5.8, containing vitamins, 3% (w/v) Suc, gelzan (2.4 g L−1), and timentin. Branch roots (2–3 cm) were inoculated just above the elongation zone for reproducibility (Graham et al., 2007) with 2-mm agar plugs taken from the periphery of virulent P. sojae culture plates. At the site inoculation, a 1.5-cm barrier was placed between the medium and the root-P. sojae interface to prevent P. sojae growth on the medium. Plates were incubated under 16-h light, 8-h dark cycle for the time indicated.
Statistics
The Tukey post hoc test in one-way ANOVA was applied to determine whether any statistically significant differences existed between group means between treatments and genotypes. As mentioned in the figure legends, boxes with different letters were statistically different (at α = 0.05).
Accession Numbers
Accession numbers are as follows: G4DT, GLYMA.10G295300; GmMYB29A1, Glyma.10G006600; GmMYB29A2, Glyma.02G005600; GmMYB176, Glyma.05G032200; GmNAC42-1, Glyma.02G284300; HIDH, GLYMA.01G239600; IFS1, Glyma.07G202300; IFS2, Glyma.13G173500.
Supplemental Data
The following supplemental materials are available.
Supplemental Figure S1. Amino acid alignment of SG2 domains of GmMYB29A2-like proteins by Clustal Omega.
Supplemental Figure S2. Nuclear localization of nGFP-GmMYB29A2 in transgenic soybean hairy roots by confocal fluorescence microscopy.
Supplemental Table S1. Genes upregulated by P. sojae WGE in W82 hairy roots.
Supplemental Table S2. Genes upregulated by P. sojae WGE in H63 germinating seeds.
Supplemental Table S3. Genes upregulated by P. sojae WGE in both W82 hairy roots and H63 germinating seeds.
Supplemental Table S4. Protein accession numbers from Phytozome.
Supplemental Table S5. Predicted MYB recognition elements.
Supplemental Table S6. Primers used in this study.
ACKNOWLEDGMENTS
We acknowledge the West Virginia University Genomics Core Facility for support provided to help make this publication possible, Gustavo MacIntosh and Jessica Hohenstein (Iowa State University) for Agrobacterium rhizogenes strain K599, Wayne Parrott and Tim Chappell (University of Georgia) for the soybean hairy root transformation protocol, Brett Tyler (Oregon State University) for race 1 P. sojae, Erich Grotewold (University of Michigan) for the yeast YM4271, Tsuyoshi Nakagawa (Shimane University) for the pGWB2 vector, the Arabidopsis Biological Resource Center (The Ohio State University, Columbus) for pDEST-GADT7, and Hiroyuki Tsuji (Yokohama City University) and the late Ko Shimamoto for pANDA35HK, and use of the West Virginia University Shared Research Facilities’ UPLC-PDA-MSn. Our sincere thanks to the reviewers for the extremely insightful comments.
LITERATURE CITED
Author notes
This work was supported by West Virginia University start-up funds (to N.K.) and was based upon work that is supported by the USDA | National Institute of Food and Agriculture (NIFA; hatch, accession no. 1010200) and the West Virginia Agricultural and Forestry Experimental Station (project no. WVA00687).
Articles can be viewed without a subscription.
Present address: Department of Genetic Engineering and Biotechnology, Shahjalal University of Science and Technology, 3114 Sylhet, Bangladesh.
Present address: Biology Department, York University, Toronto M3J 1P3, Ontario, Canada.
Senior author.
The author responsible for distribution of materials integral to the findings presented in this article in accordance with the policy described in the Instructions for Authors (www.plantphysiol.org) is: Nik Kovinich ([email protected]).
M.A.J. conducted UPLC-PDA, cloning, statistics, and hairy root transformations; M.A.J. and M.L. conducted the EMSAs; M.A.J., M.L., and N.K. conducted the RT-qPCR; M.A.J. and N.K. interpreted the results; B.H. conducted the Y1H; A.M.I. processed the RNA-seq data; R.J.P. conducted the RNA-seq library preparation; N.K. designed the study, organized and supervised the research, and wrote the manuscript.