-
PDF
- Split View
-
Views
-
Cite
Cite
Priyanka Paul, Sanjay Kumar Singh, Barunava Patra, Xiaoyu Liu, Sitakanta Pattanaik, Ling Yuan, Mutually Regulated AP2/ERF Gene Clusters Modulate Biosynthesis of Specialized Metabolites in Plants , Plant Physiology, Volume 182, Issue 2, February 2020, Pages 840–856, https://doi.org/10.1104/pp.19.00772
- Share Icon Share
Abstract
APETALA2/ETHYLENE RESPONSE FACTOR (AP2/ERF) gene clusters regulate the biosynthesis of diverse specialized metabolites, including steroidal glycoalkaloids in tomato (Solanum lycopersicum) and potato (Solanum tuberosum), nicotine in tobacco (Nicotiana tabacum), and pharmaceutically valuable terpenoid indole alkaloids in Madagascar periwinkle (Catharanthus roseus). However, the regulatory relationships between individual AP2/ERF genes within the cluster remain unexplored. We uncovered intracluster regulation of the C. roseus AP2/ERF regulatory circuit, which consists of ORCA3, ORCA4, and ORCA5. ORCA3 and ORCA5 activate ORCA4 by directly binding to a GC-rich motif in the ORCA4 promoter. ORCA5 regulates its own expression through a positive autoregulatory loop and indirectly activates ORCA3. In determining the functional conservation of AP2/ERF clusters in other plant species, we found that GC-rich motifs are present in the promoters of analogous AP2/ERF clusters in tobacco, tomato, and potato. Intracluster regulation is evident within the tobacco NICOTINE2 (NIC2) ERF cluster. Moreover, overexpression of ORCA5 in tobacco and of NIC2 ERF189 in C. roseus hairy roots activates nicotine and terpenoid indole alkaloid pathway genes, respectively, suggesting that the AP2/ERFs are functionally equivalent and are likely to be interchangeable. Elucidation of the intracluster and mutual regulation of transcription factor gene clusters advances our understanding of the underlying molecular mechanism governing regulatory gene clusters in plants.
Plants produce a vast array of bioactive specialized metabolites in response to various biotic and abiotic stresses. Many specialized metabolites with nutritional and medicinal values are beneficial to animals and humans. While significant progress has been made in discovering the genes encoding key enzymes in the biosynthesis of specialized metabolites, molecular regulatory mechanisms controlling the metabolic pathways are insufficiently understood. Biosynthesis of specialized metabolites is primarily regulated at the transcriptional level (Colinas and Goossens, 2018). The APETALA2/ETHYLENE RESPONSE FACTOR (AP2/ERF) family transcription factors (TFs) have emerged as key regulators of specialized metabolite biosynthesis, including nicotine in tobacco (Nicotiana tabacum; Shoji et al., 2010; De Boer et al., 2011), terpenoid indole alkaloids (TIAs) in Madagascar periwinkle (Catharanthus roseus; van der Fits and Memelink, 2000; Paul et al., 2017) and Ophiorrhiza pumila (Udomsom et al., 2016), artemisinin in Artemisia annua (Yu et al., 2012; Lu et al., 2013), and steroidal glycoalkaloids (SGAs) in tomato (Solanum lycopersicum) and potato (Solanum tuberosum; Cárdenas et al., 2016; Thagun et al., 2016; Nakayasu et al., 2018). AP2/ERFs are subdivided into 12 phylogenetic groups (Nakano et al., 2006). Several group IX AP2/ERFs form physically linked gene clusters that regulate the biosynthesis of specialized metabolites. TF gene clusters have been characterized in a limited number of plant species, including tobacco (Shoji et al., 2010; Kajikawa et al., 2017), tomato (Cárdenas et al., 2016; Thagun et al., 2016; Nakayasu et al., 2018), potato (Cárdenas et al., 2016), and C. roseus (Paul et al., 2017). The tobacco NICOTINE2 (NIC2) locus comprises at least 10 AP2/ERFs that are homologous to the C. roseus ORCAs. Not all NIC2 ERFs are equally effective in regulating nicotine biosynthesis; ERF189 and ERF221/ORC1 play major roles in nicotine biosynthesis (Shoji et al., 2010; De Boer et al., 2011). The AP2/ERF gene clusters in tomato and potato comprise five and eight ERFs, respectively. GLYCOALKALOID METABOLISM9 (GAME9)/JASMONATE-RESPONSIVE ERF4 (JRE4), a member of the AP2/ERF gene clusters in tomato and potato, is key to the biosynthesis of SGAs and the upstream isoprenoids. Knockdown, knockout, or overexpression of the GAME9 genes in tomato and potato affects the SGA pathway gene expression and SGA production (Cárdenas et al., 2016; Thagun et al., 2016; Nakayasu et al., 2018). In C. roseus, the ORCA cluster consists of at least three AP2/ERFs, ORCA3, ORCA4, and ORCA5, of which ORCA3 and ORCA4 are known to regulate the biosynthesis of the pharmaceutically valuable TIAs (Fig. 1A; van der Fits and Memelink, 2000; Paul et al., 2017).

Expression of ORCA3, ORCA4, and ORCA5 in response to JA and ACC. A, Simplified diagram of the TIA biosynthetic pathway in C. roseus. TIA pathway genes studied in this work are highlighted in blue, and genes regulated by ORCAs and CrMYC2a (van der Fits and Memelink, 2000; Paul et al., 2017; Schweizer et al., 2018; this study) are indicated by circles and triangles, respectively. B, Ten-day-old C. roseus seedlings were treated with 100 µm MeJA (JA) and/or 50 μm ACC for 2 h, and gene expression in whole seedling was measured by RT-qPCR. Mock-treated seedlings were used as controls (CN). C, Measurement of ajmalicine, catharanthine, and tabersonine in JA-, ACC-, and JA+ACC-treated C. roseus seedlings. Alkaloids were extracted and analyzed using liquid chromatography-tandem mass spectrometry. The levels of alkaloids were estimated based on peak areas compared with standards. Data represent means ± sd of three biological samples each with 15 to 17 seedlings. Different letters denote statistical differences as assessed by one-way ANOVA and Tukey’s honestly significant difference (HSD) test, P < 0.05. ASα, Anthranilate synthase; CPR, cytochrome P450 reductase; DW, dry weight; G10H, geraniol 10-hydroxylase; HL1/2/3/4, hydrolase 1/2/3/4; IS, iridoid synthase; LAMT, loganic acid methyltransferase; MAT, minovincine 19-O-acetyltransferase; SGD, strictosidine β-glucosidase; SLS, secologanin synthase; STR, strictosidine synthase; T19H, tabersonine 19-hydroxylase; TAT, tabersonine derivative 19-O-acetyltransferase; TEX1/TEX2, tabersonine 6,7-epoxidase isoforms 1 and 2; V19H, vincadifformine 19-hydroxylase.
In addition to the group IX AP2/ERFs, TF gene clusters have been identified in the group III AP2/ERFs, C-repeat Binding Factors (CBFs; Gilmour et al., 1998; Zhang et al., 2004), Auxin Response Factors (ARFs; Hagen and Guilfoyle, 2002), R2R3 MYBs (Zhang et al., 2000, 2019), and basic helix-loop-helix (bHLH) factors (Sánchez-Pérez et al., 2019). TF gene clusters likely originated from tandem gene duplication events (Shoji et al., 2010; Kellner et al., 2015). Unlike the operon-like, nonhomologous metabolic gene clusters (Boycheva et al., 2014; Nützmann and Osbourn, 2014; Nützmann et al., 2016), TF gene clusters encode homologous TFs with overlapping or unique functions. It has been suggested that gene duplication offers the opportunity for mutual regulation among the duplicated genes (Shoji et al., 2010); however, mutual regulatory relationships among the members of any TF cluster remained unconfirmed. Furthermore, the ORCA, NIC2, and GAME9/JRE locus ERFs are phylogenetically related and commonly respond to the phytohormone jasmonic acid (JA), suggesting the evolution of similar regulatory mechanisms in diverse metabolic pathways (Shoji et al., 2010; Thagun et al., 2016). Question thus arose as to whether AP2/ERFs from different clusters are functionally equivalent and interchangeable. Elucidation of the mutual regulatory relationship among the ERF gene clusters implies an evolutionarily conserved molecular mechanism that controls the biosynthesis of functionally and structurally diverse specialized metabolites.
In this study, we discovered a regulatory relationship among the members of the ORCA cluster. The direct activation of ORCA4 by ORCA3 and ORCA5, as well as self-regulation of ORCA5, highlight the presence of feed-forward and autoregulatory loops in the ORCA cluster. We also demonstrated the intracluster regulation among the tobacco NIC2 ERFs. Moreover, ORCA5 overexpression in tobacco hairy roots up-regulated nicotine biosynthetic genes and nicotine accumulation, and reciprocal overexpression of NIC2 ERF189 in C. roseus hairy roots induced the TIA biosynthetic genes, suggesting that the ORCAs and NIC2 ERFs are functionally equivalent and are likely interchangeable.
RESULTS
Phylogenetic Analysis Positions ORCAs, GAME9, and NIC2 ERFs in the Same Clade
AP2/ERFs are divided into 12 groups based on domain structure and other conserved motifs. The group IX AP2/ERFs are involved in phytohormone signaling and defense response (Nakano et al., 2006). Phylogenetic analysis of group IX ERFs from tomato, tobacco, potato, and C. roseus showed that ORCAs are grouped together with NIC2 and GAME9 ERFs, which are involved in nicotine and SGA biosynthesis in tobacco and tomato, respectively (Shoji et al., 2010; Cárdenas et al., 2016; Supplemental Fig. S1). Interestingly, this clade does not include ERFs from Arabidopsis (Arabidopsis thaliana), suggesting that the ERFs in this clade are possibly evolved for the biosynthesis of structurally complex specialized metabolites.
ORCA Gene Cluster Is Differentially Induced by Methyl Jasmonate and Ethylene
Methyl jasmonate (MeJA) is a key elicitor of biosynthesis of a number of specialized metabolites, including nicotine (Shoji et al., 2000), β-thujaplicin (Zhao et al., 2004), artemisinin (Shen et al., 2016), taxol (Mirjalili and Linden, 1996), and SGAs (Thagun et al., 2016; Nakayasu et al., 2018). Ethylene (ET) acts synergistically with MeJA to promote the biosynthesis of taxol in Taxus cuspidate (Mirjalili and Linden, 1996), β-thujaplicin in Cupressus lusitanica (Zhao et al., 2004), and hydroxycinnamic acid amides in Arabidopsis (Li et al., 2018), while ET attenuates the effects of MeJA on nicotine and SGA biosynthetic pathway gene expression in Nicotiana species (tobacco and Nicotiana attenuata) and tomato, respectively (Shoji et al., 2000, 2010; Winz and Baldwin, 2001; Nakayasu et al., 2018). In C. roseus, MeJA induces the expression of ORCA3, ORCA4, and ORCA5 as well as their targets (van der Fits and Memelink, 2000; Paul et al., 2017). To determine the effects of ET alone or in combination with MeJA on ORCA gene expression, C. roseus seedlings were treated with MeJA, 1-aminocyclopropane-1-carboxylic acid (ACC), or both for 2 h, and transcript accumulation was measured by reverse transcription quantitative PCR (RT-qPCR). Expression of ORCA5 was induced 9.5-fold by MeJA but remained unaffected by ACC; however, MeJA-induced expression of ORCA5 was attenuated in the presence of ACC, reduced to 7.5-fold. Expression of ORCA4 and ORCA3 was induced 7- and 12-fold, respectively, by MeJA and reduced to 0.2- to 0.3-fold by ACC (Fig. 1B). Similar to ORCA5, MeJA-responsive expression of ORCA4 and ORCA3 was reduced to 2.5- and 7-fold, respectively, in the presence of ACC. Expression of STR and TDC, two key targets of ORCAs, and CrMYC2a were induced 4-, 12-, and 5.7-fold, respectively, by MeJA treatment. The MeJA-induced expression was reduced to 2- to 4-fold in the presence of ACC (Fig. 1B). In addition, we measured the TIA contents in seedlings treated with MeJA and ACC either alone or in combination. MeJA induced, whereas ACC repressed, the accumulation of tabersonine and ajmalicine. Moreover, MeJA-induced accumulation of tabersonine, but not of ajmalicine, was attenuated by ACC. Accumulation of catharanthine was reduced in MeJA or ACC-treated seedlings (Fig. 1C).
ORCA5 Is a Nucleus-Localized Transcriptional Activator
To determine the transactivation activity, ORCA3, ORCA4, or ORCA5, fused to the GAL4-DNA-binding domain, was coelectroporated into tobacco protoplasts with a luciferase reporter driven by a minimal cauliflower mosaic virus (CaMV) 35S promoter with GAL4-responsive elements as described previously (Paul et al., 2017). Transactivation activities of ORCA3, ORCA4, and ORCA5 were 6.5-, 6.6-, and 12-fold, respectively, higher than the reporter-only control (Supplemental Fig. S2A). The significant inductions of reporter activity in plant cells suggest that three ORCAs are transcriptional activators. To determine the subcellular localization, ORCA5 coding sequence was fused in frame to the enhanced GFP (eGFP) and expressed in tobacco protoplasts. Compared with the protoplasts expressing the eGFP control, in which GFP was detected throughout the cell, the ORCA5-eGFP fusion protein was localized to the nucleus (Supplemental Fig. S2B), consistent with its putative function as a TF.
ORCA4 and ORCA5 Bind to the JRE in the STR Promoter
We have shown that ORCA4 and ORCA5 activate the promoters of key TIA pathway genes, including STR, TDC, and CPR in tobacco cells (Paul et al., 2017). A previous study has shown that ORCA3 binds to the JRE in the STR promoter (van der Fits and Memelink, 2001). To determine whether ORCA4 and ORCA5 also bind the same JRE in the STR promoter, we performed an electrophoretic mobility shift assay (EMSA). We purified the recombinant GST-tagged ORCA3, ORCA4, and ORCA5 (GST-ORCA3/4/5) and CrMYC2a (GST-CrMYC2a) proteins from Escherichia coli using GST affinity chromatography as described previously (Paul et al., 2017; Patra et al., 2018; Fig. 2A; Supplemental Fig. S3A). CrMYC2a, which binds the T/G-box motif, was used as a negative control. The purified ORCA3, ORCA4, ORCA5, or CrMYC2a protein was incubated with a 5′ biotin-labeled probe covering the JRE of the STR promoter. Similar to ORCA3, ORCA4 and ORCA5 also bind to the JRE, resulting in a mobility shift (Fig. 2B). The binding of GST-tagged ORCA5 to the JRE of the STR promoter was further confirmed by competition using 10× and 100× excess of the unlabeled (cold) probe. The intensity of the signal decreased gradually with the increase of the concentration of cold probe (Supplemental Fig. S3B). As shown in Figure 2B, the unlabeled probe, 1,000-fold in excess, outcompeted the labeled probe and abolished the signal, suggesting that the shifted band was indeed the ORCA5-JRE complex. We thus used 1,000× excess of the cold probe for the competition experiments with ORCA3 and ORCA4, and, similar to ORCA5, the cold probe completely abolished the signals on the gel, indicating that the shifted band was indeed the ORCA3/ORCA4-JRE complex (Fig. 2B). We did not detect any signal for CrMYC2a, suggesting that CrMYC2a does not bind to JRE in the STR promoter (Supplemental Fig. S3B).
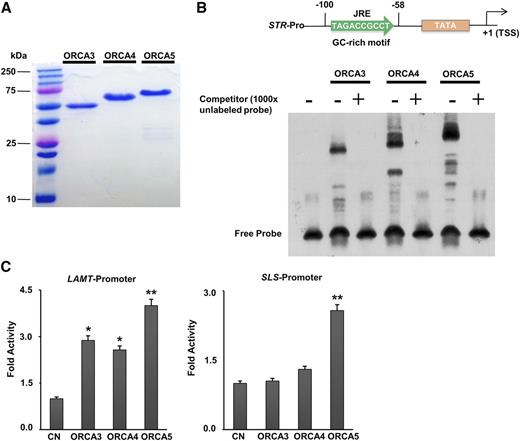
ORCA binding to the GC-rich motif in the STR promoter and differential activation of TIA pathway gene promoters. A, ORCA3, ORCA4, and ORCA5 were expressed in E. coli, and the recombinant proteins were purified to homogeneity as demonstrated by SDS-PAGE. B, Binding of ORCA3, ORCA4, and ORCA5 to the GC-rich motif in the STR promoter. The nucleotide sequence of the GC-rich motif and the position of the jasmonate-responsive element (JRE; −100 to −58) relative to the transcription start site (TSS) is shown at top. The autoradiograph shows the DNA-protein complex of the biotin-labeled GC-rich motif probe with ORCA3, ORCA4, or ORCA5. The labeled probe was outcompeted by 1,000× unlabeled probe (+). C, Transactivation of the LAMT and SLS promoters, fused to the firefly luciferase (LUC) reporter, by ORCA3, ORCA4, and ORCA5 in tobacco cells. Control (CN) represents reporter plasmid alone. A plasmid containing the GUS reporter, driven by the CaMV 35S promoter and rbcS terminator, was used as an internal control. LUC and GUS activities were measured 20 h after electroporation. LUC activity was normalized against GUS activity. Data presented here are means ± sd of three biological replicates. Statistical significance was calculated using Student’s t test: *, P < 0.05 and **, P < 0.01.
ORCA TFs Differentially Activate TIA Pathway Genes
The ORCAs are known to regulate a number of genes of the indole pathway and downstream branches (van der Fits and Memelink, 2000; Paul et al., 2017). In this study, we investigated their roles in the regulation of additional genes in the TIA pathway. Biosynthesis of secologanin in C. roseus (Fig. 1A) requires nine enzymes, seven of which are involved in the conversion of geranyl diphosphate to loganic acid and are regulated by BIS1 (Van Moerkercke et al., 2015) and BIS2 (Van Moerkercke et al., 2016). Loganic acid is converted to secologanin by LAMT and SLS. We used protoplast-based transactivation assay to determine whether LAMT and SLS are regulated ORCAs. The LAMT (1,376 bp) or SLS (980 bp) promoter, fused to a firefly luciferase reporter gene, was coelectroporated into tobacco protoplasts with or without the constructs expressing ORCA3, ORCA4, or ORCA5 (Fig. 2C). ORCA3, ORCA4, and ORCA5 significantly activated the LAMT promoter compared with the control. ORCA5, but not ORCA3 and ORCA4, significantly activated the SLS promoter (approximately 2.5-fold) compared with the control (Fig. 2C).
Derepressed CrMYC2a and ORCA5 Have Synergistic Effects on TIA Pathway Genes
A recent study has shown that mutation of a conserved Asp to Asn (D126N) in the JAZ interaction domain of CrMYC2a prevents CrMYC2a from interacting with CrJAZ3 and CrJAZ8, thus derepressing CrMYC2a from the inactive complex with the JAZ proteins. In addition, coexpression of the derepressed CrMYC2a (CrMYC2aD126N) with ORCA3 has a synergistic effect on the expression of several TIA pathway genes (Schweizer et al., 2018). To determine whether the derepressed CrMYC2a acts synergistically with ORCA5, we generated the CrMYC2aD126N mutant by site-directed mutagenesis and evaluated its effect on four key TIA pathway gene promoters, TDC, STR, LAMT, and SLS, which are regulated by ORCA5. As shown in Figure 3, CrMYC2a had no additive effect on the STR promoter activity when coexpressed with ORCA5. The TDC, LAMT, and SLS promoter activities were slightly higher when CrMYC2a was coexpressed with ORCA5. However, coexpression of CrMYC2aD126N with ORCA5 had a synergistic effect on the activation of all four promoters (Fig. 3).
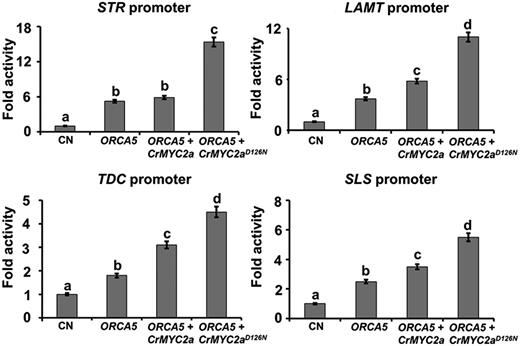
Derepressed CrMYC2a and ORCA5 synergistically affect TIA pathway genes. Activation of the TDC, STR, LAMT, and SLS promoters, fused to the firefly luciferase (LUC) reporter, by ORCA5, CrMYC2a, and CrMYC2aD126N in tobacco cells is shown. A reporter plasmid containing the promoter-LUC cassette was coelectroporated into tobacco protoplasts with effector plasmids harboring TF genes. Control (CN) represents reporter plasmid alone. A plasmid containing the GUS reporter, driven by the CaMV 35S promoter and rbcS terminator, was used as an internal control. LUC and GUS activities were measured 20 h after electroporation. The LUC activity was normalized against the GUS activity. Data presented here are means ± sd of three biological replicates. Different letters denote statistical differences as assessed by one-way ANOVA and Tukey’s HSD test: P < 0.05.
ORCA5 Overexpression Activates TIA Pathway Genes and Boosts TIA Accumulation in C. roseus Hairy Roots
To further elucidate the regulatory role of ORCA5 in TIA biosynthesis, we generated transgenic C. roseus hairy roots overexpressing ORCA5 (ORCA5-OE). The transgenic status of hairy roots was confirmed by PCR (Supplemental Fig. S4A). Two empty vector (EV) control and two overexpression lines (OE-1 and OE-2) were selected for further analysis. Compared with the EV control, expression of ORCA5 was 24- to 40-fold higher in the transgenic lines (Supplemental Fig. S4B). Expression of a number of TIA pathway genes, including ASα, TDC, CPR, G10H, IS, SLS, STR, and SGD, was significantly higher in the ORCA5 overexpression lines compared with the EV control. In addition, expression of the genes encoding C2H2 zinc finger repressors, ZCT1, ZCT2, and ZCT3, was also increased. Interestingly, expression of ORCA3 and ORCA4 was increased significantly in ORCA5-overexpressing hairy roots, suggesting that ORCA5 possibly regulates other members in the ORCA cluster (Fig. 4A).
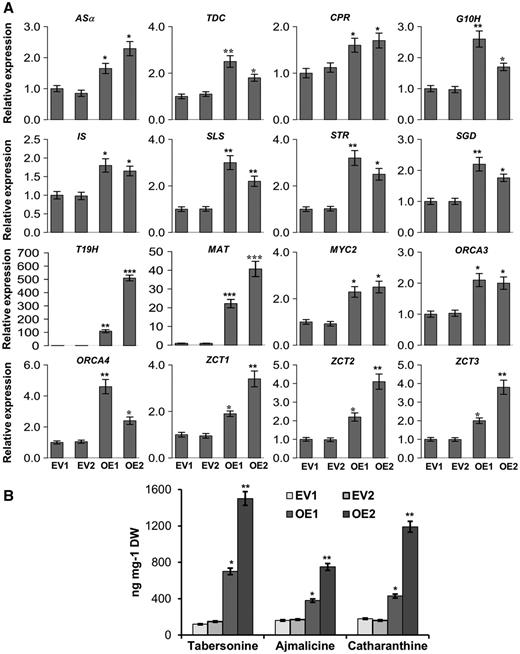
Relative expression of key TIA pathway genes and alkaloid accumulation in ORCA5-overexpressing C. roseus hairy roots. A, Relative expression of the TIA pathway genes and TF genes in two EV controls and two ORCA5-overexpressing hairy root lines (OE-1 and OE-2) as measured by RT-qPCR. B, Measurement of tabersonine, ajmalicine, and catharanthine in EV controls, OE-1, and OE-2. Alkaloids were extracted and analyzed using liquid chromatography-tandem mass spectrometry, and the levels of alkaloids were estimated based on peak areas compared with standards. Data presented here are means ± sd of three biological replicates. Statistical significance was calculated using Student’s t test: *, P < 0.05; **, P < 0.01; and ***, P < 0.001. DW, Dry weight.
Previous studies have shown that overexpression of ORCA3 in C. roseus hairy roots does not result in increased TIA accumulation (Peebles et al., 2009; Wang et al., 2010; Zhou et al., 2010). In this study, overexpression of ORCA5 significantly increased the transcripts levels of genes in both indole (i.e. AS and TDC) and iridoid (i.e. CPR, G10H, IS, and SLS) branches of the TIA pathway. In addition, expression of the downstream pathway genes, STR and SGD, was also significantly increased. To determine the metabolic outcomes of ORCA5 overexpression, we measured the alkaloids in the two independent hairy root lines. Accumulation of tabersonine, ajmalicine, and catharanthine increased significantly in ORCA5-OE lines compared with the EV lines (Fig. 4B).
In C. roseus, tabersonine, ajmalicine, and catharanthine are detected in roots and aerial parts, while vindoline is only accumulated in aerial parts (van der Heijden et al., 2004). Recent studies have shown that four separate hydrolases (HL1–HL4) are involved in the conversion of the unstable intermediate derived from O-acetylstemmadenine to tabersonine by HL1, to catharanthine by HL2, and to vincadifformine by HL3/4 (Qu et al., 2018, 2019; Fig. 1A). In roots, the tabersonine is converted to hörhammericine catalyzed by T19H (Giddings et al., 2011) and MAT (Laflamme et al., 2001). Recently, a BADH acetyltransferase, TAT, has been characterized in C. roseus. TAT is highly expressed in roots and has been shown to acetylate 19-hydroxytabersonine derivatives from C. roseus roots at a higher efficiency than MAT (Carqueijeiro et al., 2018b). In addition, two conserved cytochrome P450s, TEX1 and TEX2, have been identified in C. roseus. TEX1 is preferentially expressed in roots whereas TEX2 transcripts are present in stem, leaf, and flower (Carqueijeiro et al., 2018a). TEX1/2 catalyze the stereoselective epoxidation of tabersonine to lochnericine, which is then converted to hörhammericine by T19H and subsequently acetylated by TAT to form 19-O-acetylhörhammericine. In a parallel branch, a root-specific cytochrome P450, V19H, catalyzes the conversion of vincadifformine to minovincinine, which is then O-acetylated by MAT to form echitovenine (Williams et al., 2019). We found that, similar to other TIA pathway genes, expression of HL2, HL4, T19H, TAT, MAT, and TEX2 was induced by 1.5- to 18-fold in MeJA-treated C. roseus seedlings (Fig. 5A). However, we did not observe significant change in the expression of HL1, HL3, V19H, and TEX1 in response to MeJA treatment. Next, we measured the expression of these genes in EV and ORCA5-OE hairy root lines and found that expression of MAT and T19H was induced by 20- to 500-fold in ORCA5-OE compared with EV (Fig. 4A). Expression of HL3, V19H, TEX1, TEX2, and TAT was also induced by twofold to 11-fold in the ORCA5-OE lines (Fig. 5B), suggesting that these genes are likely regulated by ORCAs. In the ORCA5-OE lines, expression of HL1 and HL4 was slightly repressed whereas HL2 expression did not change significantly. This is similar to a recent study where transient overexpression of ORCA3 and/or MYC2a in C. roseus flower petal had no effect on HL1 and HL2 expression, indicting that additional factors are involved in the regulation of the TIA pathway (Schweizer et al., 2018).
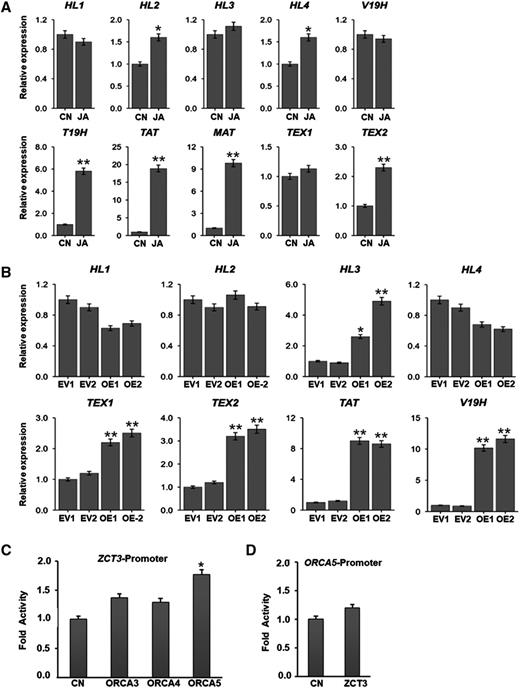
Relative expression of TIA pathway genes in response to MeJA and in ORCA5-overexpressing hairy roots. A, Ten-day-old C. roseus seedlings (15–17 seedlings in each replicate) were treated with 100 μm MeJA (JA) for 2 h, and expression of HL1 to HL4, V19H, TEX1, TEX2, T19H, TAT, and MAT in seedlings was measured by RT-qPCR. Mock-treated seedlings were used as controls (CN). B, Relative expression of HL1 to HL4, V19H, TAT, TEX1, and TEX2 in EV controls and two ORCA5-overexpressing hairy root lines (OE-1 and OE-2) was measured by RT-qPCR. C, Activation of the ZCT3 promoter, fused to the luciferase (LUC) reporter, by ORCA3, ORCA4, or ORCA5 in tobacco cells. D, Transactivation of the ORCA5 promoter, fused to the LUC reporter, in tobacco cells. Control (CN) represents reporter plasmid alone. In both C and D, a plasmid containing the GUS reporter, driven by the CaMV 35S promoter and rbcS terminator, was used as an internal control. LUC and GUS activities were measured 20 h after electroporation. The LUC activity was normalized against the GUS activity. Data presented here are means ± sd of three biological replicates each with four to five samples. Statistical significance was calculated using Student’s t test: *, P < 0.05 and **, P < 0.01.
ORCA5 Activates the ZCT3 Promoter
ZCTs are negative regulators of the TIA pathway (Pauw et al., 2004). In both ORCA4- (Paul et al., 2017) and ORCA5-overexpressing hairy root lines (Fig. 4A), expression of ZCTs was significantly increased. We analyzed the cis-elements in the ZCT promoters and found that the ZCT3 promoter contains putative AP2/ERF-binding sites (GC-rich motif). The findings suggest that ORCA5 regulates ZCT3 possibly by binding to its promoter while indirectly regulating ZCT1 and ZCT2. We thus tested the activation of ZCT3 by ORCAs. The ZCT3 promoter (961 bp) was fused to a firefly luciferase reporter gene and coelectroporated into tobacco protoplasts with or without the constructs expressing ORCA3, ORCA4, or ORCA5 (Fig. 5C). Only ORCA5 moderately but significantly activated the ZCT3 promoter compared with the control. To test whether ORCA5 is regulated by ZCT3, the ORCA5 promoter was fused to a firefly luciferase reporter gene and coelectroporated into tobacco protoplasts with or without the construct expressing ZCT3. No significant repression of the ORCA5 promoter was observed (Fig. 5D).
To demonstrate that ORCA5 activates ZCT3 likely by binding to its promoter, we performed a yeast one-hybrid assay. Plasmids expressing the GAL4-AD-ORCA5 fusion, controlled by the ADH promoter, and the HIS3 nutritional reporter driven by the ZCT3 promoter were cotransformed into yeast cells. Transformed yeast cells, harboring the ZCT3-HIS3 reporter and AD-ORCA5, grew on selection medium lacking His, Leu, and Trp with 50 mm 3-amino-1,2,4-triazole (3-AT), indicating activation of the ZCT3 promoter by ORCA5 (Fig. 6A).
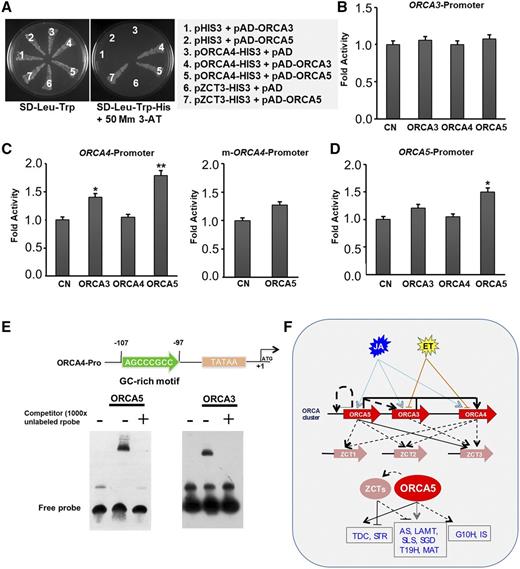
Intracluster regulatory relationship among the members of the ORCA cluster. A, Yeast one-hybrid assay demonstrating activation of the ORCA4 promoter by ORCA3 or ORCA5 and the ZCT3 promoter by ORCA5. ORCA3 or ORCA5, fused to the GAL4 activation domain (pAD-ORCA3/ORCA5), was cotransformed into yeast cells with the pORCA4-HIS3 or pZCT3-HIS3 reporter plasmid. The transformants were grown in either the double synthetic dropout (SD) selection medium lacking Leu and Trp (SD-Leu-Trp) or triple selection medium lacking His, Leu, and Trp (SD-Leu-Trp-His) with 50 mm 3-AT. B to D, Transactivation of the promoters of ORCA3 (B), ORCA4 and mutant ORCA4 (m-ORCA4; C), and ORCA5 (D) by ORCA3, ORCA4, or ORCA5 in tobacco cells. Data presented here are means ± sd of three biological replicates each with four to five samples. Control (CN) represents reporter plasmid alone. Statistical significance was calculated using Student’s t test: *, P < 0.05 and **, P < 0.01. E, Binding of ORCA3 and ORCA5 to the GC-rich motif in the ORCA4 promoter. Nucleotide sequence and position of the GC-rich motif relative to the translation start site are shown at top. The autoradiograph shows the DNA-protein complex of the biotin-labeled probe covering the GC-rich motif with ORCA3 or ORCA5. The binding of the labeled probe was outcompeted by 1,000× unlabeled probe (+). F, Model summarizing the intracluster regulation among the ORCAs and coregulation of ORCAs and ZCTs of the TIA pathway. The ORCA genes are activated by JA but repressed by ET. ORCA3 and ORCA5 regulate ORCA4. ORCA5 regulates its own expression. ORCA5 activates ZCT3 whereas ORCAs indirectly regulate ZCT1 and ZCT2. Solid blue arrows indicate activation by JA; solid yellow T-bars represent repression by ET. Solid black arrows represent direct activation, whereas broken black arrows represent indirect or undetermined activation. ORCA5 activates whereas ZCT represses several genes in the indole and iridoid branches of the TIA pathway.
ORCA5 Activates the ORCA4 Promoter
The expression of both ORCA3 and ORCA4 was increased significantly in ORCA5-OE hairy root lines (Fig. 4A), indicating that ORCA5 possibly regulates the expression of ORCA3 and ORCA4. To test this possibility, ORCA3 (778 bp), ORCA4 (883 bp), and ORCA5 (890 bp) promoters, fused to a firefly luciferase reporter, were coelectroporated into tobacco protoplasts with or without the plasmids expressing ORCA3, ORCA4, and ORCA5. None of the ORCAs could activate the ORCA3 promoter, suggesting that the ORCAs are unable to bind to the promoter despite the induction of ORCA3 in ORCA5-OE lines (Fig. 6B). ORCA3 and ORCA5, but not ORCA4, significantly activated the ORCA4 promoter (Fig. 6C). In addition, ORCA5 activated its own promoter compared with the control (Fig. 6D). However, ORCA3 or ORCA4 had no effects on the transcriptional activity of the ORCA5 promoter (Fig. 6D). The activation of ORCA4 by ORCA3 and ORCA5, activation of ORCA3 and ORCA4 by ORCA5, and self-regulation of ORCA5 indicate the possible presence of autoregulatory and feed-forward loops in the ORCA cluster.
ORCA3 and ORCA5 Bind to the ORCA4 Promoter
We identified a GC-rich motif (AGCCCGCCC) to be a putative AP2/ERF-binding site in the ORCA4 promoter and mutated it to AGCAAAACC by site-directed mutagenesis. The mutant promoter, mORCA4-pro, was fused to the luciferase reporter to generate a reporter vector. The reporter vectors harboring the wild-type or mutant ORCA4 promoter were coelectroporated into tobacco protoplasts with the plasmid expressing ORCA5. Mutation in the GC-rich motif reduced activation of the ORCA4 promoter by ORCA5 (Fig. 6C), suggesting that ORCA5 activates ORCA4 likely by binding to the GC-rich motif in its promoter.
To further verify that ORCA3 and ORCA5 bind the GC-rich element in the ORCA4 promoter, we performed a yeast one-hybrid assay. ORCA3 or ORCA5 fused to the GAL4-AD was cotransformed into yeast cells with the HIS3 reporter driven by the ORCA4 promoter. Yeast cells, harboring the ORCA4-HIS3 reporter and AD-ORCA3 or AD-ORCA5, grew on selection medium lacking His, Leu, and Trp with 50 mm 3-AT, suggesting that ORCA3 and ORCA5 can activate the ORCA4 promoter (Fig. 6A).
We also carried out EMSA to validate the binding of ORCA3 and ORCA5 to the GC-rich motif in the ORCA4 promoter. Recombinant, GST-tagged ORCA3 or ORCA5 protein was purified and incubated with 5′ biotin-labeled probes covering the GC-rich motif of the ORCA4 promoter. Figure 6E shows that ORCA3 and ORCA5 proteins individually interacted with the GC-rich motif, resulting in a mobility shift. The binding of ORCA3 and ORCA5 to the labeled probe was confirmed by a competition experiment using unlabeled (cold) probes. The binding signals of the biotin-labeled probes could be eliminated by excess concentrations (1,000×) of cold probe (Fig. 6E), suggesting that ORCA3 or ORCA5 binds to the GC-rich motif in the ORCA4 promoter.
GC-Rich Motifs Are Present in the Promoters of AP2/ERF Gene Clusters in Other Plants
AP2/ERFs are known to bind the GC-rich motifs in target gene promoters (Fujimoto et al., 2000; Shoji et al., 2013). Group IX ERFs bind differentially to three GC-rich motifs, P-box (CCGCCCTCCA), CS1-box (TAGACCGCCT), and GCC-box (AGCCGCC; Shoji et al., 2013). A recent study has identified a consensus sequence for GC-rich motifs ([A/C]GC[A/C]C[T/C][C/T]C) present in the promoters of nicotine biosynthetic genes in tobacco (Kajikawa et al., 2017). In addition, ORCA3 and ORCA5 bind to a GC-rich motif (AGCCCGCC; this study) in the ORCA4 promoter. The question thus arose whether GC-rich elements are also present in the promoters of AP2/ERF gene clusters identified in other plant species. To address this question, we manually searched for similar GC-rich motifs approximately 1 kb 5′ of the protein-coding regions of NIC2 and GAME9 genes in tobacco, tomato, and potato. Of the 10 NIC2 promoters, two GC-rich sequences were found each of ERF168, ERF115, and ERF179. Both tomato GAME9-like1 (Solyc01g090300) and GAME9-like2 (Solyc01g090310) contain a single GC-rich sequence, while potato GAME9-like2, GAME9-like3, GAME9-like4, and GAME9-like7 (Cárdenas et al., 2016) contain several in their promoters (Supplemental Fig. S5).
Intracluster and Mutual Regulation in AP2/ERF Gene Clusters
The conserved nature of the GC-rich elements in promoters of the AP2/ERF clusters indicates the possible intracluster regulatory mechanisms that are mutually shared among different plant species. To test this possibility, the C. roseus ORCA4 promoter-luciferase reporter construct was coelectroporated into tobacco protoplasts with or without the plasmids expressing tobacco ERF189 or ERF221. Both tobacco ERF189 and ERF221 significantly activated the ORCA4 promoter compared with the control (Fig. 7A). Similarly, the tobacco ERF115 (1,056 bp) or ERF179 (1,070 bp) promoter-luciferase reporter construct was coelectroporated into tobacco protoplasts with or without the construct expressing ERF189 or ORCA5. The activation of the ERF115 and ERF179 promoters by ERF189 or ORCA5 was moderate but statistically significant (Fig. 7B). In addition, we found two potential ERF-binding motifs (GGCACCT and GGCCAAGC) in the ERF115 promoter. Mutation of either individual motif did not significantly affect the activation of ERF115-luciferase by ERF189; however, mutation of both motifs reduced the activity of ERF115-luciferase reporter by 70% compared with the wild-type promoter (Fig. 7C). Collectively, these findings suggest the presence of intracluster and mutual regulation in both NIC2 and ORCA clusters.
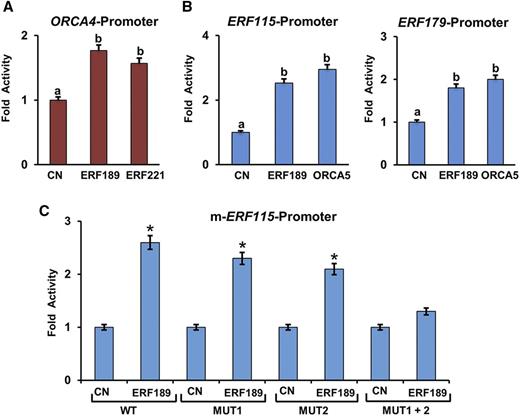
Mutual regulatory relationship among C. roseus ORCA and tobacco NIC2 AP2/ERFs. A and B, Transactivation of the ORCA4 promoter by NIC2 ERF, ERF189, or ERF221 (A) and the tobacco ERF115 and ERF179 promoters by ERF189 or ORCA5 (B). Data represent means ± sd of three biological samples. Different letters denote statistical differences as assessed by one-way ANOVA and Tukey’s HSD test: P < 0.05. C, Transactivation of the mutant ERF115 promoter by ERF189 in a tobacco protoplast-based transactivation assay. A plasmid containing the GUS reporter, driven by the CaMV 35S promoter and rbcS terminator, was used as an internal control. Control (CN) represents reporter plasmid alone. The luciferase (LUC) and GUS activities were measured 20 h after electroporation. The LUC activity was normalized against the GUS activity. Data presented here are means ± sd of three biological replicates each with four to five samples. Statistical significance was calculated using Student’s t test: *, P < 0.05. WT, Wild type.
C. roseus ORCA ERFs and Tobacco NIC2-Locus ERFs Are Likely Interchangeable
C. roseus ORCA3/4/5 are homologous to tobacco NIC2 locus AP2/ERFs, ERF189 and ERF221 (a.k.a. ORC1; Shoji et al., 2010; De Boer et al., 2011). In addition, both ORCAs and NIC2 ERFs are induced by MeJA and recognize GC-rich motifs in target gene promoters in two diverse metabolic pathways (Shoji et al., 2010; De Boer et al., 2011). It is thus intriguing to speculate that C. roseus ORCAs and tobacco NIC2-locus ERFs are functionally equivalent and interchangeable. We tested this assumption by coelectroporation of the putrescine N-methyltransferase (PMT; 1,500 bp) or quinolinate phosphoribosyltransferase (QPT; 1,579 bp) promoter-luciferase reporter vector into tobacco protoplasts with or without the plasmids expressing ERF221, ORCA3, ORCA4, or ORCA5. As expected, ERF221 significantly activated the PMT and QPT promoters compared with the control. ORCA3 and ORCA5 also activated the PMT and QPT promoters, although to lower levels compared with the activation by ERF221 (Fig. 8A). The STR promoter (587 bp) fused to the luciferase reporter was coelectroporated into tobacco protoplasts with or without the construct expressing ORCA3, ERF189, or ERF221. Similar to ORCA3, a known STR activator, both ERF189 and ERF221 significantly activated the STR promoter (Fig. 8A), suggesting that tobacco ERF189 and ERF221 are functional equivalents of C. roseus ORCAs. To determine the activation specificity of PMT and QPT by NIC2 ERFs or ORCAs, we cloned a tobacco bZIP TF that is not involved in the regulation of nicotine biosynthesis (Yang et al., 2001) and used it as a negative control. As shown in Supplemental Figure S6, the bZIP TF was unable to activate the PMT or QPT promoter in tobacco cells. Similarly, CrMYC1, a C. roseus bHLH TF not known to regulate the TIA pathway (Chatel et al., 2003), was unable to activate the STR promoter in tobacco cells.
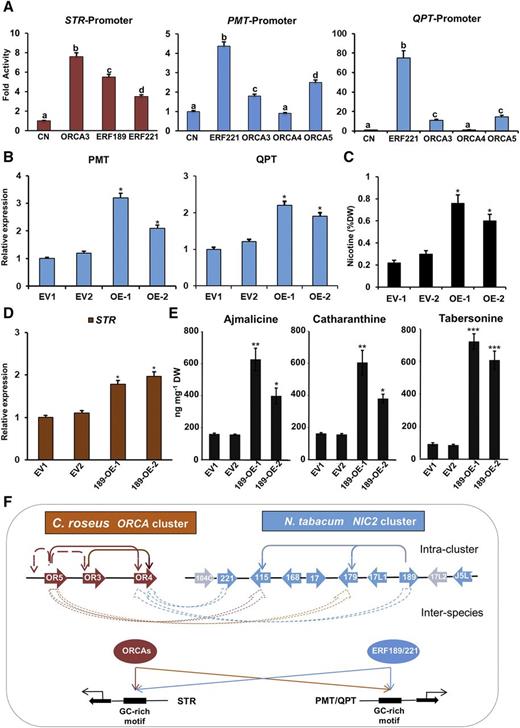
C. roseus ORCAs and tobacco NIC2 ERFs are likely interchangeable. A, Transactivation of C. roseus STR promoter by ORCA3, ERF189, or ERF221 and tobacco PMT and QPT promoters by ERF221, ORCA3, ORCA4, or ORCA5 in the tobacco protoplast assay. A plasmid containing the GUS reporter, driven by the CaMV 35S promoter and rbcS terminator, was used as an internal control. Control (CN) represents reporter plasmid alone. The luciferase (LUC) and GUS activities were measured 20 h after electroporation. The LUC activity was normalized against the GUS activity. Data represent means ± sd of three biological replicates each with four to five samples. Different letters denote statistical differences as assessed by one-way ANOVA and Tukey’s HSD test: P < 0.05. B, Relative expression of PMT and QPT in two EV control (EV1 and EV2) and two ORCA5-overexpressing (OE-1 and OE-2) tobacco hairy root lines, as measured by RT-qPCR. Tobacco elongation factor1α (EF1α) was used as an internal control. C, Nicotine contents in two EV control (EV1 and EV2) and two ORCA5-overexpressing (OE-1 and OE-2) tobacco hairy root lines. Nicotine concentrations are presented as percentage dry weight (%DW). D, Relative expression of STR in EV1 and EV2 (control) and two ERF189-overexpressing (189-OE-1 and 189-OE-2) C. roseus hairy root lines, as measured by RT-qPCR. C. roseus EF1α was used as an internal control. E, Measurement of ajmalicine, catharanthine, and tabersonine in EV1 and EV2 controls, OE-1, and OE-2. Alkaloids were extracted and analyzed using liquid chromatography-tandem mass spectrometry, and the levels of alkaloids were estimated based on peak areas compared with standards. Data presented here are means ± sd of three biological replicates. Statistical significance was calculated using Student’s t test: *, P < 0.05; **, P < 0.01; and ***, P < 0.001. F, Model depicting the mutual regulatory relationship among and between the ORCA and NIC2 locus AP2/ERFs. Thin solid arrows represent direct activation, and thin broken arrows represent indirect activation within a cluster. Thick arrows indicate the interspecies mutual regulation of the ERFs.
To functionally verify the conserved regulatory roles of AP2/ERFs of different clusters, we generated tobacco hairy roots overexpressing ORCA5. The transgenic status of the hairy roots was confirmed by PCR (Supplemental Fig. S7), and two hairy root lines were used for further analysis. Expression of PMT and QPT was 2.5- to 3- fold higher in ORCA5-expressing hairy roots compared with the EV control (Fig. 8B). Moreover, nicotine contents in the two ORCA5-overexpressing lines were threefold to fourfold higher compared with the control lines (Fig. 8C), a result that is consistent with a previous study showing that overexpression of ERF189 in tobacco hairy roots resulted in 2- to 3-fold increases in PMT and QPT expression and alkaloid accumulation (Shoji et al., 2010). We also generated C. roseus hairy roots overexpressing ERF189, and two transgenic lines were used for further analysis (Supplemental Fig. S8). STR expression was approximately 2-fold higher in ERF189-expressing hairy roots compared with the EV control (Fig. 8D). In addition, the two ERF189-overexpressing lines accumulated 2- to 7-fold higher ajamalicine, catharanthine, and tabersonine compared with the controls (Fig. 8E).
DISCUSSION
Physically linked clusters of nonhomologous, structural genes have been identified in numerous plant species, including Arabidopsis, rice (Oryza sativa), maize (Zea mays), oat (Avena sativa), tomato, potato, and opium poppy (Papaver somniferum; Boycheva et al., 2014; Nützmann and Osbourn, 2014; Nützmann et al., 2016). These gene clusters generally encode enzymes that are involved in the biosynthesis of specialized metabolites (Boycheva et al., 2014; Nützmann and Osbourn, 2014; Nützmann et al., 2016). Unlike the structural gene clusters, TF gene clusters comprise homologous genes that likely arose as the result of duplication events. It is unclear whether the duplicated TF genes are functionally redundant and coregulated by the same transcriptional circuit or if they have evolved through gene divergence to possess unique functions, including differential responses to hormonal signals and regulation of one another.
We showed that ORCAs and key TIA pathway genes exhibit two distinct expression patterns in response to ET alone or the combined treatment of ET and MeJA (Fig. 1B). CrMYC2a, ORCA5, and TDC were up-regulated by MeJA but not affected by ET. On the other hand, expression of ORCA3, ORCA4, and STR was significantly induced by MeJA and repressed by ET. Moreover, when treated simultaneously, ET antagonizes the MeJA-induced expression of ORCA3, ORCA4, ORCA5, TDC, and STR (Fig. 1B). Expression divergence has been observed among the tobacco NIC2 ERFs (Shoji et al., 2010) and tomato JREs (Nakayasu et al., 2018) in response to MeJA and ET. MeJA-induced expression of ERF189/199 is antagonized by ET, whereas the expression of other NIC2 ERFs is insensitive to ET treatment (Shoji et al., 2010). Other duplicated regulatory genes in Arabidopsis also exhibit expression divergence (Ganko et al., 2007). The plant genomes sequenced to date have shown whole-genome, tandem, and/or segmental duplications that result in neofunctionalization, subfunctionalization, or pseudofunctionalization of duplicated genes (Rensing et al., 2008; Chae et al., 2014). These findings suggest that duplicated regulatory genes, including ORCA and NIC2 ERFs, experience subfunctionalization (Shoji et al., 2010).
We demonstrated that ORCA5 has a broader transactivation specificity than ORCA3 and ORCA4 (Fig. 2C). Overexpression of ORCA5 in C. roseus hairy roots significantly induced the expression of genes in the indole branch and downstream of the iridoid branch, such as SLS, resulting in increased TIA accumulation (Fig. 4A). In addition, expression of CrMYC2a was also up-regulated in ORCA5-overexpressing hairy roots. In tobacco, not all NIC2 ERFs are equally effective in activating nicotine pathway genes. This functional divergence among the ERFs may be attributed to the sequence differences in the AP2 DNA-binding domain and/or the region outside of the AP2 domain (Shoji et al., 2010). MYC2 is known to regulate plant specialized metabolites, including nicotine, TIAs, and SGA. Previously, we have demonstrated that CrMYC2a expression strongly correlates with those of TIA structural and regulatory genes. Similar to MYC2 regulation of nicotine biosynthesis in tobacco (Shoji and Hashimoto, 2011), CrMYC2a coregulates TIA pathway genes with ORCA3 (Paul et al., 2017). In addition, CrMYC2a expression is induced in response to JA (Fig. 1B) and increased in ORCA5-overexpressing hairy roots (Fig. 4A). A recent study has shown that transient co-overexpression of a derepressed CrMYC2a (CrMYC2aD126N) with ORCA3 synergistically affects the expression of several TIA pathway genes (Schweizer et al., 2018). Similar to the previous study, we found that CrMYC2aD126N, when coexpressed with ORCA5, has additive effects on the activation of TIA pathway genes. Collectively, these findings suggest that CrMYC2a and ORCAs are part of a regulatory network that modulates TIA biosynthesis in C. roseus. Transient overexpression of CrMYC2a or CrMYC2aD126N alone or in combination with ORCA3 activates a limited number of TIA pathway genes, suggesting that additional but unidentified TFs are likely involved in the TIA gene regulatory network.
Positive and negative regulatory loops are the hallmarks of metabolic pathways in plants. In the Arabidopsis JA signaling pathway, MYC2 activates the expression of JAZ repressors, which, in turn, interact with MYC2 to attenuate the intensity of the JA signal (Chini et al., 2007; Kazan and Manners, 2013). AtMYBL2, a repressor of anthocyanin biosynthesis in Arabidopsis, is regulated by the bHLH activator, TRANSPARENT TESTA8 (TT8). AtMYBL2 competes with the R2R3 MYBs, PAP1 and PAP2, to form a complex with TT8 that represses anthocyanin accumulation (Matsui et al., 2008). Recently, we demonstrated that in C. roseus, CrMYC2a and BIS1 activate the expression of the bHLH TF, RMT1, which acts as a repressor of CrMYC2a targets (Patra et al., 2018). Similarly, in tomato, MYC2 regulates the expression of a small group of JA-responsive bHLH TFs, MYC2-targeted bHLH1 (MTB1), MTB2, and MTB3. MTB proteins inhibit the formation of the MYC2-MED25 complex and compete with MYC2 to bind to its targets (Liu et al., 2019). Here, we showed that, similar to ORCA4 (Paul et al., 2017), overexpression of ORCA5 significantly activates ZCTs in C. roseus hairy roots (Fig. 4A). Moreover, we demonstrated that ORCA5 activates ZCT3 possibly by binding to the GC-rich element in the promoter (Figs. 5C and 6A). In C. roseus cells, ZCTs repress STR and TDC, the direct targets of ORCAs, by binding to their promoters (Pauw et al., 2004). The up-regulation of ZCTs by ORCA4 or ORCA5 overexpression suggests the existence of a negative regulatory loop that is probably involved in the fine-tuning of TIA biosynthesis (Fig. 6F).
Individual genes in the tobacco and C. roseus ERF gene clusters play overlapping and unique roles in controlling the structural genes in nicotine and TIA biosynthetic pathways, respectively (Shoji et al., 2010; Paul et al., 2017). However, the regulatory relationship among the members within an ERF cluster, or any known plant TF clusters, has not been elucidated prior to this study. Here, we demonstrated that an intracluster regulatory mechanism exists in both the C. roseus ORCA cluster and the tobacco NIC2 cluster. The self-regulated ORCA5 activates ORCA4 by binding to its promoter and ORCA3, likely through an uncharacterized TF. ORCA3 also activates ORCA4 by binding to the GC-rich motif in its promoter (Fig. 6). In tobacco, ERF189 activates both the ERF115 and ERF179 promoters (Fig. 7B). GC-rich sequences are not found within the 1-kb promoter regions of ERF189 and GAME9. The fact that GC-rich motifs are present only in the promoters of some ERFs indicates that certain key regulators, such as ERF189, likely play important roles in controlling the amplification loop in the ERF cluster. The intracluster regulation of ERF clusters implies that the individual components within a cluster are not simply redundant duplications of one another. The positive amplification loops help plants to make sufficient precursors required for the spatiotemporal biosynthesis of specialized metabolites. The mechanism is likely conserved in other ERF clusters in plants. It is also reasonable to predict that similar self-regulation mechanisms exist in other TF clusters, such as those formed by group III AP2/ERFs, CBFs (Zhang et al., 2004), and ARFs (Hagen and Guilfoyle, 2002).
The conserved nature of the intra-ERF-cluster regulation prompted us to speculate that ERFs from gene clusters of different plant species are functionally equivalent and interchangeable, despite low sequence similarity (35% to 51%). In this study, we showed that C. roseus ORCA5 can activate both tobacco ERF115 and ERF179, and tobacco ERF189 and ERF221 can activate the C. roseus ORCA4 promoter (Fig. 7, A and B). Furthermore, ORCA3 and ORCA5 can activate the PMT and QPT promoters (Fig. 8A). Similarly, the STR promoter in the TIA pathway can be activated by tobacco ERF189 and ERF221 (Fig. 8A). Moreover, ORCA5 overexpression in tobacco hairy roots induced the expression of PMT and QPT, resulting in increased nicotine accumulation (Fig. 8, B and C). Similarly, ERF189 overexpression in C. roseus hairy roots activated the expression of STR and induced TIA accumulation (Fig. 8, D and E). The mutual activations of two distinct metabolic pathways by the ORCA and NIC2 clusters support our hypothesis that the AP2/ERFs are functionally equivalent and are likely interchangeable (Fig. 8F). Other ERF gene clusters, such as GAME9 ERFs of tomato and potato (Supplemental Fig. S5), also contain the GC-rich elements in their promoters and respond to JA induction similar to the ORCA and NIC2 clusters. We thus propose that the intracluster and mutual regulatory functions are widely conserved among the ERF gene clusters of diverse plant species, although additional experimental verifications are required.
TF gene clusters have been identified in nonplant organisms, including nematodes, Drosophila, mouse, and human. The nonplant, homeodomain HOX TF clusters, which play critical roles in invertebrate and vertebrate development, have been characterized (Lappin et al., 2006; Montavon and Duboule, 2013). By comparison, the plant TF clusters are poorly investigated. As more and more TF clusters are being identified, the unique functions and mutual regulatory relationships of the clustered TFs require in-depth examination. Central to the knowledge gaps is the regulatory relationship within a cluster and among different species. Understanding of such relationships will shed light on TF evolution as well as the functional equivalence and divergence of TFs involved in specialized metabolism. This study demonstrates intracluster and mutual regulation of AP2/ERF gene clusters, suggesting that a conserved regulatory mechanism modulates the biosynthesis of diverse groups of plant specialized metabolites.
MATERIALS AND METHODS
Plant Materials
Catharanthus roseus ‘Little Bright Eye’ (NE Seed) was used for cloning, gene expression, and generation of transgenic hairy roots. The tobacco (Nicotiana tabacum) ‘Xanthi’ cell line was used for protoplast-based transient expression assays. Tobacco ‘Samsun NN’ was used for the generation of hairy roots.
RNA Isolation and cDNA Synthesis
C. roseus ‘Little Bright Eye’ seeds were surface sterilized using 30% (v/v) commercial bleach for 15 min, washed five times with sterile water, and inoculated on one-half-strength Murashige and Skoog (MS) medium (Caisson Labs). The plates were kept at 28°C in the dark for 2 d and then transferred to a growth room at 28°C with constant light (Patra et al., 2018). Ten-day-old seedlings were immersed in one-half-strength MS medium with 100 μm MeJA and/or 50 μm ET precursor, ACC, for 2 h. Mock-treated seedlings were used as controls. Total RNA isolated from the seedlings was used for cDNA synthesis as described previously (Suttipanta et al., 2007).
RT-qPCR
RT-qPCR was performed as described previously (Suttipanta et al., 2011). The primers used in RT-qPCR are listed in Supplemental Table S1. In addition to the C. roseus EF1α, 40S Ribosomal Protein S9 was used as a second internal control (Liscombe et al., 2010). All PCRs were performed in triplicate and repeated at least twice.
Total RNAs isolated from EV control and ORCA5-overexpressing hairy roots were used for cDNA synthesis and RT-qPCR as previously described (Suttipanta et al., 2011). The comparative cycle threshold method (Applied Biosystems) was used to measure transcript levels. In addition to tobacco EF1α (Shoji et al., 2010; GenBank accession no. D63396), α-tubulin (GenBank accession no. AJ421411) was also used as a reference gene.
Subcellular Localization
For subcellular localization, the full-length cDNA of ORCA5 was fused to the N terminus of the eGFP driven by the CaMV 35S promoter and rbcS terminator in a pBS plasmid to generate pORCA5-eGFP. A pBS plasmid containing only eGFP was used as a control. The plasmids containing either eGFP or ORCA5-eGFP were individually electroporated into tobacco protoplasts as described previously (Pattanaik et al., 2010b) and visualized after 20 h of incubation in the dark with a fluorescence microscope (Eclipse TE200; Nikon).
Tobacco Protoplast Isolation and Electroporation
The 5′ flanking regions of LAMT (−1,375 to −1; relative to the ATG), SLS (−979 to −1), STR (−586 to −1), ZCT3 (−960 to −1), ORCA3 (−777 to −1), ORCA4 (−882 to −1), and ORCA5 (−889 to −1) promoters were PCR amplified from C. roseus genomic DNA. The PMT (−1,499 to −1), QPT (−1,578 to −1), ERF115 (−1,055 to −3), and ERF179 (−1,069 to −3) promoters were amplified from tobacco genomic DNA using gene-specific primers. The two GC-rich motifs, TGGCACCT and GGCCAAGC, in the ERF115 promoter were mutated to aaaACCT and GaaaAAGC using site-directed mutagenesis. The reporter plasmids for transient protoplast assays were generated by cloning LAMT, SLS, STR, ZCT3, ORCA3/4/5, PMT, ERF115, and ERF179 promoters in a modified pUC vector containing a firefly luciferase and rbcS terminator. The effector plasmids were constructed by cloning ORCA3/4/5, ERF189/221, and ZCT3 into a modified pBS vector under the control of the CaMV 35S promoter and rbcS terminator. The GUS driven by the CaMV 35S promoter and rbcS terminator was used as an internal control in the protoplast assay. For the transactivation assay, ORCA3, ORCA4, and ORCA5 were fused to the GAL4 DNA-binding domain in a pBS plasmid containing a mirabilis mosaic virus promoter and rbcS terminator. The reporter plasmid used in the assay contains firefly luciferase driven by a minimal CaMV 35S promoter with five tandem repeats of GAL4 Response Elements and rbcS terminator fused. Protoplast isolation from tobacco cell suspension cultures and electroporation with supercoiled plasmid DNA were performed using previously described protocols (Pattanaik et al., 2010b). The reporter, effector, and internal control plasmids were electroporated into tobacco protoplasts in different combinations; luciferase and GUS activities in transfected protoplasts were measured as described previously (Suttipanta et al., 2007). Each experiment was repeated three times.
Construction of a Plant Expression Vector and Generation of Hairy Roots
For plant transformation, ORCA5 and ERF189 were PCR amplified from C. roseus and tobacco seedling cDNA, respectively, and cloned in pCAMBIA2301 vector containing the CaMV 35S promoter and the rbcS terminator (Pattanaik et al., 2010a). The pCAMBIA2301 vector alone was used as an EV control. The plasmids were mobilized into Agrobacterium rhizogenes R1000 by freeze-thaw. Transformation of C. roseus seedlings and generation of hairy roots were performed using the protocol described previously (Suttipanta et al., 2011; Paul et al., 2017). The transgenic status of the hairy root lines was verified by PCR amplification of rolB, rolC, virC, nptII, and GUS genes. Primers used in this study are listed in Supplemental Table S1. Two independent hairy root lines were selected for further analysis.
Alkaloid Extraction and Analysis
For extraction of alkaloids, 10-d-old seedlings were immersed in one-half-strength MS medium with 100 μm MeJA and/or 50 μm ACC for 24 h. MeJA- and/or ACC-treated seedlings and transgenic hairy roots were frozen in liquid nitrogen and ground to powder. Samples were extracted in methanol (1:100, w/v) twice for 24 h on a shaker. Pooled extracts were then dried via a rotary evaporator and diluted in methanol (10 μL mg−1 of the initial sample). The samples were then analyzed using HPLC, followed by electrospray injection in a tandem mass spectrometer, as previously described (Suttipanta et al., 2011; Paul et al., 2017). The known alkaloid standards were run to identify elution times and mass fragments.
Yeast One-Hybrid Assay
The ORCA4 (883 bp)/ZCT3 (961 bp) promoter was cloned in the pHIS2 vector (Clontech), containing the HIS3 reporter gene, to generate the reporter plasmid (pORCA4/ZCT3-HIS3). The full-length ORCA3 and ORCA5 cDNAs were cloned into the yeast expression plasmid, pAD-GAL4-2.1 (Stratagene), to generate the effector plasmids (pORCA3/ORCA5-AD). The reporter and effector plasmids were transformed into yeast strain Y187, and transformants were selected on SD medium lacking Leu and Trp. Transformed colonies were then streaked on SD medium lacking His, Leu, and Trp with 50 mm 3-AT to check promoter activation.
Recombinant Protein Production and EMSA
The ORCA3, ORCA4, and ORCA5 genes were cloned into the pGEX 4T-1 vector (GE Healthcare Biosciences) to generate GST fusion proteins. The constructs were verified by DNA sequencing and transformed into Escherichia coli BL21 cells containing pRIL (Agilent). Protein expression was induced by adding isopropyl β-d-thiogalactopyranoside to a final concentration of 0.1 mm to the cell cultures at A600 ∼ 0.8 and induced for 2 h at 37°C. The cells were harvested by centrifugation and lysed using CelLytic B (Sigma) according to the manufacturer’s instructions. The GST fusion proteins were bound to Glutathione Sepharose 4B columns (Amersham) and eluted by using 10 mm reduced glutathione in 50 mm Tris-HCl (pH 8) buffer. Bacterial expression and purification of recombinant CrMYC2a protein were performed as previously described (Patra et al., 2018). For EMSA experiments, biotin-labeled DNA probes were synthesized by Integrated DNA Technologies and annealed to produce double-stranded probes. cDNA probes were designed to include the jasmonate-responsive elements of the STR promoter (−100ACATCACTCTTAGACCGCCTTCTTTGAAAGTGATTTCCCTTGGACCTT−58 relative to the transcription start site; van der Fits and Memelink, 2001) and putative GC-rich element of the ORCA4 promoter (−106CCTTCATAGCCCGCCCAATTGGTAAACGTGCACCAACCTCC−66 relative to the translation start, ATG). The EMSA experiment was carried out using a light-shift chemiluminescent EMSA kit (Thermo Fisher Scientific). For the binding reactions, 40 fmol of double-stranded DNA was incubated with purified protein (500 ng of each protein) for 60 min at room temperature. The protein-DNA binding for ORCA5 was further confirmed by performing competition experiments, where 10-, 100-, and 1,000-fold excess amounts of cold probe (without biotin label) were added to the binding reactions. For ORCA3 and ORCA4, a 1,000-fold excess amount of cold probe was added to the binding reactions. Recombinant CrMYC2a protein was used as a negative control on biotin-labeled STR probe. The DNA-protein complexes were resolved by electrophoresis on 6% nondenaturing polyacrylamide gels and then transferred to BiodyneB modified membrane (0.45 mm; Pierce). The band shifts were detected by a chemiluminescent nucleic acid detection module (Pierce) and exposed to x-ray films.
Phylogenetic Analysis of Group IX AP2/ERFs
Protein sequences for tobacco, tomato (Solanum lycopersicum), and potato (Solanum tuberosum) were downloaded from the Sol Genomics Network database (Fernandez-Pozo et al., 2015), and protein sequences for C. roseus were obtained from the Medicinal Plant Genomics Resource database (http://medicinalplantgenomics.msu.edu/). The Arabidopsis (Arabidopsis thaliana) AP2/ERFs sequences were obtained from a previously published report (Nakano et al., 2006). The group IX AP2/ERF protein sequences from Arabidopsis were used as queries using BLAST (Camacho et al., 2009) to identify the AP2/ERFs from tobacco, tomato, potato, and C. roseus. Putative AP2/ERF sequences were screened using the Pfam database for the AP2/ERF domain (Finn et al., 2016). The group IX AP2/ERF protein sequences were aligned using ClustalW with the default settings, and MEGA6.0 was used to construct the phylogenetic tree using the neighbor-joining method with bootstrap values set as 1,000 replicates. The tree image was generated with the Evolview v2 (He et al., 2016).
Generation of Tobacco Hairy Roots and Measurement of Nicotine
Leaf discs of in vitro-grown tobacco ‘Samsun NN’ plantlets were infected with A. rhizogenes strain R1000 harboring the pCAMBIA2301-ORCA5 overexpression construct. After 2 d of co-cultivation, leaf discs were transferred to MS medium supplemented with 400 mg L−1 cefotaxime and kept at 25°C in the dark. Hairy roots developed from the leaf discs were transferred to MS medium with 400 mg L−1 cefotaxime and 100 mg L−1 kanamycin for further proliferation.
Freeze-dried EV control and ORCA5-overexpressing hairy roots were exhaustively extracted for pyridine alkaloids by methyl tert-butyl alcohol and aqueous sodium hydroxide. Alkaloid contents were determined using gas chromatography with flame ionization detection (Perkin Elmer; Lewis et al., 2008). Nicotine content was reported as percentages on a dry tobacco weight basis.
Statistical Analyses
The data presented here were statistically analyzed by Student’s t test or one-way ANOVA and Tukey’s HSD test for multiple comparisons. The significance levels (P values) are described in the figure legends.
Accession Numbers
Accession numbers are as follows: AJ251249 (ORCA3), KR703577 (ORCA4), KR703578 (ORCA5), AB827951 (ERF189), and XM_016622819 (ERF221).
Supplemental Data
The following supplemental materials are available.
Supplemental Figure S1. Phylogenetic analysis of group IX AP2/ERFs in tobacco, tomato, potato, and C. roseus.
Supplemental Figure S2. Subcellular localization of ORCA5 and transactivation assay of ORCAs in tobacco cells.
Supplemental Figure S3. ORCA5 and CrMYC2a binding to the GC-rich motif in the STR promoter.
Supplemental Figure S4. Molecular analysis of ORCA5-overexpressing C. roseus hairy roots.
Supplemental Figure S5. Positions and sequences of GC-rich motifs in the AP2/ERF promoters of C. roseus, tobacco, tomato, and potato.
Supplemental Figure S6. Activation assays of the QPT, PMT, and STR promoters using tobacco bZIP and CrMYC1.
Supplemental Figure S7. Molecular analysis of ORCA5-overexpressing tobacco hairy roots.
Supplemental Figure S8. Molecular analysis of ERF189-overexpressing C. roseus hairy roots.
Supplemental Table S1. Oligonucleotides used in this study.
ACKNOWLEDGMENTS
We thank John May (Department of Civil Engineering and Environmental Research Training Laboratories, University of Kentucky) for assistance on liquid chromatography-tandem mass spectrometry and Huihua Ji (Kentucky Tobacco Research and Development Center, University of Kentucky) for assistance on nicotine measurement.
LITERATURE CITED
Author notes
This work was supported by the Harold R. Burton Endowed Professorship to L.Y. and by the National Science Foundation under cooperative agreement 1355438 to L.Y.
Articles can be viewed without a subscription.
These authors contributed equally to the article.
Senior authors.
The author responsible for distribution of materials integral to the findings presented in this article in accordance with the policy described in the Instructions for Authors (www.plantphysiol.org) is: Ling Yuan ([email protected]).
L.Y. and S.P. designed the research; P.P., S.K.S., B.P., X.L., and S.P. performed experiments; P.P., S.K.S., and S.P. analyzed data; P.P., S.K.S., S.P., and L.Y. wrote the article.