-
PDF
- Split View
-
Views
-
Cite
Cite
David Seung, Alberto Echevarría-Poza, Burkhard Steuernagel, Alison M. Smith, Natural Polymorphisms in Arabidopsis Result in Wide Variation or Loss of the Amylose Component of Starch , Plant Physiology, Volume 182, Issue 2, February 2020, Pages 870–881, https://doi.org/10.1104/pp.19.01062
- Share Icon Share
Abstract
Starch granules contain two Glc polymers, amylopectin and amylose. Amylose makes up approximately 10% to 30% (w/w) of all natural starches thus far examined, but mutants of crop and model plants that produce amylose-free starch are generally indistinguishable from their wild-type counterparts with respect to growth, starch content, and granule morphology. Since the function and adaptive significance of amylose are unknown, we asked whether there is natural genetic variation in amylose synthesis within a wild, uncultivated species. We examined polymorphisms among the 1,135 sequenced accessions of Arabidopsis (Arabidopsis thaliana) in GRANULE-BOUND STARCH SYNTHASE (GBSS), encoding the enzyme responsible for amylose synthesis. We identified 18 accessions that are predicted to have polymorphisms in GBSS that affect protein function, and five of these accessions produced starch with no or extremely low amylose (< 0.5% [w/w]). Eight further accessions had amylose contents that were significantly lower or higher than that of Col-0 (9% [w/w]), ranging from 5% to 12% (w/w). We examined the effect of the polymorphisms on GBSS function and uncovered three mechanisms by which GBSS sequence variation led to different amylose contents: (1) altered GBSS abundance, (2) altered GBSS activity, and (3) altered affinity of GBSS for binding PROTEIN TARGETING TO STARCH1—a protein that targets GBSS to starch granules. These findings demonstrate that amylose in leaves is not essential for the viability of some naturally occurring Arabidopsis genotypes, at least over short timescales and under some environmental conditions and open an opportunity to explore the adaptive significance of amylose.
The aim of our work was to shed light on the occurrence of a seemingly redundant but ubiquitous component of starch, the Glc polymer amylose. Starch is the major storage carbohydrate in plants and one of the most abundant biopolymers on Earth. It occurs as semicrystalline, insoluble granules consisting of two Glc polymers: amylopectin and amylose. Amylopectin, the major polymer, has α-1,4-linked linear chains with frequent α-1,6-linked branches. The self-organization of amylopectin chains gives rise to the semicrystalline granule matrix (Zeeman et al., 2010; Pfister and Zeeman, 2016). Amylose consists of long, linear or minimally branched chains of α-1,4-linked Glc residues and is believed to reside in amorphous regions within the granule matrix. Amylose is not necessary for granule formation: mutant plants lacking amylose form essentially normal semicrystalline granules. The amylose content of starch varies between species and organs and is generally 20% to 35% (w/w) of starch in seeds and nonphotosynthetic storage organs (e.g. tubers and storage roots). Starch in leaves is turned over during the day-night cycle and has a lower amylose content than starch of storage organs. In Arabidopsis (Arabidopsis thaliana), starch accumulates primarily in leaves and contains 8% to 10% (w/w) amylose (Zeeman et al., 2002a; Seung et al., 2015). The shoot endodermis and columella cells of root tips also contain starch: the sedimentation of amyloplasts in these cell types is involved in gravity sensing (Vitha et al., 2007; Streb and Zeeman, 2012). Starch also accumulates transiently in developing embryos, but is undetectable in mature seeds (Streb and Zeeman, 2012).
The enzyme responsible for amylose synthesis—GRANULE BOUND STARCH SYNTHASE (GBSS)—differs from the other isoforms of starch synthase that synthesize amylopectin in being exclusively located within starch granules, where it produces amylose within the amylopectin matrix (Denyer et al., 1999; Tatge et al., 1999; Denyer et al., 2001; Hebelstrup et al., 2017). It does not accumulate in the plastid stroma and appears to be unstable when dissociated from starch granules (Smith et al., 2004; Seung et al., 2015). A second protein—PROTEIN TARGETING TO STARCH 1 (PTST1)—is necessary for normal amylose synthesis in Arabidopsis leaves and cassava roots, the only organs studied in this context thus far (Seung et al., 2015; Bull et al., 2018). PTST1 interacts directly with a coiled coil on GBSS and with starch through its C-terminal starch-binding domain (Carbohydrate Binding Module48 [CBM48; Lombard et al., 2014]), and it is proposed to facilitate GBSS localization on starch granules (Lohmeier-Vogel et al., 2008; Seung et al., 2015). Arabidopsis mutants lacking either GBSS or PTST1 produce amylose-free starch, and ptst1 mutants have almost undetectable levels of GBSS (Seung et al., 2015). Storage roots of ptst1 mutants in cassava have lower GBSS abundance on starch, and the amylose content of starch is 30% lower than in wild-type roots (Bull et al., 2018).
The significance of amylose for plant growth and survival remains unknown. Where rigorous comparisons have been made, amylose-free and low-amylose mutants of crop plants prove to have essentially the same growth characteristics, grain/root/tuber weights, and starch contents as their wild-type counterparts (for example in wheat [Triticum durum; Vignaux et al., 2004; Yasui, 2006], barley [Hordeum vulgare; Howard et al., 2014], potato [Solanum tuberosum; Kuipers et al., 1994], rice [Oryza sativa; Zhang et al., 2018], and cassava [Manihot esculenta; Koehorst-van Putten et al., 2012; Karlström et al., 2016]). The maize (Zea mays) and rice mutants (also known as “waxy” or “glutinous” varieties) have been cultivated for centuries for their desirable cooking and eating properties (Olsen and Purugganan, 2002; Stamp et al., 2016). Arabidopsis gbss and ptst1 mutants are indistinguishable from the wild type and have the same starch contents (Seung et al., 2015). Loss of amylose synthesis in all of these species is thus physically and functionally compensated by increased amylopectin synthesis via other isoforms of starch synthase.
Despite the lack of evidence that amylose is required for plant growth and fitness, GBSS and PTST1 are both conserved throughout the plant kingdom, and amylose is present in the starch of all land plants examined. Studies thus far have been on crop species, including those that were selected over long periods for desirable starch properties, and on model plants grown in controlled conditions. The possibility remains that amylose presents a selective advantage in wild species growing in natural environments. To explore the extent of variation in the amylose content of starch in a wild species, we used the genetic resources from the 1,135 sequenced natural accessions of Arabidopsis (1001 Genomes Consortium, 2016) to identify a subset of accessions containing polymorphisms in GBSS, which proved to encompass unprecedented intraspecies variation in amylose content. Most surprisingly, we identified five accessions that produce amylose-free leaf starch. Exploration of the molecular basis of this variation uncovered several independent mechanisms that influence amylose content of starch in natural populations.
RESULTS
Identification of Arabidopsis Accessions with Polymorphisms in GBSS
To explore variation in amylose content of leaf starch in wild Arabidopsis accessions, we looked for natural GBSS alleles containing polymorphisms likely to affect protein function. The existence of SNPs in the GBSS gene was reported previously from a set of 30 accessions, but their impact on amylose synthesis was not assessed (Schwarte et al., 2013). There are now 1135 genetically distinct, sequenced accessions, covering a wide geographical distribution (Horton et al., 2012; 1001 Genomes Consortium, 2016). We used the Polymorph tool and the Arabidopsis 1001 Genomes Browser to discover small nucleotide polymorphisms (SNPs) in the GBSS locus (At1g32900) of all accessions (1001 Genomes Consortium, 2016; https://tools.1001genomes.org/polymorph/index.html). The PARSESNP tool (Taylor and Greene, 2003) and the GBSS gene model (At1g32900.1) were used to predict the effects of SNPs on gene function.
Three accessions had SNPs likely to prevent the production of functional GBSS protein. In the Vash-1 accession (from Vashlovani National Park, Georgia), the GBSS start codon was changed from ATG to TTG, probably preventing the initiation of translation (Fig. 1; Table 1). Two accessions carried mutations at splice donor sites: at the end of exon 5 in Jl-3 (from Brno, Czech Republic) and at the end of exon 10 in IP-Tau-0 (collected near Taüll, Spain). To examine GBSS transcript splicing in Jl-3 and IP-Tau-0, we amplified their GBSS coding sequences from complmentary DNA (cDNA). In Jl-3, the GBSS transcript was correctly spliced (Supplemental Fig. S1), but in IP-Tau-0 the transcript was misspliced and intron 10 was retained. The translation of this transcript would result in a truncated protein, where the last 81 amino acids of GBSS are substituted with 18 amino acids encoded by the retained intron. This mature protein is predicted to be 52.01 kDa, whereas the Col-0 GBSS is 58.48 kDa. We did not find any accession that had lost the GBSS locus completely or any that had premature stop codons or frameshifts within the GBSS coding sequence.
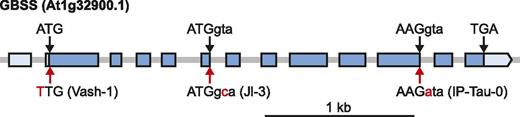
Schematic illustration of the Arabidopsis GBSS locus. Exons are depicted by the blue boxes, while the 5′ and 3′ untranslated regions are depicted by the light blue boxes. The position of the translation start (ATG) and stop (TGA) codons are indicated. The positions of the start codon mutation in Vash-1 and the splice site mutations in Jl-3 and IP-Tau-0 are indicated with red arrows, and the variant nucleotide in these accessions is shown in red below the gene model. The reference sequence (from Col-0) at these positions is shown above the gene model. Exon and intron sequences are depicted in uppercase and lowercase letters, respectively.
Arabidopsis accessions with polymorphisms in GBSS
Amino acid numbering corresponds to the GBSS gene model (At1g32900.1) in the reference accession, Col-0. Amino acid substitutions that are predicted by the PROVEAN program to have a deleterious effect on protein function are shown in bold. Substitutions that occur within the chloroplast transit peptide are indicated in italic font within parentheses.
Accession . | Collection Location . | Mutations . | |||
---|---|---|---|---|---|
Name . | Number . | Country . | Latitude . | Longitude . | . |
Start Codon/Splice Site Mutations | |||||
Vash-1 | 9991 | Georgia | 41.2381 | 46.3728 | Start codon (M1L) |
Jl-3 | 7424 | Czech Republic | 49.2 | 16.6166 | Splice donor at end of exon 5 |
IP-Tau-0 | 9899 | Spain | 42.54 | 0.84 | Splice donor at end of exon 10 |
Amino Acid Substitutions | |||||
Cvi-0 | 6911 | Cape Verde | 15.1111 | −23.6167 | F291L |
IP-Pva-1 | 9888 | Spain | 40.93 | −3.31 | R304Q |
IP-Vas-0 | 9904 | Spain | 40.95 | −3.31 | (N9H, V28L, A29S, G35A, N51K, S66L, R68G), M256I |
Gn2-3 | 9790 | Germany | 48.58 | 9.18 | G394E, N430S |
TueSB30-3 | 9999 | Germany | 48.53 | 9.06 | G394E, N430S |
Ped-0 | 9947 | Spain | 40.74 | −3.9 | (N9H, V28L, A29S, G35A), V403F, A458G |
IP-Ang-0 | 9519 | Spain | 41.94 | 2.64 | (N9H, V28L, A29S, G35A, N51K, S66L, R68G), M256I, K425M |
Amel-1 | 6990 | Netherlands | 53.448 | 5.73 | E452K |
IP-Hue-3 | 9851 | Spain | 42.96 | −6.1 | A458G |
Monte-1 | 9966 | Italy | 40.28 | 15.65 | (N9H, V28L, A29S, G35A, N51K, S66L, R68G), M256I, P467S |
IP-Vis-0 | 9600 | Spain | 39.85 | −6.04 | E208K, P535Q |
IP-Per-0 | 9879 | Spain | 37.6 | −1.12 | E208K, P535R, P574L |
IP-Vaz-0 | 9593 | Spain | 42.26 | −2.99 | R252H, Y552N |
Ra-0 | 6958 | France | 46 | 3.3 | (N9H, H20Q, V28L, A29S, G35A, N51K, S66L, R68G), A556T |
MNF-Pin-39 | 2016 | United States | 43.5356 | −86.1788 | S570Y |
Accession . | Collection Location . | Mutations . | |||
---|---|---|---|---|---|
Name . | Number . | Country . | Latitude . | Longitude . | . |
Start Codon/Splice Site Mutations | |||||
Vash-1 | 9991 | Georgia | 41.2381 | 46.3728 | Start codon (M1L) |
Jl-3 | 7424 | Czech Republic | 49.2 | 16.6166 | Splice donor at end of exon 5 |
IP-Tau-0 | 9899 | Spain | 42.54 | 0.84 | Splice donor at end of exon 10 |
Amino Acid Substitutions | |||||
Cvi-0 | 6911 | Cape Verde | 15.1111 | −23.6167 | F291L |
IP-Pva-1 | 9888 | Spain | 40.93 | −3.31 | R304Q |
IP-Vas-0 | 9904 | Spain | 40.95 | −3.31 | (N9H, V28L, A29S, G35A, N51K, S66L, R68G), M256I |
Gn2-3 | 9790 | Germany | 48.58 | 9.18 | G394E, N430S |
TueSB30-3 | 9999 | Germany | 48.53 | 9.06 | G394E, N430S |
Ped-0 | 9947 | Spain | 40.74 | −3.9 | (N9H, V28L, A29S, G35A), V403F, A458G |
IP-Ang-0 | 9519 | Spain | 41.94 | 2.64 | (N9H, V28L, A29S, G35A, N51K, S66L, R68G), M256I, K425M |
Amel-1 | 6990 | Netherlands | 53.448 | 5.73 | E452K |
IP-Hue-3 | 9851 | Spain | 42.96 | −6.1 | A458G |
Monte-1 | 9966 | Italy | 40.28 | 15.65 | (N9H, V28L, A29S, G35A, N51K, S66L, R68G), M256I, P467S |
IP-Vis-0 | 9600 | Spain | 39.85 | −6.04 | E208K, P535Q |
IP-Per-0 | 9879 | Spain | 37.6 | −1.12 | E208K, P535R, P574L |
IP-Vaz-0 | 9593 | Spain | 42.26 | −2.99 | R252H, Y552N |
Ra-0 | 6958 | France | 46 | 3.3 | (N9H, H20Q, V28L, A29S, G35A, N51K, S66L, R68G), A556T |
MNF-Pin-39 | 2016 | United States | 43.5356 | −86.1788 | S570Y |
Amino acid numbering corresponds to the GBSS gene model (At1g32900.1) in the reference accession, Col-0. Amino acid substitutions that are predicted by the PROVEAN program to have a deleterious effect on protein function are shown in bold. Substitutions that occur within the chloroplast transit peptide are indicated in italic font within parentheses.
Accession . | Collection Location . | Mutations . | |||
---|---|---|---|---|---|
Name . | Number . | Country . | Latitude . | Longitude . | . |
Start Codon/Splice Site Mutations | |||||
Vash-1 | 9991 | Georgia | 41.2381 | 46.3728 | Start codon (M1L) |
Jl-3 | 7424 | Czech Republic | 49.2 | 16.6166 | Splice donor at end of exon 5 |
IP-Tau-0 | 9899 | Spain | 42.54 | 0.84 | Splice donor at end of exon 10 |
Amino Acid Substitutions | |||||
Cvi-0 | 6911 | Cape Verde | 15.1111 | −23.6167 | F291L |
IP-Pva-1 | 9888 | Spain | 40.93 | −3.31 | R304Q |
IP-Vas-0 | 9904 | Spain | 40.95 | −3.31 | (N9H, V28L, A29S, G35A, N51K, S66L, R68G), M256I |
Gn2-3 | 9790 | Germany | 48.58 | 9.18 | G394E, N430S |
TueSB30-3 | 9999 | Germany | 48.53 | 9.06 | G394E, N430S |
Ped-0 | 9947 | Spain | 40.74 | −3.9 | (N9H, V28L, A29S, G35A), V403F, A458G |
IP-Ang-0 | 9519 | Spain | 41.94 | 2.64 | (N9H, V28L, A29S, G35A, N51K, S66L, R68G), M256I, K425M |
Amel-1 | 6990 | Netherlands | 53.448 | 5.73 | E452K |
IP-Hue-3 | 9851 | Spain | 42.96 | −6.1 | A458G |
Monte-1 | 9966 | Italy | 40.28 | 15.65 | (N9H, V28L, A29S, G35A, N51K, S66L, R68G), M256I, P467S |
IP-Vis-0 | 9600 | Spain | 39.85 | −6.04 | E208K, P535Q |
IP-Per-0 | 9879 | Spain | 37.6 | −1.12 | E208K, P535R, P574L |
IP-Vaz-0 | 9593 | Spain | 42.26 | −2.99 | R252H, Y552N |
Ra-0 | 6958 | France | 46 | 3.3 | (N9H, H20Q, V28L, A29S, G35A, N51K, S66L, R68G), A556T |
MNF-Pin-39 | 2016 | United States | 43.5356 | −86.1788 | S570Y |
Accession . | Collection Location . | Mutations . | |||
---|---|---|---|---|---|
Name . | Number . | Country . | Latitude . | Longitude . | . |
Start Codon/Splice Site Mutations | |||||
Vash-1 | 9991 | Georgia | 41.2381 | 46.3728 | Start codon (M1L) |
Jl-3 | 7424 | Czech Republic | 49.2 | 16.6166 | Splice donor at end of exon 5 |
IP-Tau-0 | 9899 | Spain | 42.54 | 0.84 | Splice donor at end of exon 10 |
Amino Acid Substitutions | |||||
Cvi-0 | 6911 | Cape Verde | 15.1111 | −23.6167 | F291L |
IP-Pva-1 | 9888 | Spain | 40.93 | −3.31 | R304Q |
IP-Vas-0 | 9904 | Spain | 40.95 | −3.31 | (N9H, V28L, A29S, G35A, N51K, S66L, R68G), M256I |
Gn2-3 | 9790 | Germany | 48.58 | 9.18 | G394E, N430S |
TueSB30-3 | 9999 | Germany | 48.53 | 9.06 | G394E, N430S |
Ped-0 | 9947 | Spain | 40.74 | −3.9 | (N9H, V28L, A29S, G35A), V403F, A458G |
IP-Ang-0 | 9519 | Spain | 41.94 | 2.64 | (N9H, V28L, A29S, G35A, N51K, S66L, R68G), M256I, K425M |
Amel-1 | 6990 | Netherlands | 53.448 | 5.73 | E452K |
IP-Hue-3 | 9851 | Spain | 42.96 | −6.1 | A458G |
Monte-1 | 9966 | Italy | 40.28 | 15.65 | (N9H, V28L, A29S, G35A, N51K, S66L, R68G), M256I, P467S |
IP-Vis-0 | 9600 | Spain | 39.85 | −6.04 | E208K, P535Q |
IP-Per-0 | 9879 | Spain | 37.6 | −1.12 | E208K, P535R, P574L |
IP-Vaz-0 | 9593 | Spain | 42.26 | −2.99 | R252H, Y552N |
Ra-0 | 6958 | France | 46 | 3.3 | (N9H, H20Q, V28L, A29S, G35A, N51K, S66L, R68G), A556T |
MNF-Pin-39 | 2016 | United States | 43.5356 | −86.1788 | S570Y |
Many of the polymorphisms in GBSS were predicted to cause nonsynonymous amino acid substitutions in the protein. A total of 51 different substitutions was found among the 1135 accessions (Supplemental Dataset S1). We predicted whether each was likely to affect protein function using the PROVEAN tool, which assesses the effects of substitutions based on similarity to other homologs (Choi et al., 2012; Choi and Chan, 2015). Eighteen of the nonsynonymous substitutions occurred in the first 79 amino acids that constitute the chloroplast transit peptide. Transit peptides are variable in sequence, and most substitutions in this region occurred in multiple accessions and were not predicted to be deleterious by PROVEAN. By contrast, most substitutions in the mature protein were rare and found in less than 2% of sequenced accessions. An exception was the Met-256Ile substitution, present in 15% of sequenced accessions and not predicted to be deleterious by PROVEAN. Out of a total of 33 different amino acid substitutions in the mature protein, 14 were predicted to be deleterious by PROVEAN. None were in known catalytic residues or in the binding sites of ADP-Glc and acceptor glucan substrates. A Glu-452Lys substitution (in Amel-1 from Ameland, the Netherlands) was of particular interest, because we showed previously that the Glu-452 residue resides in the coiled coil of GBSS and is necessary for a normal level of interaction between GBSS and PTST1 (Seung et al., 2015). This substitution was detected by the 1001 Genomes Browser but not by the Polymorph browser. Apart from this instance, the SNP data in the two browsers were identical.
To explore how the polymorphisms in GBSS affect amylose synthesis, we selected a set of 18 accessions for further analysis. This set included the three accessions with start codon or splice site mutations and 15 that are predicted to have various amino acid substitutions (including all of the substitutions predicted to be deleterious) and an accession containing only the common Met-256Ile substitution (IP-Vas-0; Table 1). In addition to this set, we also analyzed the reference accession Col-0 and the gbss transfer DNA insertion mutant (in the Col-0 background)—the amylose contents of which were reported previously (Seung et al., 2015).
The genotypes of the selected accessions were confirmed by sequencing the GBSS locus amplified from genomic DNA. All of the polymorphisms reported by the 1001 Genomes Browser were confirmed, including the SNP predicted to cause a Glu-452Lys substitution in Amel-1, which was missed by the Polymorph tool. Several additional polymorphisms in the region encoding the transit peptide were found in some accessions (Supplemental Dataset S1). Their absence from Polymorph may be due to incomplete sequencing data in the pseudogenome assemblies.
The Accessions Carrying Polymorphisms in GBSS Produce Starch with Varying Amylose Content
For analysis of starch composition and content, accessions were grown for 3 weeks in a controlled environment under a 12-h day/12-h night regime. Rosettes of Col-0 and most of the accessions stained dark brown/blue with Lugol’s iodine solution at the end of the day, indicative of the presence of both amylopectin and amylose (Fig. 2A). By contrast, the gbss mutant stained light brown, characteristic of amylose-free starch, and five accessions (Vash-1, IP-Tau-0, Gn2-3, TueSB30-3 and MNF-Pin-39) stained similarly, suggesting that they may also lack amylose.
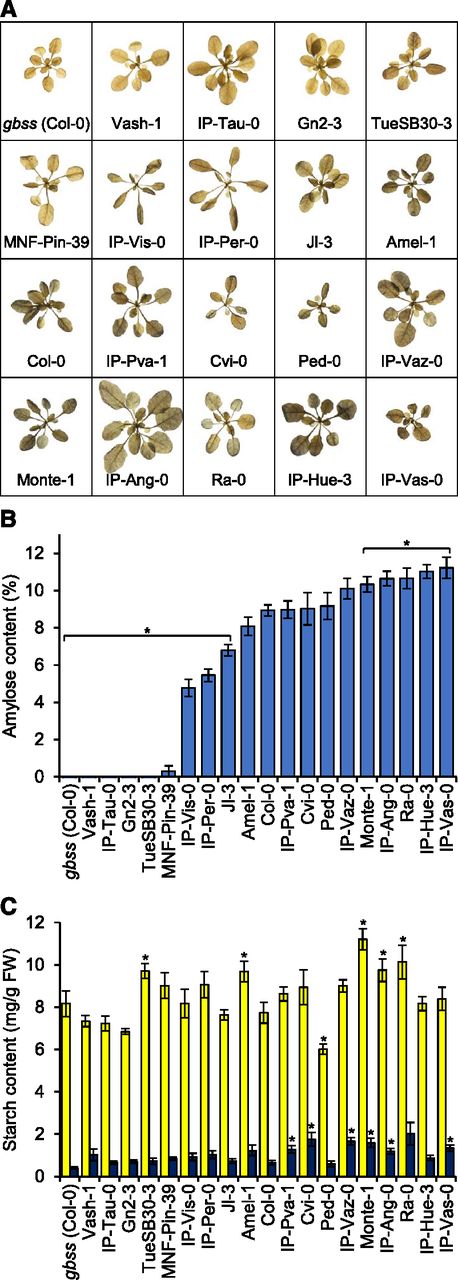
Variation in amylose content among Arabidopsis accessions. A, Iodine-stained rosettes. Three-week-old plants were harvested at the end of day, cleared of chlorophyll, and then stained with Lugol’s iodine solution. B, Amylose content of starch. Amylose was detected using an iodine colorimetry-based method in starch from 3-week-old plants harvested at the end of the day. Values are the mean ± sem of n = 5–6 individual rosettes. Those that are significantly different from the reference accession, Col-0 (two-tailed t test, P < 0.05), are indicated with an asterisk (*). C, Total starch content at the end of day (yellow) and end of night (dark blue). Measurements were made on the same batch of plants as B. Values are the mean ± sem of 5–6 individual rosettes. Values that are significantly different from those of the reference accession, Col-0, at each time point (two-tailed t test, P < 0.05) are indicated with an asterisk (*).
The apparent amylose content of starch was measured using iodine colorimetry (Zeeman et al., 2002a; Hostettler et al., 2011). Consistent with our previous study, starch from Col-0 rosettes contained around 9% (w/w) amylose, and the gbss mutant had no detectable amylose (Seung et al., 2015). The amylose content of starches from the other accessions ranged from 0% to 12% (w/w; Fig. 2B). We divided the accessions into four categories: amylose-free (no detectable amylose), low-amylose (significantly lower amylose than Col-0), mid-amylose (not significantly different from Col-0), and high-amylose (significantly higher amylose than Col-0).
To our knowledge, the amylose-free Arabidopsis accessions are the first examples of wild plants that do not contain amylose. They included Vash-1, IP-Tau-0, Gn2-3, and TueSB30-3, rosettes of which stained light brown with iodine (Fig. 2A). These data suggest that the start codon and splice site mutations in Vash-1 and IP-Tau-0, respectively, cause a complete loss of GBSS function. Gn2-3 and TueSB30-3 (both from southern Germany) carry the same GBSS allele, encoding two amino acid substitutions (Gly-394Glu and Asn-430Ser). The loss of amylose in these accessions suggests that one or both amino acids is essential for GBSS activity in vivo (Fig. 2B). Among the low-amylose accessions, MNF-Pin-39 starch had less than 0.5% (w/w) amylose, consistent with its brown color when stained with iodine, whereas the other accessions had between 5% and 7% (w/w) amylose. Thus, the amylose content of MNF-Pin-39 was closer to the amylose-free accessions than the low-amylose accessions. Starch from the high-amylose accessions had between 10% and 12% (w/w) amylose.
A high-amylose content is typical of starch degradation mutants of Arabidopsis that fail to degrade all of their starch at night (Zeeman et al., 2002a; Seung et al., 2015). We investigated whether there was a relationship between amylose content and the extent of starch turnover among the selected accessions. There was a 4-fold variation in the end-of-night starch content and an almost 2-fold variation in end-of-day starch content (Fig. 2C), but there was no obvious relationship between amylose content and total starch content at either timepoint (Supplemental Fig. S2). Thus, the variation in amylose content among the accessions cannot be attributed to differences in starch turnover.
The Low-Amylose Accessions Have Reduced Amounts of GBSS Protein
To assess the impact of the various polymorphisms on GBSS protein abundance, we purified starch from three accessions selected from each of the amylose-content categories, extracted starch-bound proteins and used immunoblotting with fluorescent antibodies to quantify GBSS protein (Fig. 3A). GBSS abundance on starch was substantially lower in the amylose-free accessions than in the other accessions: Vash-1 had no detectable GBSS protein, and IP-Tau-0 and TueSB30-3 had levels that were 10% to 20% of the Col-0 level. GBSS in these three accessions was also barely detectable in immunoblots of total protein from leaves (Supplemental Fig. S3). Hence, the reduced abundance of GBSS on starch was not due to a failure of the protein to bind to starch. Interestingly, although the Amel-1 GBSS contained a substitution at Glu-452 (Glu-452Lys) shown previously to be important for its interaction with PTST1 (Seung et al., 2015), the GBSS level on Amel-1 starch was only 30% lower than that of Col-0, and its amylose content was comparable to that of Col-0.
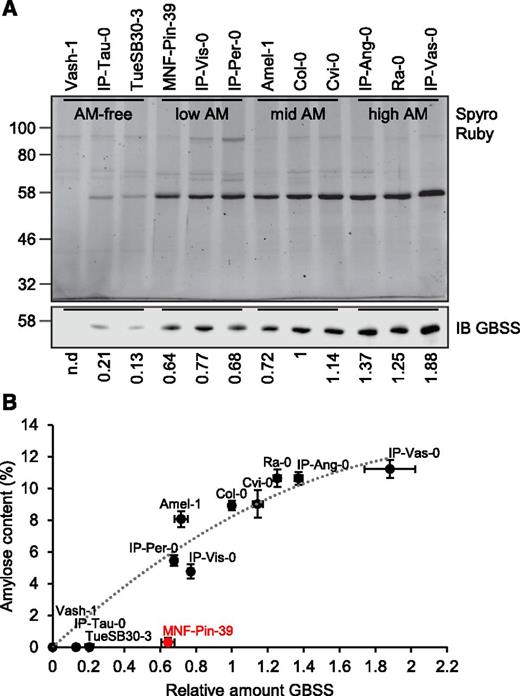
GBSS protein abundance in the different Arabidopsis accessions. A, GBSS abundance on starch in selected amylose-free (AM-free), low-amylose (low AM), mid-amylose (mid AM), and high-amylose (high AM) accessions. Granule-bound proteins extracted by gelatinizing purified starch were separated on a 10% SDS-PAGE gel and stained for total protein using Sypro Ruby (top). Lanes were loaded on an equal starch basis (0.15 mg starch per lane). GBSS was also detected specifically in the extracts by immunoblotting (IB) with the GBSS antibody (bottom; 0.05 mg starch per lane). The quantification of band intensities in the immunoblot relative to the Col-0 band is shown below the blot and is the average from two separate protein extractions. The migration of molecular weight markers (in kilodaltons) is indicated on the left. n.d., not detected. B, Relationship between GBSS abundance on starch and amylose content. Amylose contents from Figure 2B (mean ± sem of n = 5–6 individual rosettes) were plotted against the relative amount of granule-bound GBSS estimated in A (mean of two independent determinations, where the tips of the error bar represent the two individual values). Most data points fit a polynomial trendline, except for MNF-Pin-39 (marked in red).
Overall, GBSS abundance on starch correlated positively with amylose content (Fig. 3B). As observed for other species previously (Tsai, 1974; Flipse et al., 1996; Denyer et al., 2001), the relationship between GBSS abundance and amylose content was nonlinear, and fit a second-order polynomial curve (equation y = −2.2x2 + 10.4x; where y is amylose content and x is relative GBSS abundance; R2 = 0.92). Amylose content plateaued at GBSS levels above that of Ra-0. Thus IP-Vas-0, which had ∼40% more GBSS on starch than the other high-amylose accessions, did not have a higher amylose content than those accessions. One accession in particular deviated from the curve: MNF-Pin-39 had 60% of the GBSS level of Col-0 but made almost no amylose.
MNF-Pin-39 GBSS Has Low Activity
Using recombinant proteins, we tested the effect on GBSS activity in vitro of the amino acid substitutions in the selected amylose-free, low-amylose, and high-amylose accessions. Mature GBSS proteins with an N-terminal His-tag were expressed in Escherichia coli and purified using Ni2+-NTA beads. GBSS activity was measured as the rate of incorporation of 14C Glc from ADP-[14C]Glc into amylopectin. All GBSS variants were active, but the variants from MNF-Pin-39 and IP-Per-0 had significantly lower specific activities than the variants from other accessions (Fig. 4). The low activity of the MNF-Pin-39 variant suggests that the Ser-570Tyr substitution is detrimental to activity and is consistent with the very low amylose content of this accession despite substantial GBSS abundance (Fig. 3). The low activity of the IP-Per-0 variant was unexpected because the accession produces amylose. It is possible that these amino acid substitutions affect catalytic activity on dilute, soluble substrates in vitro but are less important in vivo, where GBSS is located on or inside the granule matrix. From the high-amylose accessions, only the IP-Ang-0 GBSS variant had higher specific activity than the Col-0 GBSS (Fig. 4). This suggests that increased GBSS abundance on starch rather than altered specific activity accounts for high-amylose content in these accessions. Surprisingly, the GBSS of the amylose-free accessions TueSB30-3 and Gn2-3 had almost twice the activity of the Col-0 GBSS. Thus, it appears that the amino acid substitutions in the GBSS variant from these accessions are not detrimental to activity but prevent amylose synthesis in vivo by another mechanism.
![GBSS-specific activity in vitro. Purified recombinant GBSS protein (0.25 µg) was incubated with ADP-[14C]Glc for 10 min with potato amylopectin (50 mg mL−1). After incubation, the amylopectin was precipitated and pelleted. GBSS activity was calculated by measuring the radioactivity incorporated into amylopectin. Values are the mean ± sem of three replicate reactions, and those that are significantly different from Col-0 GBSS (two-tailed t test, P < 0.05) are indicated with an asterisk (*).](https://oup.silverchair-cdn.com/oup/backfile/Content_public/Journal/plphys/182/2/10.1104_pp.19.01062/2/m_plphys_v182_2_870_f4.jpeg?Expires=1747940984&Signature=1nQmCnj3lY5PDGBkmEmWLNAfxI5ggL73eJZiRDu~vVucORGWFFyQRNZlNSQIbOflsO0YJriHLilQM3O8e232-O-ruTrrTiCdrMxDAHEMrPrPTxSr44oTQVgnn3wT7r6bxZWNwBPhLVUu5sdZM68neI2lLIf2LyTn1seb1b8EvCEG7elPrIRKvdPPcfFM5L8--YYFsMP9lLqRMeBrf7d2EOgkQ0Pq7IM~7~unsI48PNvMFzNa0LRXZCdrNoEOrmcipeGirl1CWhE9EI-dCoUu-rK3seIS8ew0y5DsQCZJC~xpfJCmIACMpTKGqYINNdpH4v-Lv6uD44E0NDohBTRY0g__&Key-Pair-Id=APKAIE5G5CRDK6RD3PGA)
GBSS-specific activity in vitro. Purified recombinant GBSS protein (0.25 µg) was incubated with ADP-[14C]Glc for 10 min with potato amylopectin (50 mg mL−1). After incubation, the amylopectin was precipitated and pelleted. GBSS activity was calculated by measuring the radioactivity incorporated into amylopectin. Values are the mean ± sem of three replicate reactions, and those that are significantly different from Col-0 GBSS (two-tailed t test, P < 0.05) are indicated with an asterisk (*).
The TueSB30-3/Gn2-3 GBSS Cannot Interact with PTST1
We tested whether the recombinant GBSS variants had an altered capacity to interact with PTST1. Reduction or loss of PTST1 binding would be expected to reduce the amount of granule-bound GBSS and hence reduce amylose synthesis (Seung et al., 2015). In pull-down assays, most GBSS variants bound PTST1 (from Col-0) to approximately the same extent as the Col-0 GBSS, but the variants from IP-Per-0, Amel-1, and TueSB30-3/Gn2-3 did not (Fig. 5A). The former two showed reduced binding to PTST1 compared to Col-0 GBSS, while no interaction between the TueSB30-3/Gn2-3 GBSS variant and PTST1 was detected. The reduced interaction between PTST1 and the Amel-1 variant GBSS is explained by its Glu-452Lys substitution: we showed previously that Glu-452 lies in the coiled-coil motif and is essential for full binding to PTST1 (Seung et al., 2015). The lack of interaction between PTST1 and the TueSB30-3/Gn2-3 GBSS variant implicates either the Gly-394Glu or the Asn-430Ser substitution in preventing PTST1 binding. Tests with variant proteins that contained one or the other of these substitutions showed that Gly-394 is essential for binding but Asn-430 is not (Fig. 5B). Gly-394 resides on a flexible loop directly adjacent to the coiled-coil region of GBSS (Supplemental Fig. S4). This residue appears to be more important in PTST1 binding than the charged residues within the coiled coil identified previously as the PTST1 interaction site (Seung et al., 2015).
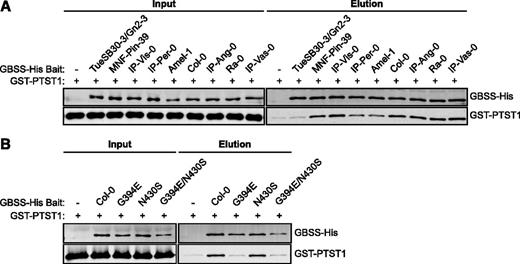
The TueSB30-3/Gn2-3 GBSS cannot interact with PTST1. A, Pull-down assay of interaction between recombinant GBSS and PTST1. The purified tagged proteins, GBSS-His, and GST-PTST1 (0.1 µg each) were coincubated with Ni2+-NTA resin for 1 h (input), and resin-bound proteins were subsequently eluted (elution). The input and elution fractions were analyzed by immunoblotting with anti-His and anti-PTST1 antibodies to detect GBSS-His and GST-PTST1 proteins, respectively. B, The effect of the two amino acid variants in the TueSB30-3/Gn2-3 GBSS on interaction with PTST1 were tested individually. Pull-down assays with purified recombinant proteins carrying the substitutions were performed as in A.
TueSB30-3/Gn2-3 GBSS Can Produce Amylose in the Root Tip
We investigated whether the five accessions that produced amylose-free or low-amylose (< 0.5% [w/w]) starch in leaves are also affected in amylose synthesis in the root tip. Starch accumulates in nonphotosynthetic amyloplasts of columella cells and is thought to function primarily in gravity perception in this tissue rather than in a metabolic role. We stained root tips with iodine for a qualitative analysis of starch composition. As expected, amyloplasts in the columella cells of the Col-0 wild type stained dark blue, while those of the gbss mutant stained brown (Fig. 6). Vash-1 and IP-Tau-0 root tips stained brown like the gbss mutant, suggesting that the strong reduction in GBSS protein in these accessions prevents synthesis of significant amounts of amylose in any tissue. Interestingly, although Gn2-3 and TueSB30-3 produced amylose-free starch in leaves, their root tips stained like those of Col-0, indicating that they contained amylose. Since the TueSB30-3/Gn2-3 GBSS variant cannot interact with PTST1 (Fig. 5), we investigated whether PTST1 is required for amylose accumulation in the root tip (Fig. 6). Root amyloplasts of the ptst1 mutant stained like Col-0, indicating that PTST1 is not required for amylose accumulation in this tissue. These data are consistent with TueSB30-3/Gn2-3 GBSS being catalytically active but unable to interact with PTST1 (Figs. 4 and 5). Thus, this GBSS cannot function in leaves, in which PTST1 is required for amylose synthesis, but can function in cell types in which PTST1 is not required. Amylose was detected in root tips of MNF-Pin-39, as expected from the low but detectable activity of MNF-Pin-39 GBSS and the presence of amylose in leaves of this accession.
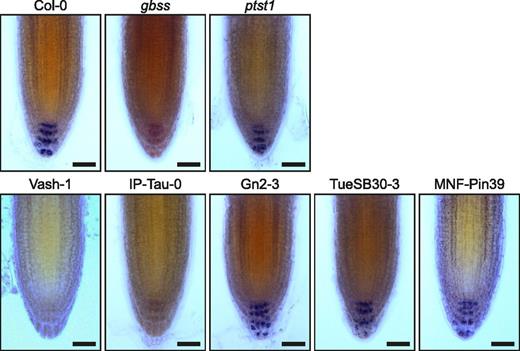
Visualization of starch in Arabidopsis columella cells. Primary root tips of 7-d-old Arabidopsis seedlings were stained with Lugol’s iodine solution and imaged using light microscopy. Bars = 20 µm.
DISCUSSION
Intraspecies Diversity in Amylose Content in Arabidopsis
We found that across the 1135 sequenced Arabidopsis accessions, the GBSS gene contained numerous SNPs. Three SNPs affected splice sites or the start codon, and 51 SNPs were predicted to result in different nonsynonymous amino acid substitutions relative to the GBSS of Col-0—14 of which were predicted to be deleterious to GBSS function (Supplemental Data S1). We selected 18 different accessions that represent this variation and found that leaf starch from four of them contained no detectable amylose, one had a very low amylose content (< 0.5% [w/w] of starch), and a further eight had amylose contents that were statistically significantly different from that of Col-0 starch (Fig. 2). Amylose-free varieties of crops have been cultivated for centuries, but these mutants have been maintained in several crop species by positive selection for the desirable properties of amylose-free starch and flour (Olsen and Purugganan, 2002; Stamp et al., 2016; Zhang et al., 2019). Thus, the discovery of wild accessions of a noncultivated plant species that contain no or very low amylose is surprising.
The existence of accessions with abnormally high or low/zero amylose contents could not have been predicted simply from differences in GBSS abundance and amino acid sequences. For example, the drastic reduction in amylose content in TueSB30-3, Gn2-3, and MNF-Pin-39 results from amino acid substitutions involving residues that were not previously known to be important for GBSS function. Two out of three splice site and start codon mutations prevented GBSS production (Vash-1 and IP-Tau-0), but the third (Jl-3) did not. Given the extent of variation of GBSS sequence and consequent variation in amylose content within Arabidopsis, equivalent variation may well exist in other wild species.
Diversity and Persistence of Natural Loss-of-Function gbss Alleles
Although we have uncovered examples of Arabidopsis accessions that do not produce amylose, most accessions are likely to have a functional GBSS and produce amylose. This, together with the occurrence of amylose in all plants thus far examined, suggests that the possession of amylose must present some selective advantage in most environments over the possession of starch consisting only of amylopectin. At a physical level, the presence of amylose alters the relative widths of the amorphous and crystalline lamellae that form the semicrystalline 9-nm-wide rings of the starch granule matrix (Jenkins and Donald, 1995). No physiological consequences of this structural difference are known. In Arabidopsis, the patterns of starch synthesis and degradation are identical in wild-type and gbss leaves grown in controlled conditions (Seung et al., 2015). The presence/absence of amylose in leaf starch has been claimed to influence the transition from the vegetative to the flowering state in Arabidopsis (Ortiz-Marchena et al., 2014), but we found no obvious relationship between amylose content and flowering time within the accessions with altered amylose contents (Supplemental Fig. S5).
However, the discovery of wild amylose-free plants suggests that the presence of amylose in leaf starch is not necessary for survival under at least some environments and opens an opportunity to study the adaptive significance of amylose synthesis. The amylose-free accessions are widely geographically scattered, suggesting that loss of amylose may not be detrimental in these diverse regions, at least in the short term (Supplemental Fig. S5). Further work examining the prevalence of the loss-of-function gbss alleles in local populations would be required to establish how far these mutations have spread. Interestingly, the same gbss allele was found in TueSB30-3 and Gn2-3, which originate from locations in Germany (Tübingen and Gniebel, respectively) that are only 15 km apart. The rosettes were morphologically different, and genome-wide SNP analysis revealed many SNPs that were present in only one of the two accessions (Supplemental Fig. S6). However, there were large regions on chromosomes 1 and 5 in which the accessions had identical SNPs. The GBSS locus was in such a region, on chromosome 1. Given the close proximity of the collection sites, it is likely that this gbss allele has persisted in the region over the period of time taken for local populations to transfer alleles and/or diverge from each other. Interestingly, other accessions collected in the region (the other “Tue” alleles) do not contain the defective GBSS allele, suggesting that the local population contains a mix of individuals with and without amylose (Supplemental Table S1).
The other three low-amylose/amylose-free accessions (Vash-1, IP-Tau-0, and MNF-Pin-39, from Georgia, Spain, and the United States, respectively) each had a unique gbss allele (Table 1). It seems possible that these mutations arose recently and persist because loss of amylose has little or no impact on fitness in these locations. Further analyses of accessions in these local areas would reveal whether the mutations are confined to a single population or are present in genetically distinct populations.
By contrast to leaves—where there are no reports of naturally amylose-free starches prior to our study—spontaneous mutations in GBSS genes that lead to amylose-free or low-amylose seed starch were selected for in several crops, including rice, sorghum (Sorghum bicolor), millet (Setaria italica), barley, and amaranth (Amaranthus cruentus). These species all possess two isoforms of GBSS, and in most cases, one is confined to vegetative, maternal tissues and the other to the starch-storing endosperm or perisperm of the seed (Cheng et al., 2012; Park et al., 2017). Loss of the endosperm-specific isoform does not affect the amylose content of starch in vegetative tissues. We are not aware of previous reports of natural accessions with amylose-free starch in seeds or other storage tissues in any undomesticated species. In general, the paucity of information about starch composition in populations of wild species precludes any conclusions about the frequency and significance of this phenomenon. Since there is only one GBSS gene in Arabidopsis, the accessions that had disrupted GBSS protein production (Vash-1 and IP-Tau-0) are most likely compromised in amylose synthesis in all organs. Consistent with this, amylose was undetectable in root tips of both accessions (Fig. 6). However, TueSB30-3 and Gn2-3 produced amylose in the root tip (discussed below). Comparing the persistence of these different mutant alleles in wild populations may distinguish the effect on fitness of losing amylose in the whole plant, compared to losing amylose only in the leaves. It is interesting to note that we have not found any accession that has physically lost the GBSS locus. Large deletions that eliminate whole genes are more likely to also affect neighboring loci and are thus likely to be more detrimental to fitness.
GBSS Activity and Abundance on Starch Underpins Variation in Amylose Content
We found three independent mechanisms that result in low or zero amylose starch in Arabidopsis: (1) mutations that abolish production of GBSS protein (e.g. Vash-1 and IP-Tau-0; Figs. 1 and 3), (2) mutations that prevent enzymatic activity (e.g. MNF-Pin-39; Fig. 4), and (3) mutations that prevent the targeting of GBSS to the starch granule (e.g. Gn2-3 and TueSB30-3; Fig. 5).
Several factors have been proposed to influence the amylose content of starch in wild-type Arabidopsis leaves, in particular the size and persistence of starch granules and the amount of GBSS on starch (Zeeman et al., 2002a, 2002b; Smith et al., 2004; Seung et al., 2015). Because amylose synthesis occurs within the granule matrix formed from amylopectin, amylose accumulation is potentially limited by the extent of amylopectin synthesis in the light. This idea may explain the plateauing of amylose content as GBSS abundance increases (Fig. 3) and is consistent with the observation that starch degradation mutants with high starch contents at the end of the night also have elevated amylose contents. Presumably their larger, more persistent granules allow more amylose to be accommodated (Zeeman et al., 2002a). However, granule persistence and volume were not factors that determined the variation of amylose content between accessions. There was no correlation between amylose content and the starch content at the end of day or the end of night (Fig. 2, Supplemental Fig. S2).
The range of amylose contents we observed was strongly related to the amount of GBSS on starch granules rather than GBSS-specific activity (Fig. 3B). As expected from studies in other species (Tsai, 1974; Flipse et al., 1996; Denyer et al., 2001; Sestili et al., 2012), the relationship between GBSS abundance and amylose content fit a polynomial curve that plateaued at high GBSS abundance, such that the accessions with the highest levels of GBSS per unit starch (IP-Ang-0 and IP-Vas-0) had only modest elevations of amylose content relative to Col-0. Presumably, factors such as granule volume and the availability of ADP-Glc increasingly limit amylose synthesis as GBSS abundance rises (Fig. 3). An outlier on the curve was MNF-Pin-39, which had only 35% less GBSS than Col-0 but produced very little amylose. This phenomenon could be explained by the low specific activity of the recombinant protein in vitro (Fig. 4). The amino acid change in the GBSS variant of MNF-Pin-39 is Ser-570Tyr. Ser-570 is highly conserved among putative GBSS orthologs but is not close to the catalytic residues (Supplemental Fig. S4). It is possible that the Ser-570Tyr substitution causes a change in the structure or flexibility of the protein that indirectly affects catalysis.
The capacity of GBSS to interact with PTST1 is an important determinant of its abundance in starch. Three GBSS variants (from TueSB30-3/Gn2-3, Amel-1, and IP-Per-0) had reduced or no capacity to bind PTST1, and accessions carrying these variants had lower GBSS abundance on starch relative to Col-0 (Fig. 5). For TueSB30-3/Gn2-3, the reduced/zero capacity for binding to PTST1 is likely to be the major or sole cause of the low GBSS abundance on starch and the absence of amylose, since the variant recombinant GBSS protein had a high specific activity (Fig. 4). Interestingly, we found that PTST1 is not required for amylose biosynthesis in root tips, and the TueSB30-3 and Gn2-3 accessions were also not amylose free in the roots. This may reflect differences in starch turnover between tissues. While there is some starch turnover in Arabidopsis roots (Vitha et al., 2007), it is unlikely that the starch is degraded completely during the night as in leaves, and this may provide more time for amylose to accumulate. Similarly, it is known that some amylose can accumulate in the absence of PTST1 in leaves of mutants that do not fully degrade their starch at night and also in storage roots of cassava, which accumulate starch over a much longer period than in leaves (Seung et al., 2015; Bull et al., 2018). For Amel-1 and IP-Per-0, the variant proteins had similarly reduced capacities for binding PTST1, and GBSS abundance was similarly reduced in both accessions relative to Col-0 (Figs. 3 and 5). However, the situation in IP-Per-0 may be more complex, as it had ∼60% of the amylose content of Col-0 despite very low specific activity (Fig. 4). In this case, the deleterious consequences of an amino acid substitution for protein performance in vitro do not reflect its capacity to synthesize amylose in vivo.
Natural Accessions as a Tool to Discover Factors Regulating Amylose Synthesis
It is entirely possible that variation in genes other than GBSS may influence the amylose content of leaves in wild plants. An obvious candidate is PTST1, and sequencing data revealed several SNPs predicted to generate amino acid substitutions, but we did not find any premature stop codon, start codon, or splice site mutations in PTST1 using the Polymorph tool. No SNPs were predicted to be deleterious by Provean (Supplemental Dataset S2), but it remains possible that some may affect PTST1 function. The sequence of PTST1 was identical to that of Col-0 in the accessions in which GBSS protein had a reduced interaction with PTST1 protein (TueSB30-3, Gn2-3, and Amel-1).
We suggest that a survey of leaf amylose content across the sequenced accessions might uncover genes in addition to GBSS and PTST1 that influence amylose accumulation. In addition, it may reveal polymorphisms outside the coding sequence of these genes that influence amylose content, and the effect on amylose content of amino acid substitutions that were not predicted as deleterious by the PROVEAN program. Out of the 14 amino acid substitutions that were predicted to be deleterious by PROVEAN, only two led to amylose-free or low-amylose (< 0.5% [w/w]) phenotypes. It is therefore possible that some substitutions predicted to be nondeleterious by the program may in fact lead to nonfunctional protein. Overall, a broader, unbiased survey of amylose content among different accessions may lead to a greater understanding of GBSS function, while shedding light on the role and adaptive significance of the amylose polymer.
MATERIALS AND METHODS
Plant Materials and Growth Conditions
Arabidopsis (Arabidopsis thaliana) plants were grown on soil in controlled environment chambers with 12-h light, 12-h dark cycles at a constant temperature of 20°C and relative humidity of 65%. For staining starch in root tips, seedlings were grown under the same conditions but on plates containing half strength Murashige and Skoog salts stabilized with 0.8% (w/v) agar.
Arabidopsis accessions from the 1001 Genomes Project collection were ordered from the Nottingham Arabidopsis Stock Centre (NASC). The gbss transfer DNA knockout mutant, GABI_914G01, was described previously (Seung et al., 2015).
Sequencing and SNP Analysis
To identify SNPs in GBSS in different Arabidopsis accessions, we used the sequencing data generated by the 1001 Genomes Consortium (2016). SNPs were identified in the GBSS locus (At1g32900) using the web-based Polymorph tool (https://tools.1001genomes.org/polymorph/index.html) and the Arabidopsis 1001 Genomes Browser (http://signal.salk.edu/atg1001/3.0/gebrowser.php). The impact of each SNP on amino acid sequence was predicted using the PARSESNP tool (Taylor and Greene, 2003; http://blocks.fhcrc.org/∼proweb/input/; note that as of October 2019, this webtool is no longer available). The impact of the missense mutations on protein function was predicted using PROVEAN Protein tool (Choi and Chan, 2015; http://provean.jcvi.org/seq_submit.php) using the NCBI nonredundant protein database (September 2012) as the search database. Deleterious mutations were predicted using the default cutoff score of −2.5.
Primers for amplification or sequencing reactions are provided in Supplemental Table S1. The GBSS locus was amplified from genomic DNA purified using a high-fidelity polymerase (either Phusion or Q5, New England Biolabs). The PCR product was cloned into the blunt end vector pJET1.2, and sequenced by Sanger sequencing.
For cDNA sequencing, total RNA was isolated from leaves 2 h after dawn (the time of peak GBSS expression Smith et al., 2004]). Total cDNA was synthesized from (2 µg RNA) using the GoScript Reverse Transcriptase kit (Promega) with oligo dT primers. GBSS cDNA was amplified from total cDNA using the Q5 polymerase.
For the genome-wide comparison of SNPs presented in Supplemental Figure S6, whole-genome sequencing data for Gn2-3 (sample 9790, SRR1946353) and TueSB30-3 (sample 9999, SRR1946535) were downloaded from the NCBI short read archive. Reads from both accessions were mapped to the reference sequence (Col-0) using bwa (version 0.7.12) and samtools (version 1.7) software with default parameters. The sorted mapping files were converted to mpileup format using samtools. The mpileup files were screened for SNPs using a custom Java program. SNPs were called in either one of the two accessions if the allele was supported by at least 90% of the reads. The same SNP was called in the remaining accession if it was present in > 70% of the reads. The genome was scanned using 50-kb windows, and the percentage of common SNPs (present in both accessions) relative to the total SNPs was calculated and plotted using R. Only regions that had read coverage ≥ 5 in both accessions were included in the calculation. Details of program calls and scripts are available at https://github.com/steuernb/starch-arabidopsis-ecotilling.
Quantification of Starch and Amylose Content and Iodine Staining
Starch was assayed according to a previously described method (Smith and Zeeman, 2006). In brief, rosettes (3 weeks old) were harvested and immediately frozen in liquid N2. The frozen rosettes were pulverized in a ball mill and extracted with 0.7 m perchloric acid. The insoluble fraction was sedimented at 5000g for 5 min, washed once in double-distilled water, three times in 80% (v/v) aqueous ethanol, and resuspended in a known volume of double-distilled water. Starch in the insoluble fraction was gelatinized for 15 min at 95°C and digested to Glc using α-amylase (Megazyme E-BSTAA) and amyloglucosidase (Roche). Glc was assayed using the hexokinase/Glc-6-phosphate dehydrogenase method (Roche), and starch content was calculated in Glc equivalents.
Amylose was assayed with a modification of the iodine colorimetry method (Zeeman et al., 2002a; Hostettler et al., 2011). First, starch was quantified as described above. A sample of the insoluble fraction (prior to gelatinization) that contained 50 µg of starch was adjusted to 350 µL with water, gelatinized for 10 min at 95°C, then filtered through a 20-µm nylon mesh. The filtrate was mixed with Lugol’s solution (KI/I2 solution; Sigma-Aldrich L6146) in a 1:9 ratio (filtrate:Lugol’s solution). The absorption spectra between 500 and 750 nm was recorded, and amylose content was quantified using a previously described formula (Zeeman et al., 2002a).
For iodine staining of leaves, rosettes harvested at the end of the day were decolorized for 12 to 24 h in 80% (v/v) aqueous ethanol at 20°C to 25°C, washed in water, and stained for 30 min in undiluted Lugol’s solution. Rosettes were destained in water for a period sufficient to reveal color differences between Col-0 and gbss mutant leaves.
Starch Purification
Between 60 and 120 4-week-old rosettes harvested at the end of day were pooled for each purification, conducted as previously described (Seung et al., 2015).
Protein Extractions, SDS-PAGE, and Immunoblotting
For total protein, three to five individual leaves harvested at the end of the day were homogenized in 40 mm Tris-HCl, pH 6.8, 5 mm MgCl2, 2% (w/v) SDS, and Complete protease inhibitor cocktail (Roche). The homogenate was heated at 95°C for 10 min, then centrifuged at 20,000g for 10 min. Protein concentration in the supernatant was quantified (Pierce BCA assay; Thermo Scientific) before adding SDS-PAGE loading buffer.
For granule-bound protein, purified starch granules were suspended in SDS-PAGE loading buffer at 10 mg mL−1. Starch was gelatinized by heating at 95°C for 10 min, then the gelatinized starch was removed by centrifugation at 20,000g for 1 h.
For total protein staining, we used Sypro Ruby Protein Gel Stain (Thermo Scientific) according to the manufacturer’s instructions. For immunoblotting, we used the following primary antibodies: anti-GBSS (Seung et al., 2015) at 1:1000 dilution, anti-GST-PTST1 (Seung et al., 2015) at 1:5000 dilution, anti-His (Abcam ab18184 at 1:3000 dilution), and anti-actin (Sigma Aldrich A0480 at 1:10,000 dilution). Bands were detected using infrared fluorescence (LI-COR Odyssey) using the IRDye 800CW-donkey-anti-rabbit and 680RD-donkey-anti-mouse fluorescent secondary antibodies (LI-COR).
Purification of Recombinant Proteins and Pull-Down Assay
For cloning the GBSS-cTP:pProExHTb vector, the GBSS coding sequence without the region encoding the chloroplast transit peptide (the first 79 amino acids) was amplified from vectors described previously (Seung et al., 2015), and inserted into the pProExHTb (Invitrogen) vector using the EcoRI and XhoI sites, in frame with the sequence encoding the N-terminal His-tag. The GBSS amino acid variants were introduced into the vector using the Quikchange Lightning (Agilent) or Q5 (New England Biolabs) site-directed mutagenesis kits. Primers used for cloning and mutagenesis are in Supplemental Table S1. Escherichia coli BL21 ∆glgAP cells (lacking the endogenous glycogen synthase [Szydlowski et al., 2009]) carrying the GBSS-cTP:pProExHTb plasmid were cultured in liquid lysogeny broth media at 37°C until OD600 = 0.5 to 0.6. Protein expression was induced with 1 mm β-d-1-thiogalactopyranoside for 12 to 14 h at 18.5°C. Cells were pelleted, and resuspended in 50 mm Tris-HCl, pH 7.5, 300 mm NaCl, 30 mm imidazole, 1 mg mL−1 lysozyme. Cells were incubated for 30 min at 4°C then sonicated for 1 min. The lysate was cleared by centrifugation at 20,000g for 10 min, then the supernatant was incubated with Ni2+-NTA-agarose beads (Qiagen) for 1 h at 4°C. The resin was washed five times with 5 volumes of 50 mm Tris-HCl, pH 7.5, 300 mm NaCl, 30 mm imidazole, 0.5% (w/v) Triton X-100, then five times with 5 volumes of the same medium without Triton X-100. The resin was eluted with 3 volumes of 50 mm Tris-HCl, pH 7.5, 300 mm NaCl, 100 mm imidazole, then 3 volumes of 50 mm Tris-HCl, pH 7.5, 300 mm NaCl, 250 mm imidazole. Fractions containing the His-GBSS protein (detected by Bradford assay) were combined (usually the first five fractions), concentrated to 0.5 mL with an Amicon Ultra 10,000 MWCO centrifugal filter (Millipore), and was buffer-exchanged into 50 mm Tris-HCl, pH 7.5, 2 mm dithiothreitol, 10% (v/v) glycerol using a NAP-5 desalting column (GE Healthcare) prior to storage at −80°C.
Procedures for GST-PTST1 purification with Glutathione-Sepharose 4B and the pull-down assay with His-GBSS and Ni2+-NTA-agarose beads (Qiagen) were described previously (Seung et al., 2015).
GBSS Activity Assay
GBSS activity was assayed as previously described (Denyer and Smith, 1992), with the following modifications: purified recombinant GBSS protein (0.25 µg) was incubated with 250 µL 50 mg mL−1 potato amylopectin (Sigma) in 100 mm Bicine-KOH, pH 8, 25 mm potassium acetate, 2 mm MgCl2, 0.05% (w/v) bovine serum albumin, and 5 mm dithiothreitol for 30 min, then the assay was started with 5 µL of 50 µm ADP-[14C]Glc (American Radiolabeled Chemicals; ARC 3297; 11.1 GBq; 300 mCi mmol−1) to give a final concentration of 1 µm in an assay volume of 250 µL. After a 10-min incubation at 22°C, the reaction was stopped with 1 mL of 75% (v/v) methanol/1% (w/v) KCl. Precipitated amylopectin was washed once in 1 mL of 75% (v/v) methanol/1% (w/v) KCl prior to scintillation counting.
Accession Numbers
The Arabidopsis GBSS locus is At1g32900. The Arabidopsis PTST1 locus is At5g39790.
Supplemental Data
The following supplemental materials are available:
Supplemental Figure S1. Effect of GBSS splice site mutations in IP-Tau-0 and Jl-3.
Supplemental Figure S2. Relationship between starch content and amylose content.
Supplemental Figure S3. GBSS protein abundance in total protein extracts of Arabidopsis leaves.
Supplemental Figure S4. Location of Gly-394, Glu-452, and Ser-570 on the Arabidopsis GBSS homology model.
Supplemental Figure S5. Collection locations of the selected accessions.
Supplemental Figure S6. TueSB30-3 and Gn2-3 are genetically distinct accessions.
Supplemental Table S1. Oligonucleotides used in this study.
Supplemental Dataset S1. Variation in GBSS amino acid sequence among 1135 Arabidopsis accessions.
Supplemental Dataset S2. Variation in PTST1 amino acid sequence among 1135 Arabidopsis accessions.
Supplemental Dataset S3. GBSS cDNA sequences from Col-0, IP-Tau-0, and Jl-3.
ACKNOWLEDGMENTS
The authors thank the Horticultural Services department at the John Innes Centre for their assistance in preparing plant material.
LITERATURE CITED
Author notes
This work was funded through a Biotechnology and Biological Sciences Research Council (BBSRC, UK) Future Leader Fellowship BB/P010814/1 (to D.S.), a John Innes Foundation Chris J. Leaver Fellowship (to D.S.), a Genetics Society Summer Studentship (to A.E.-P. and A.M.S.), and a BBSRC Institute Strategic Programme grant BB/P016855/1 awarded to the John Innes Centre.
Articles can be viewed without a subscription.
Senior author.
The author responsible for distribution of materials integral to the findings presented in this article in accordance with the policy described in the Instructions for Authors (www.plantphysiol.org) is: David Seung ([email protected]).
D.S. conceived the study and designed the experiments; D.S. and A.E.-P. conducted the experiments; B.S. designed and conducted the bioinformatics analyses; all authors analyzed data; D.S. and A.M.S. wrote the article with contributions from all authors. D.S. agrees to serve as the author responsible for contact and ensures communication.