-
PDF
- Split View
-
Views
-
Cite
Cite
Takeshi Yoshihara, Edgar P. Spalding, Switching the Direction of Stem Gravitropism by Altering Two Amino Acids in AtLAZY1 , Plant Physiology, Volume 182, Issue 2, February 2020, Pages 1039–1051, https://doi.org/10.1104/pp.19.01144
- Share Icon Share
Abstract
From germination to flowering, gravity influences plant growth and development. A rice (Oryza sativa) mutant with a distinctly prostrate growth habit led to the discovery of a gene category that participates in the shaping of plant form by gravity. Each so-called LAZY gene includes five short regions of conserved sequence. The importance of each of these regions in the LAZY1 gene of Arabidopsis (Arabidopsis thaliana; AtLAZY1) was tested by mutating each region and measuring how well transgenic expression of the resulting protein variant rescued the large inflorescence branch angle of an atlazy1 mutant. The effect of each alteration on subcellular localization was also determined. Region I was required for AtLAZY1 to reside at the plasma membrane, which is necessary for its function. Mutating region V severely disrupted function without affecting subcellular localization. Regions III and IV could be mutated without large impact on function or localization. Altering region II with two conservative amino acid substitutions (L92A/I94A) had the profound effect of switching shoot gravity responses from negative (upward bending) to positive (downward bending), resulting in a “weeping” inflorescence phenotype. Mechanical weakness of the stem was ruled out as an explanation for the downward bending. Instead, experiments demonstrated that the L92A/I94A change to AtLAZY1 reversed the auxin gradient normally established across stems by the gravity-sensing mechanism. This discovery opens up new avenues for studying how auxin gradients form across organs and new approaches for engineering plant architecture for agronomic and other practical purposes.
The gravity vector is a source of information that the shoot system uses to orient growth of its components. For example, primary and secondary stems (Fukaki et al., 1996a, 1996b; Yamauchi et al., 1997; Roychoudhry et al., 2013; Yoshihara et al., 2013), leaf petioles (Mano et al., 2006), floral pedicels (Wei et al., 2010), and monocot tillers (Abe et al., 1996; Wu et al., 2013; Sang et al., 2014) each use gravity to guide growth at an angle that evolution has selected and the environment modified. Understanding how plants decode the gravity vector to orient growth is thus an important topic in plant biology.
A gravity-directed change in growth direction, or gravitropism, begins with a perception process that causes a difference in the cell elongation rate across the organ (Morita, 2010; Singh et al., 2017; Su et al., 2017). The growth differential across the organ, which produces the appropriate degree of curvature, results from the redistribution of the growth hormone auxin to the lower side of the organ. The perception event relies on starch-filled plastids called statoliths settling to the lowest point in the sensory cells, which in Arabidopsis (Arabidopsis thaliana) inflorescence stems are the endodermal cells surrounding the vascular bundles in the growing region (Tasaka et al., 1999; Saito et al., 2005). The subsequent auxin redistribution phase relies on the PIN-FORMED (PIN) auxin transport proteins relocating within the endodermal cells (Rakusová et al., 2015). As a result, growth-promoting concentrations of auxin accumulate on the lower side of the organ and upward bending ensues.
A plant-specific LAZY gene family, discovered through characterization of a rice (Oryza sativa) mutant with unusually wide tiller angles (Li et al., 2007; Yoshihara and Iino, 2007), encodes proteins that function in gravitropism. Studies of lazy mutants in Arabidopsis, maize (Zea mays), Medicago (Medicago truncatula), and rice have begun to indicate where in a gravitropism pathway LAZY proteins function (Li et al., 2007; Yoshihara and Iino, 2007; Dong et al., 2013; Yoshihara et al., 2013; Ge and Chen, 2016). Statoliths appear to sediment normally in Arabidopsis and rice lazy mutants that do not form a normal gravity-induced auxin gradient or gravitropic bending response. Etiolated hypocotyls of an Arabidopsis lazy quadruple mutant (atlazy1;2;3;4) are essentially agravitropic but display a robust phototropic response (Yoshihara and Spalding, 2017), which also depends on PIN-mediated auxin redistribution (Haga and Sakai, 2013; Fankhauser and Christie, 2015). These and other results indicate that LAZY proteins function in a gravity-specific process downstream of statolith sedimentation but upstream of the auxin redistribution process.
LAZY genes are found only in embryophytes (Yoshihara et al., 2013). They encode moderate-sized proteins with no known or predictable biochemical function. Comparing LAZY gene sequences from a variety of land plants identified five short regions of conserved sequence ranging from region I at the N terminus to region V at the C terminus (Yoshihara et al., 2013). The only recognizable domain is an ethylene-responsive element binding factor-associated amphiphilic repression (EAR) motif (Yoshihara et al., 2013) that is generally associated with transcriptional repression mechanisms (Kagale et al., 2010). A well-established example is the binding of TOPLESS proteins to the EAR motif of AUX/IAA proteins, which repress auxin-responsive genes (Salehin et al., 2015; Dinesh et al., 2016). The EAR motif of a wheat LAZY protein, TaDRO1, was found to interact with a wheat TOPLESS protein (Ashraf et al., 2019).
Dardick et al. (2013) showed that region II of LAZY genes contains a GφL(A/T)GT motif, which places LAZY genes within a larger family, IGT, to which the Tiller Angle Control 1 (TAC1) gene belongs. Interestingly, TAC1 functionally opposes LAZY by increasing the tiller angle in rice (Yu et al., 2007) and branch angles in peach (Prunus persica) trees (Dardick et al., 2013) and Arabidopsis inflorescences (Waite and Dardick, 2018).
Functional fusions of LAZY proteins with GFP show they reside at the cell periphery (Li et al., 2007; Dong et al., 2013; Yoshihara et al., 2013), colocalizing with a plasma membrane resident dye (Dong et al., 2013; Yoshihara et al., 2013). In Arabidopsis, AtLAZY1 also resides in the nucleus, but mutations that prevent nuclear localization do not interfere with its branch angle-setting function (Yoshihara et al., 2013). Therefore, it is not clear whether the potential interaction between nuclear-localized auxin response factors and the EAR-like motif are relevant to the major role AtLAZY1 plays in shoot gravity responses. The C-terminal domain of AtLAZY1 also binds to microtubules (Sasaki and Yamamoto, 2015). Dong et al. (2013) showed that ZmLAZY1 binds to IAA17 in the nucleus and to a receptor-like kinase at the plasma membrane. In studies of rice, Li et al. (2019) found that OsLAZY1 interacts with a protein called OsBRXL4 at the plasma membrane. Narrower and wider tiller angles are associated with reduced OsBRXL4 expression and overexpression, respectively. More study of LAZY-interacting proteins promises to clarify how and where in cells gravity signals are converted into growth differentials.
Yoshihara et al. (2013) demonstrated that four LAZY genes in Arabidopsis possess all five conserved regions (I–V). They are AT5G14090/LAZY1, AT1G17400/LAZY2, AT1G72490/LAZY4, and AT3G27025/LAZY6. One gene, At1G19115/LAZY3, lacks region III, whereas a sixth, AT3G24750/LAZY5, possesses only region V. Studies of these genes have found some unique and some shared regions of expression in roots and shoots (Yoshihara and Spalding, 2017). Studies of knockout mutants indicate that functions of the homologous genes also overlap. For example, mutating AtLAZY1 caused a large change in branch angle, but the primary inflorescence stem remained vertical. Adding atlazy2 and atlazy4 mutations to this background caused the entire inflorescence to become prostrate (Taniguchi et al., 2017; Yoshihara and Spalding, 2017), and possibly even to reverse its preferred growth direction, as has been observed in roots of the atlazy2;3;4/atngr1;2;3 triple mutant (Ge and Chen, 2016, 2019; Yoshihara and Spalding, 2017).
It is now clear that LAZY and related genes are important mediators of gravity effects on plant architecture. In spite of the importance of the topic, the molecular mechanisms in which LAZY proteins participate remain poorly understood. The work presented here examines the functional consequences of rationally selected and engineered mutations in conserved regions of the AtLAZY1 gene. The results include the discovery of a subtle change in the AtLAZY1 protein that reverses the direction of shoot gravitropism.
RESULTS
Quantitative Transgenic Rescue of the atlazy1 Branch Angle Phenotype
The angle between the base of a branch and the primary inflorescence was measured in 134 wild-type and 141 homozygous atlazy1 plants, as shown in Figure 1A (inset). The average angle for the wild type was 39.0° ± 0.5° and 81.4° ± 0.5° for atlazy1 (Fig. 1B), similar to previous reports (Yoshihara et al., 2013). The wild type and atlazy1 data were normally distributed as determined by a Shapiro-Wilks normality test. Branch angle was also measured in atlazy1 plants transformed with the wild-type AtLAZY1 gene under the control of its native promoter (pAtLAZY1:AtLAZY1). Each T1 plant is probably the product of a unique transformation event (Desfeux et al., 2000). Thus, each of the 153 T1 plants measured may be considered an independent test of genetic rescue. The average branch angle of the rescue-test population was 54.4° ± 1.4°, but fitting two Gaussian distributions to the data produced a probability density function (PDF) that showed the population to be clearly bimodal (Fig. 1C). The Shapiro-Wilks test indicated that these rescue-test data were not normally distributed. Many of the transformants displayed a wild-type branch angle, but many displayed a mutant branch angle (Fig. 1, B and C). The substantial portion of the pAtLAZY1:AtLAZY1/atlazy1 curve covering angles >60° indicates that the AtLAZY1 genomic fragment was often not fully effective. If this was solely due to the random position of insertion in the genome, a similar PDF should be observed when a different but similarly functioning gene is used with the same promoter. When AtLAZY2 was expressed under the control of the AtLAZY1 promoter, the population of transformants was unimodal, normally distributed, and similar to the wild type (Fig. 2, A and B). This suggests that the bimodality in the control rescue-test population is not caused simply by a transgene position effect but by something specific to the AtLAZY1 coding sequence, introns, untranslated regions of the transcript, and/or the AtLAZY1 protein. These data indicate that AtLAZY2, which is 19.8% identical to AtLAZY1 at the amino acid level, as determined by the T-COFFEE sequence alignment tool (Notredame et al., 2000), can perform the AtLAZY1 branch angle-related functions.
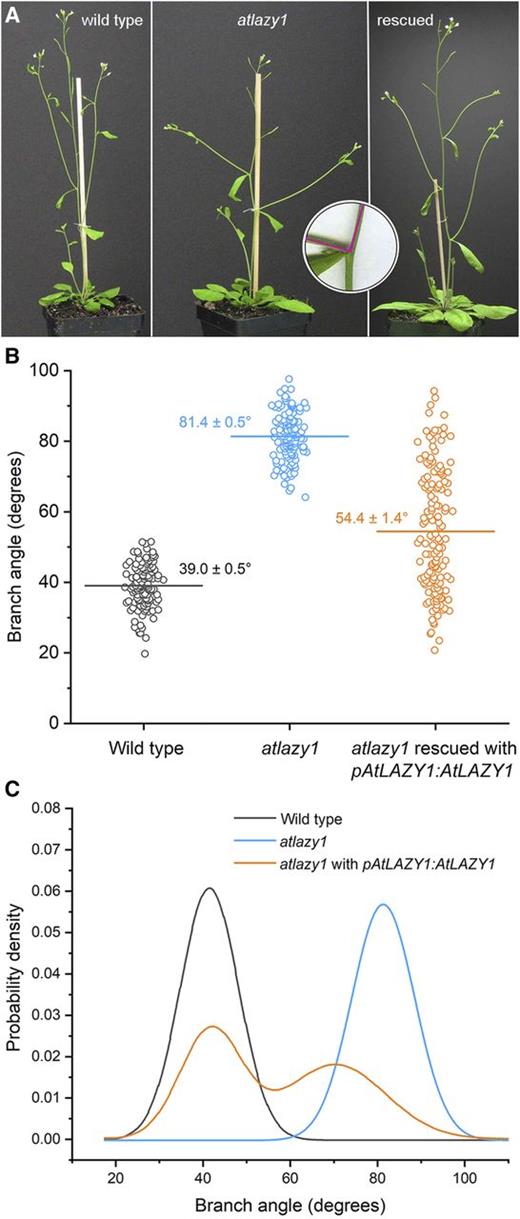
Evaluation of rescue of the atlazy1 branch angle phenotype in the T1 generation. A, Shoot architecture in the wild type, atlazy1, and atlazy1 rescued with pAtLAZY1:AtLAZY1 in the T1 generation. Shown from left are the wild type, atlazy1, and atlazy1 rescued with pAtLAZY1:AtLAZY1 in the T1 generation. The inset shows how the angle at the base of the branch was measured with two 10-mm radii centered at the intersection of a main stem and a branch. B, Distributions of branch angles measured in populations of wild type, atlazy1, and atlazy1 rescued with pAtLAZY1:AtLAZY1 in the T1 generation. The horizontal line marks the mean, which is also given (± se). The numbers of samples measured were as follows: 134 wild type, 141 atlazy1, and 153 rescued lines. C, PDFs of branch angles measured in the populations of wild-type, atlazy1, and atlazy1 plants rescued with pAtLAZY1:AtLAZY1 in the T1 generation. PDFs were obtained by fitting Gaussian distributions to the data used in B.
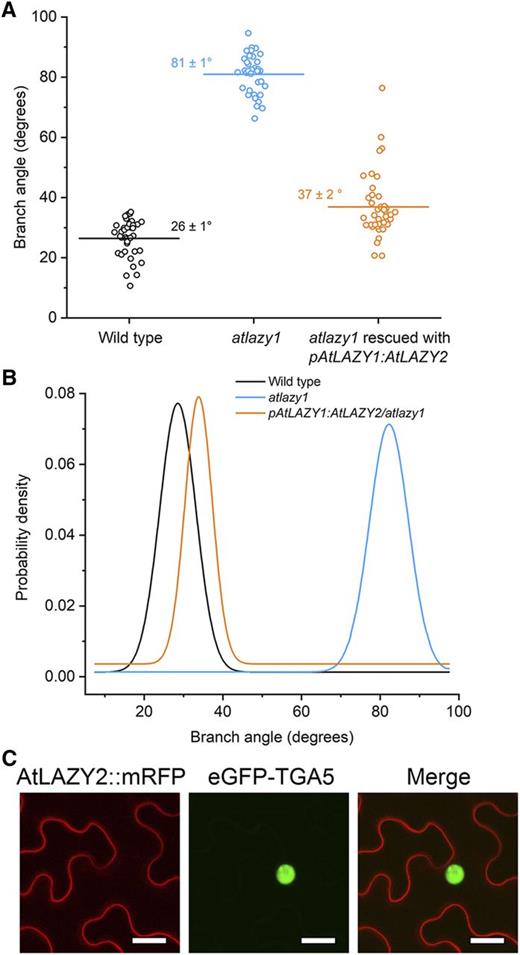
AtLAZY2 at the plasma membrane replaces AtLAZY1 function. A, Branch angles measured in populations of the wild type, atlazy1, and atlazy1 transformed with pAtLAZY1:AtLAZY2 in the T1 generation. The horizontal line marks the mean, which is also given (± se). The numbers of samples measured were as follows: 49 wild type, 60 atlazy1, and 82 atlazy1 plants rescued with pAtLAZY1:AtLAZY2. B, PDFs of branch angles shown in A. The distribution of pAtLAZY1:AtLAZY2/atlazy1 branch angles was unimodal. C, Subcellular localization of 35S:AtLAZY2-mRFP examined in Nicotiana benthamiana leaf epidermal cells. Subcellular localization of AtLAZY2-mRFP was examined by Agrobacterium tumefaciens-mediated transient expression of 35S:AtLAZY2-mRFP and 35S:TGA5-eGFP in N. benthamiana leaf epidermal cells. The red and green channels show AtLAZY2-mRFP and the TGA5-eGFP nuclear markers, respectively. Bars = 25 μm. AtLAZY2-mRFP localized to the plasma membrane and not the nucleus.
AtLAZY1 is present at the plasma membrane and the nucleus of cells (Yoshihara et al., 2013). Mutation of the internal nuclear localization signal from AtLAZY1 eliminated nuclear accumulation of the protein but did not affect its ability to rescue the atlazy1 branch angle phenotype (Yoshihara et al., 2013). AtLAZY2 is found only at the plasma membrane (Fig. 2C). Thus, rescue of the atlazy1 branch angle phenotype appears not to require accumulation of an AtLAZY protein in the nucleus.
The results in Figures 1 and 2 establish a method for structure-function analysis of the AtLAZY1 protein that is based on (1) the AtLAZY1 promoter, (2) an atlazy1 knockout mutant, (3) large numbers of independently transformed plants, and (4) branch angle PDFs to quantify the effectiveness of the transgene modification.
Structure-Function Analysis of the Five LAZY Conserved Domains
Five short regions of sequence are conserved among LAZY genes from diverse land plants (Yoshihara et al., 2013). Figure 3A shows the locations of conserved regions I–V in the 358-amino acid protein predicted from the AtLAZY1 gene sequence. The functional significance of these conserved regions in AtLAZY1 was investigated by altering one or more of the most invariant amino acids in each and transforming atlazy1 plants with the mutated versions controlled by the same AtLAZY1 promoter used to produce the transgenic plant data in Figures 1 and 2. To determine whether the site-directed mutagenesis prevented expression of the protein in the plant, a GFP-tagged version of each variant controlled by a strong heat shock promoter was visualized with confocal fluorescence microscopy in etiolated Arabidopsis hypocotyls. This method was necessary because the natural AtLAZY1 expression level is too low to be reliably detected with a fluorescence protein tag. To test the effect of the mutations on subcellular localization, a GFP-tagged version was transiently overexpressed in Nicotiana benthamiana leaves and then examined by confocal fluorescence microscopy. Representative examples of subcellular localization are shown in Figure 3, and more examples are shown in Supplemental Figure S1. Figure 3B shows that AtLAZY1 produced by atlazy1 plants transformed with pAtLAZY1:AtLAZY1 was apparently produced and localized to the plasma membrane and nucleus consistent with previous reports (Yoshihara et al., 2013; Taniguchi et al., 2017). Figure 3B also shows that the atlazy1 branch-angle phenotype was rescued by pAtLAZY1:AtLAZY1 to varying degrees (data replotted from Fig. 1, B and C). These results serve as the control for the following structure-function studies.
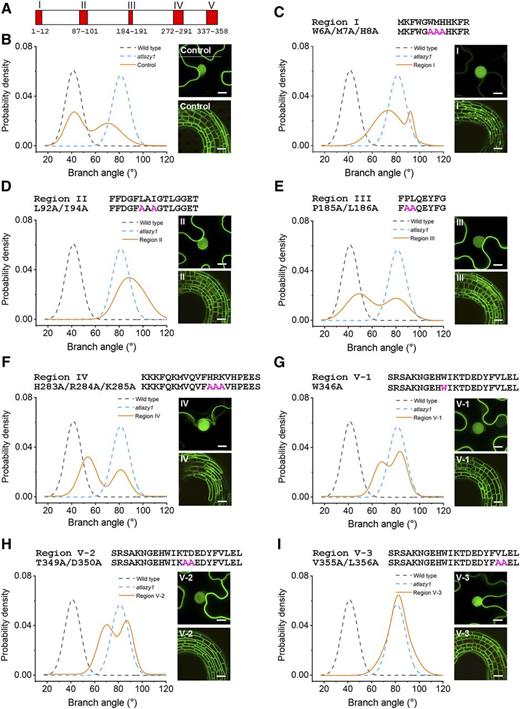
Mutagenesis of AtLAZY1 conserved regions: effects on branch angle and subcellular localization. A, Schematic showing amino acid positions of the five AtLAZY1 protein regions (AT5G14090 gene model) conserved among homologs, as defined by Yoshihara et al. (2013). B to I, Effects of mutagenesis on the function of AtLAZY1 and its subcellular localization. The functions of the engineered variants were evaluated as shown in Figure 1. Selected conserved amino acid sequences were replaced with alanines by site-directed mutagenesis of pAtLAZY1:AtLAZY1, and the engineered variants were expressed in an atlazy1 knockout background. Shown for each variant are the amino acid sequence of the conserved region, the altered sequence in magenta and in letter/number code, the PDFs derived from the branch angle measurements, the subcellular localization in Nicotiana benthamiana epidermal cells (top right), and expression in Arabidopsis hypocotyls (bottom right). PDFs of the wild type and atlazy1 are represented as dashed lines. Orange lines show the PDFs of the T1 population expressing the variants. The data in B are taken from Figure 1C. The data in B, D, and F did not pass a Shapiro-Wilks test of normality and are considered bimodal. The numbers of samples ranged from 134 for the wild type to 170 for the V-1 population. The effects of conserved regions on subcellular localization were examined by transiently expressing the variants in N. benthamiana leaf epidermal cells and in stably transformed Arabidopsis seedlings. Bars = 10 μm (for N. benthamiana) and 40 μm (for Arabidopsis).
Invariant and highly conserved residues within regions I–V that were identified by comparing LAZY genes from several species (Yoshihara et al., 2013) were subjected to site-directed mutagenesis. The targeted amino acids were selected to test the function of each of the conserved regions. The N-terminal region I was perturbed by changing amino acids 6–8 from Trp-Met-His to Ala-Ala-Ala, or W6A/M7A/H8A (Fig. 3C). This change to region I greatly diminished the gene’s ability to rescue the atlazy1 branch angle defect, as indicated by comparing the branch angle PDFs in Figure 3, B and C (see Supplemental Fig. S2 for a scatter plot of each branch angle value from which the PDFs were derived). The W6A/M7A/H8A change to region I also reduced the protein’s presence at the plasma membrane without affecting the nuclear pool (Fig. 3C; Supplemental Fig. S1). This result indicates that the N terminus is required for plasma membrane localization and branch-angle function, consistent with previous findings (Fig. 2; Yoshihara et al., 2013).
To perturb region II, a three-amino acid sequence was changed from Leu-Ala-Ile to Ala-Ala-Ala, or L92A/I94A (Fig. 3D). This change did not alter the subcellular localization pattern of the protein but it completely prevented the protein from rescuing the branch angle defect (Fig. 3D). In fact, a substantial number of the branch angles were >90°, i.e. were oriented downward. The notably unimodal function was shifted to greater angles compared to the atlazy1 background in which it had been placed. This two-amino acid variation of the protein it replaced apparently caused a phenotype more severe than complete loss of function. Two plants that showed greater branch angles than atlazy1 were selected from the population of T1 plants expressing pAtLAZY1:AtLAZY1L92A/I94A. Two lines homozygous for the transgene and homozygous for the atlazy1 background mutation were obtained and named L1 and L2. The average branch angles of these homozygous lines bearing a two-amino acid variation of AtLAZY1 in an atlazy1 knockout background were shifted even further downward, ∼30° below the horizontal (Supplemental Fig. S3). The branch angles in these lines were shifted downward even more than that of an essentially agravitropic atlazy1;2;3;4 quadruple mutant (Supplemental Fig. S3), which produces a prostrate inflorescence similar to that of the atlazy1;2;4 mutant described previously (Yoshihara and Spalding, 2017).
The variants with mutations in region III (P185A/L186A) or region IV (H283A/R284A/K285A) rescued the atlazy1 branch angle defect slightly less well than the control construct and showed typical wild-type subcellular localization (Fig. 3, E and F). These data sets appeared bimodal and did not pass a Shapiro-Wilks test of normality. Regions III and IV are not as highly conserved as the others (Yoshihara et al., 2013). Perhaps in certain environmental conditions regions III and IV would be found to have a more significant impact on aspects of shoot architecture that gravity influences.
Region V, a signature motif of LAZY proteins (Yoshihara et al., 2013), is the longest conserved region, and therefore, three distinct variants (W346A, T349A/D350A, and V355A/L356A) were created to test its role in setting branch angles. None of these changes affected production of the protein in Arabidopsis or its subcellular localization in N. benthamiana leaf cells (Fig. 3, G–I–I). The first two (variants V-1 and V-2) were markedly less able to rescue the atlazy1 branch angle defect. The third (V-3) showed no rescue ability. Thus, region V is critical to the AtLAZY1 branch angle function.
Only the W6A/M7A/H8A alteration in region I had a qualitative effect on subcellular localization (Fig. 3C). It appeared to eliminate location at the plasma membrane, and it lacked branch angle-setting function. Region I probably cannot anchor the AtLAZY1 protein to the membrane by itself because region I fused to enhanced GFP (eGFP) appeared to remain in the cytoplasm, similar to the free-eGFP control (Supplemental Fig. S4).
The structure-function results indicate that regions I, II, and V play a critical role in determining lateral branch angle. Region II mutations created a special effect that was further investigated.
A Two-Amino Acid Change to Region II Reverses Gravitropism
The downward orientation of branches in pAtLAZY1:AtLAZY1L92A/I94A/atlazy1 T1 plants (hereafter L92A/I94A) may be the result of a reversal in the normal negative gravitropism mechanism. To test this, plants were grown in a normal orientation illuminated from above (Fig. 4A), or upside down and illuminated from below (Fig. 4B). The wild-type primary inflorescence stem and its branches exhibited strong negative gravitropism (upward bending). Regardless of the imposed orientation, atlazy1 inflorescence branches emerged from the main axis at a near-horizontal angle. By contrast, the stem, branches, and even pedicels subtending the flowers of the L92A/I94A plants aligned positively (downward) with the gravity vector whether grown right-side up or upside down. The interpretation that wild-type negative gravitropism was weakened by the loss of AtLAZY1 and switched to a positive orientation by the L92A/I94A alteration was tested by rotating normally grown plants by 90°. Shoots of L92A/I94A responded with a phase of normal (negative) gravitropism that was followed by bending in the opposite direction (positive or reverse gravitropism) to bring the apex near to the original, horizontal position (Fig. 4C). A possible complication to address was that sagging due to a mechanical weakness of L92A/I94A shoots contributed to the appearance of reverse, positive gravitropism. Results of double reorientation assays argued against this possibility. When wild-type plants were inverted, the primary inflorescence and lateral branches curved to become upright and when they were returned to their original orientation, the shoots reversed the direction of new growth to become upright again (Supplemental Fig. S5). L92A/I94A branches grown upside down did not sag when returned to the normal orientation. Their new growth was distinctly downward, displaying reverse (positive) gravitropism (Supplemental Fig. S5).
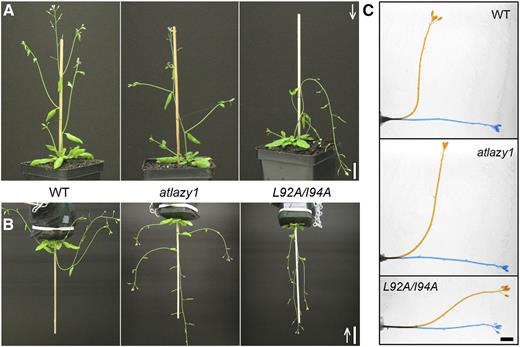
Downward orientation of inflorescence stem caused by mutating region II (L92A/I94A). A, Shoot system architecture of wild-type (WT), atlazy1, and pAtLAZY1:AtLAZY1L92A/I94A/atlazy1 (L92A/I94A) plants grown in a normal orientation for 4 weeks with light supplied from above, as shown by the white arrow. Bar = 2 cm. B, Lateral organ orientations in wild-type, atlazy1, and L92A/I94A plants grown upside-down after their branches reached ∼1–3 cm with light supplied from below (see arrow). Note that branches and pedicels oriented horizontally in atlazy1 and downward in pAtLAZY1:AtLAZY1L92A/I94A/atlazy1, though tips of shoots are oriented toward the light source in both cases. Bar = 3 cm. C, Gravitropism in excised wild-type, atlazy1, and L92A/I94A inflorescence stems. Images taken immediately after 90° rotation (0 h; blue) and 18 h after rotation (orange) were superimposed. Bar = 5 mm.
The possibility that mechanical weakness contributed wholly or in part to the apparent reverse gravitropism of L92A/I94A inflorescences was directly tested with an electronic force transducer. The experiment was designed to measure the stiffness of primary inflorescence stems that were at least 22 cm long, a point in development when the apex of L92A/I94A inflorescences, grown with support as shown in Figure 4A, clearly displayed downward growth. The custom device measured the force required to flex the free end of a stem segment by 2 mm, as shown in Figure 5, A and B. The method accurately quantified the difference in wild-type stem stiffness between the apical 3 cm (upper stem) and 7 cm below the apex (lower stem; Fig. 5C). The results obtained with the homozygous region II variants (L1 and L2) were not significantly different from the wild type, or from the essentially prostrate atlazy1;2;3;4 quadruple mutant. The results in Supplemental Figure S5 and Figure 5C indicate that differences in mechanical strength of the stems do not explain the downward orientation of shoots homozygous for a region-II variation of AtLAZY1. Instead, the evidence indicates that the subtle L92A/I94A variation in the AtLAZY1 protein reversed the gravitropic response.
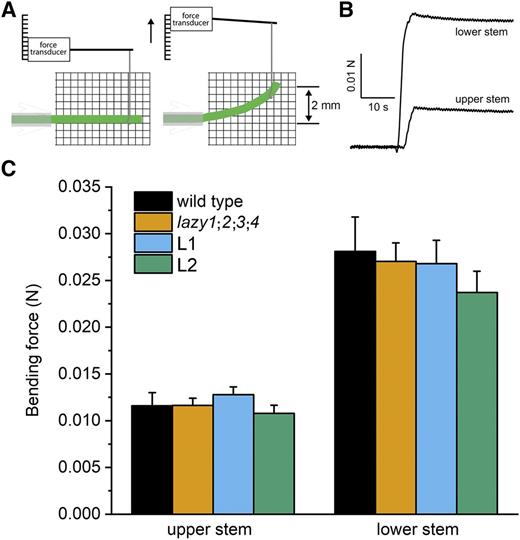
Mechanical strength of inflorescence stems. A, Diagram of the apparatus and bending stem segment. B, The force required to flex stem segments excised ∼3 cm (upper stem) and 7 cm (lower stem) from the shoot apical meristem was measured with an electronic force transducer. C, The mean force (± se) obtained from 6–14 separate segments for each position and genotype are plotted. L1 and L2 refer to independent transgenic lines that are homozygous for the L92A/I94A variation in region II and homozygous for the atlazy1 knockout mutation. The genotype means in each position were not significantly different as determined by two-way ANOVA.
The reverse gravitropism explanation was tested by measuring the reorientation time course of primary inflorescences following a 90° rotation. The apical 7 cm of the primary inflorescence was excised from plants at developmental stages before (7–10 cm) or after (22–25 cm) the point when L92A/I94A shoots display downward growth. With the cut ends maintained in a nutrient solution, excised wild-type inflorescences of either developmental age rapidly reoriented, overshot 90°, and attained a steady vertical orientation within 6 h (Fig. 6A; Supplemental Video S1). The younger L1, L2, atlazy1, and wild-type stems responded similarly, except that the overshoot phase was dampened in the mutants (Fig. 6A). However, the older L1 and L2 stem segments displayed a normal (negative) gravitropism response until an angle of ∼80° was achieved 3 h after rotation. Thereafter, by approximately the time a wild-type stem would have reached 90°, the L1 and L2 stems began to develop reverse (positive) gravitropism (Fig. 6A), which continued over the next several hours until the horizontal (0°) was approached. The atlazy1;2;3;4 quadruple mutant response, representing a largely agravitropic control, was similar to the gravitropic response Taniguchi et al. (2017) reported for a triple mutant (lzy1;lzy2;lzy3) that is equivalent to the atlazy1;2;4 mutant Yoshihara and Spalding (2017) characterized. These and other results indicate that AtLAZY1/LZY1, AtLAZY2/LZY2, and AtLAZY4/LZY3 are the major contributors to the inflorescence gravitropism response (Taniguchi et al., 2017; Yoshihara and Spalding, 2017).
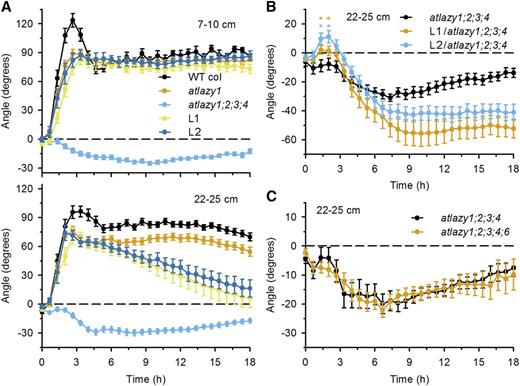
Gravitropism time courses. A, Gravitropic responses of segments excised from young inflorescences (7–10 cm long) or older inflorescences (22–25 cm long). Two lines homozygous for the pAtLAZY1:AtLAZY1L92A/I94A transgene in the atlazy1 knockout background (L1 and L2) and the atlazy1;2;3;4 quadruple mutant were measured. The effect of the L92A/I94A variant became clear in the older stems. The initial bending response reversed over hours. The means ± se of >21 trials per genotype and age are shown. WT, Wild type. B, The pAtLAZY1:AtLAZY1L92A/I94A transgene caused downward bending in atlazy1;2;3;4. The atlazy1 background of the L1 and L2 lines was replaced with atlazy1;2;3;4 for this experiment, which was conducted with stems excised from 22- to 25-cm inflorescences. The means ± se of >17 samples per genotype are shown. Asterisks mark time points that are significantly different between atlazy1;2;3;4 and L1/atlazy1;2;3;4 or between atlazy1;2;3;4 and L2/atlazy1;2;3;4 (*P < 0.05). C, Gravitropism of atlazy1;2;3;4;6 inflorescence stems (22–25 cm long) compared to the response of atlazy1;2;3;4 stems identified no role for the AtLAZY6 gene. The mean ± se obtained for >17 samples per genotype is shown.
The finding that a normal (upward bending) AtLAZY-dependent response fails to be maintained in L1 and L2 (Fig. 6A) raises the possibility that the L92A/I94A modification to AtLAZY1 impairs the function of other contributing AtLAZY proteins. To explore this possibility, crosses were performed to place the pAtLAZY1:AtLAZY1L92A/I94A in an atlazy1;2;3;4 background. The L1/atlazy1;2;3;4 and L2/atlazy1;2;3;4 lines were produced. They both showed substantially stronger reverse gravitropism than atlazy1;2;3;4 (Fig. 6B; Supplemental Video S2). Thus, impairment of other AtLAZY homologs does not explain how the L92A/I94A modification to AtLAZY1 switched the direction of stem gravitropism. The genetic analysis was expanded to include AtLAZY6, for which limited knowledge is currently available. The atlazy1;2;3;4;6 quintuple mutant, which lacks all AtLAZY family function except the aberrant AtLAZY5, did not display L92A/I94A-like reverse gravitropism (Fig. 6C). Instead of 40° to 60° of downward bending in stems possessing the region II L92A/I94A variant, the quadruple and quintuple mutants, which probably lack all LAZY gene functions, only transiently exceeded 20° of reverse bending. These results argue against the possibility that the L92A/I94A effects on branch angle and gravitropism are due to interference with other AtLAZY proteins.
The robustness of the reverse gravitropism caused by the region II variation was explored with a double-rotation experiment. Excised inflorescences from plants at least 22 cm tall were rotated by 90° clockwise for 4 h, which is the point in time at which downward, reverse gravitropism initiates (Fig. 6B). Then the stems were rotated a further 90° clockwise (a total of 180°). The apices of L1/atlazy1;2;3;4 and L2/atlazy1;2;3;4 stems, now upside down, curved in the clockwise direction for another 2 h. The tips deflected ∼30° from the vertical, which would not have occurred if the downward bending had been the consequence of sagging instead of reverse gravitropism (Supplemental Fig. S6). The atlazy1;2;3;4 quadruple mutant responded only weakly, consistent with its weak reverse gravitropism in response to a single rotation (Fig. 6B). These results (Fig. 6; Supplemental Fig. S6) demonstrate that replacing AtLAZY1 with AtLAZY1L92A/I94A reverses the gravity response of the inflorescence stem, which is a profound alteration.
The L92A/I94A Change Reverses the Auxin Gradient, But Does Not Affect Auxin Responsiveness
A conservative alteration to the AtLAZY1 sequence (L92A/I94A) reversed the behavior of the shoot. Based on how gravitropism is understood to occur, two distinctly different explanations seemed possible. One would depend on the auxin response mechanism being inverted. In this case, low auxin would promote growth and high auxin would inhibit growth. Alternatively, the auxin gradient that causes differential growth across the organ could be reversed. In this scenario, higher levels of auxin would accumulate on the upper flank rather than the lower flank of a branch, resulting in downward bending. To determine whether reversed auxin action or a reversed auxin gradient explains the behavior of L92A/I94A plants, auxin was applied to one side of growing inflorescence stems. The result was unequivocal. Stems of wild-type, atlazy1, and L1 (a line homozygous for both the pAtLAZY1:AtLAZY1L92A/I94A transgene and the atlazy1 knockout mutation) plants responded similarly by bending away from the applied auxin over a range of concentrations (Fig. 7). No switch in auxin responsiveness resulted from the L92A/I94A alteration, which makes a reversed auxin gradient the expected cause of the downward bending and reverse gravitropism of L92A/I94A plants.
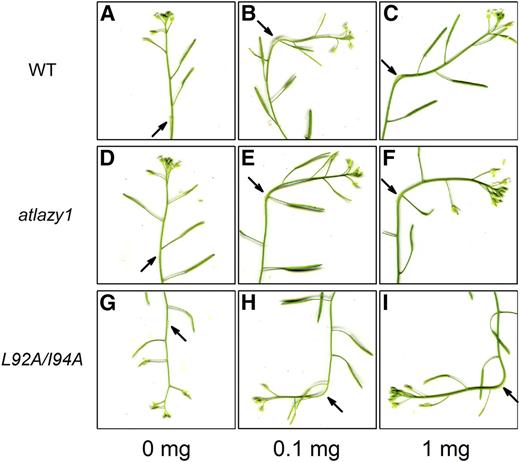
Normal growth response to auxin in L92A/I94A stems that display reverse gravitropism. Lanoline paste containing the indicated concentrations of auxin was applied to a flank of inflorescence stems and images were captured 18 h later. A to C, Wild type (WT). d to F, atlazy1. G to I, pAtLAZY1:AtLAZY1L92A/L94A/atlazy1.
To test the reversed auxin gradient hypothesis, the expression level of an auxin-responsive gene was measured in the upper and lower halves of inflorescence stems by reverse-transcription quantitative PCR (RT-qPCR). IAA5 was previously shown to be a good indicator of the gravity-induced auxin gradient in inflorescence stems (Taniguchi et al., 2014). L1/atlazy1:2;3;4 and L2/atlazy1;2;3;4, which showed pronounced reverse gravitropism (Fig. 6B), were used in this experimentation. IAA5 expression was measured at 1 and 3 h after rotation. Wild-type inflorescence stems showed an approximately 9-fold increase of IAA5 expression in the lower half of stems 1 h after rotation (Fig. 8A), as shown previously (Taniguchi et al., 2014, 2017). Unexpectedly, the basal expression of IAA5 (expression at 0 h) was much lower in atlazy1;2;3;4, L1/atlazy1;2;3;4, and L2/atlazy1;2;3;4 stems than in the wild type (Fig. 8A). Supplemental Figure S7 shows that the assay was sensitive enough to reproducibly measure the 60%- to 80%-lower levels of IAA5 expression in the mutants, so a normalization approach was taken to compare the gravity-induced expression gradients. The analysis showed that IAA5 expression was higher in the upper side of the inflorescence stems of L1/atlazy1;2;3;4, and L2/atlazy1;2;3;4 plants at 3 h (Fig. 8B), the time at which reverse gravitropism started to become evident (Fig. 6B). The reverse auxin gradient did not develop in atlazy1;2;3;4 stems, and they did not display reverse gravitropism. Therefore, the auxin gradient through which gravity naturally directs growth, diminished by loss of AtLAZY1, is reversed by the L92A/I94A alteration of the AtLAZY1 protein.
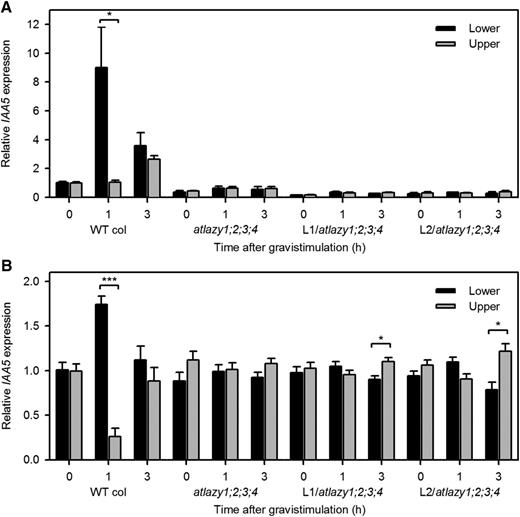
Expression of an auxin-responsive gene, IAA5, in the upper and lower halves of inflorescence stems measures the gravity-induced auxin gradient. A, A PCR-based method quantified the levels of IAA5 in the upper and lower halves of inflorescence stems after the indicated period of gravistimulation. The values were divided by the average of the wild-type (WT) upper and lower pools at 0 h. Shown are the mean ± se obtained from three biological replicates, each measured three times. An asterisk indicates a statistically significant difference (*P < 0.05). Note that basal expression level of IAA5 was lower in the mutants. B, To highlight the gradient rather than the absolute expression level, the mean expression of IAA5 between the lower and upper halves was normalized to 1. Statistically significant differences in IAA5 expression between lower and upper halves were observed in the wild type and reversed gradients were observed in the two homozygous lines, which also displayed strong reverse gravitropism. Student’s t test. (***P < 0.001, *P < 0.05).
DISCUSSION
The PDFs derived from branch angle measurements made on many T1-generation plants expressing AtLAZY1 variants were more useful than the mean value. They described the rescue ability of each AtLAZY1 variant more comprehensively than the fairly common practice of showing a representative outcome which may be difficult to objectively select. Our methodology revealed an intriguing bimodal distribution when AtLAZY1 was expressed under the control of its own promoter in an atlazy1 knockout mutant. Perhaps expression level varied between T1 plants and AtLAZY1 performs its function only when expressed above a threshold level. Perhaps some mRNA processing or posttranslational modification is triggered in some plants but not others. Whatever causes the bimodality, it clearly does not apply to the functionally related AtLAZY2 gene.
Consistent with previous results (Yoshihara et al., 2013), Figure 3C shows that loss of AtLAZY1 at the plasma membrane correlated with loss of branch-angle function. How AtLAZY1 associates with the plasma membrane, where it appears to function (Fig. 3; Yoshihara et al., 2013; Taniguchi et al., 2017), is not clear. It apparently lacks a membrane-spanning domain. Lipid modification of the protein is a possible explanation, because the GPS-Lipid program developed by Xie et al. (2016) identified the Gly at the fifth position in AtLAZY1 as a possible nonconsensus N-myristoylation site. Figure 3C shows that mutating the adjacent three amino acids greatly reduced plasma membrane association and function. Both AtLAZY2/LZY2 and AtLAZY4/LZY3 are plasma membrane localized and both have a Gly at the fifth position like the possible myristoylation site in AtLAZY1/LZY1. Arguing against this mechanism of membrane attachment is the fact that an N-terminal fragment of AtLAZY1 fused to eGFP was not associated with the plasma membrane (Supplemental Fig. S4). Future work should investigate the possibility that at least some AtLAZY proteins link to the plasma membrane via a posttranslational lipid modification, which probably involves shortening the N terminus to make the Gly the second position (Hentschel et al., 2016).
The role of nuclear-localized AtLAZY1 requires more investigation. AtLAZY1 in the nucleus appeared to be ineffective (Fig. 3C; Yoshihara et al., 2013); however, in rice, nuclear-localized OsLAZY1 appears to influence tiller angle (Li et al., 2019). The mechanism of LAZY action may differ between species and organs. Even its association with the plasma membrane may have a different molecular basis in different species. Some sequence-analysis tools find evidence for a single membrane-spanning domain in rice and maize LAZY1 (Li et al., 2007; Dong et al., 2013). Membrane-spanning domains are not predicted in AtLAZY1 or its Arabidopsis homologs.
AtLAZY1 may associate with the plasma membrane not by possessing a transmembrane span or by myristoylation but by binding to a membrane protein or complex. In support of this possibility, Li et al. (2019) reported that rice LAZY1 interacts with a protein called OsBRXL4 to sequester OsLAZY1 at the plasma membrane and therefore away from its site of action in the nucleus. Another protein reported to interact with a LAZY1 protein at the plasma membrane is a maize kinase (Dong et al., 2013). The interacting maize kinase is related to the Arabidopsis PINOID kinase that participates in directing auxin transport by affecting the localization of PIN proteins (Michniewicz et al., 2007; Huang et al., 2010; Ganguly et al., 2012, 2014). An attractive hypothesis is that AtLAZY1 functions in a protein complex at the plasma membrane that couples the presence of settled statoliths with the mechanism that determines the localization of PIN auxin efflux regulators, especially PIN3. Somehow, in this scenario, the L92A/I94A variant of AtLAZY1 reverses the output of the mechanism such that the PIN localization, and therefore the auxin gradient, is reversed. Direct tests of this idea have been hampered by the difficulty of visualizing fluorescently tagged proteins in the endodermal cells near the center of thick, heavily pigmented stems.
Altering two amino acids in the IGT domain in region II of AtLAZY1 (L92A/I94A) reversed the gravitropic response of the inflorescence stem (Fig. 6B) and produced a weeping phenotype in the adult plant (Fig. 4A). The phenomenon of reverse gravitropism due to mutations of LAZY genes has been reported previously in roots (Ge and Chen, 2016; Yoshihara and Spalding, 2017). Taniguchi et al. (2017) showed that a C-terminal (region V) portion of AtLAZY2/LZY2 fused with mCherry causes upward growth of roots, especially when expressed under the control of a LZY2/AtLAZY2 promoter in an atlazy1;2;4/lzy1;2;3 background. It is not clear whether homologous mechanisms are responsible for the previously reported examples of reverse root gravitropism and the reverse shoot gravitropism in L92A/I94A. The time-course analysis in Figure 6B shows that reverse shoot gravitropism began after a transient phase of negative (normal) gravitropism. A transient phase of normal, positive gravitropism did not precede the reverse gravitropism in atlazy2;3;4/atngr1;2;3 roots (Ge and Chen, 2016; Yoshihara and Spalding, 2017). The time course of the reverse gravitropism caused by the region V LZY2-mCherry (CCL-mCherry) protein was not reported (Taniguchi et al., 2017), so it cannot be compared to the present shoot version of the phenomenon.
Gravitropism consists of a bending phase in which an auxin gradient produces curvature and a subsequent straightening phase during which the growth differential must reverse to produce an upright stem. A mathematical model developed by Bastien et al. (2013) successfully simulates gravitropism in the stems of many species by having the straightening phase be a consequence of a proprioceptive process sensitive to the bending produced by the first phase. Rakusová et al. (2016) present evidence obtained with Arabidopsis hypocotyls indicating that the PIN3 auxin-efflux protein initially moves to the lower cell side after gravitropic stimulation to direct auxin to the lower side of the hypocotyl to cause the upward bending phase. Then, in response to the increased auxin concentration, PIN3 moves to the opposite cell side to dissipate the auxin gradient, terminate gravitropic bending, and produce a straightening phase (Rakusová et al., 2016). Genetic and pharmacological results indicate that the cytoskeleton plays a role in the PIN repositioning and possible proprioception required for the straightening response (Rakusová et al., 2011, 2019; Okamoto et al., 2015). The region II modification (L92A/I94A) may have impaired the first phase and/or strengthened the second phase, resulting in an overall reverse response (Fig. 6B) by directly or indirectly altering communication with the cytoskeleton.
The results reported here may also pertain to root gravitropism, which relies mostly on the AtLAZY2/LZY2/NGR1, AtLAZY3/LZY4/NGR3, and AtLAZY4/LZY3/NGR2 genes and PIN3 redistribution (Taniguchi et al., 2017; Ge and Chen, 2019). If mutations equivalent to L92A/I94A in these genes reverses root gravitropism, then perturbations of region II may be a powerful tool for studying the fundamental basis of gravity sensing, which was critical to the success of embryophytes after the colonization of land.
MATERIALS AND METHODS
Plant Materials and Growth Conditions
Ecotype Col-0 of Arabidopsis (Arabidopsis thaliana), obtained from the European Arabidopsis Stock Centre, was the wild type used in all experiments. The transfer DNA insertion mutants in AtLAZY genes were obtained as described in Yoshihara and Spalding (2017). To test the ability of pAtLAZY1: AtLAZY1 and its variants to rescue atlazy1, T1 seeds were surface sterilized and sown on Murashige and Skoog (MS) plates (0.05% [w/v] MES and 0.7% [w/v] agarose, pH 5.7 with KOH) supplemented with Suc (1% [w/v]) containing BASTA (7.5 μg/mL). Healthy seedlings were transplanted to pots containing a commercial soil mixture (Fafard 3B mix, Sungro Horticulture) ∼2 weeks after planting, then grown for 4–6 weeks in a growth chamber (22°C, ∼90 µmol m−2 s−1 of white light, 16-h light/8-h dark cycles).
Branch Angle Measurements
Lateral branch angles were measured as described previously (Yoshihara et al., 2013) with the following minor changes. Images of stems were obtained with a flatbed scanner. Branch angles were determined from the images by centering a 10-mm circle at the center of the node and drawing a straight line that bisected the region of the branch that intersected the circle. The angle between this branch line and a radial line that best bisected the primary stem was measured using ImageJ software (Schneider et al., 2012). The PDFs were determined by fitting two Gaussian distributions to a frequency histogram of the angle measurements for each genotype using the Origin 2018b software package (OriginLab Corp.). Dividing the cumulative fitted Gaussian curve by the number of observations and the bin width produced the displayed plots. The area under each curve equals 1. The Shapiro-Wilks test of normality was performed on each of the data sets in Figure 3 using the Origin 2018b software package and a P-value of 0.05.
Stem Bending Force Measurement
Primary inflorescences 22–25 cm long were used for measurements of bending force. Two 3-cm-long segments were excised from each primary inflorescence. The apical end of one segment was a point 3 cm below the apex and that of the other was a point 7 cm below the apex, so the “upper stem” segment represented 3–6 cm and the “lower stem” represented 7–10 cm from the tip. The basal end of the stem segment was inserted into a clamped, horizontal glass capillary tube (1.5-mm inner diameter) such that ∼1 cm of the apical portion was exposed. Cotton thread was tied in a knot around the segment ∼3 mm from the apical end to prevent a wire hook that was connected to an overhead force transducer from slipping off the segment as the transducer was slowly lifted with the aid of a manual micromanipulator. A computer-controlled charge-coupled device camera and macro zoom lens allowed the experimenter to observe when the free end of the segment had been displaced upward by 2 mm as judged with the aid of a graph paper background in the scene. The voltage output of the transducer, which is proportional to force, was measured at 20 Hz during the lift with a custom digital acquisition platform similar to that described previously (Spalding, 1995). The difference between the starting (free hanging) voltage and the steady state voltage after lifting the free end by 2 mm was determined and converted to Newtons using a linear calibration curved constructed with known masses.
Inflorescence Gravitropism
Gravitropism of inflorescence stems was measured as described in Toyota et al. (2013) with some modifications. The cut end of a 7-cm section excised from the apex of an inflorescence stem was placed in a 0.2-mL PCR tube containing half-strength MS media supplemented with 0.5% (w/v) Suc. The stem section was secured in the tube by wrapping the base with Parafilm M (Pechiney Plastic Packaging). The tubes with stems were maintained upright in a custom rack to recover for 2 h in dim red light (4–6 µmol m−2 s−1) produced by light-emitting diodes having a peak output at 660 nm. The tube rack with stems was rotated by 90° while a charge-coupled device camera (Marlin; Allied Vision Technologies), with its internal infrared filter removed and outfitted with an 8-mm lens (M0814-MP; Computar) fronted with an infrared longpass filter made from infrared-transmitting, visibly opaque plexiglass (ACRY11460; Ridout Plastics) acquired images. The sample was backlit with infrared light-emitting diodes having a peak output at 940 nm. The cameras were programmed to collect an image every 30 min for 18 h. Angle measurements were made manually from the time series of images using ImageJ software.
Plasmid Construction
All primers and DNA oligomers used in this study were synthesized by Integrated DNA Technologies and listed in Supplemental Table S1. Promoters and genes were amplified from genomic DNA by PCR and the resulting fragments were subcloned into pGEM-T-Easy (Promega) for sequence confirmation before proceeding.
For the rescuing tests of the atlazy1 branch angle phenotype, pAtLAZY1:AtLAZY1 was constructed. A wild-type genomic fragment of AtLAZY1 including 1.9 kb of the promoter region was amplified with a Pac I site at the 5′ end and a BamH I site at 3′ end. These amplified fragments were subcloned and recloned between the Pac I/BamH I sites of the binary vector pEGAD (Cutler et al., 2000). The GV3101 strain of Agrobacterium tumefaciens was transformed with the binary vector. Arabidopsis plants were transformed using the floral dip method (Clough and Bent, 1998). The Phusion site-directed mutagenesis kit (Finnzymes) was used to introduce mutations in the conserved regions. The subcloned fragments of genomic DNA of AtLAZY1 in pGEM-T-Easy obtained as stated above were subjected to site-directed mutagenesis and recloned into pEGAD after sequence confirmation.
For a promoter swapping construct, pAtLAZY1:AtLAZY2, the wild-type genomic fragment (1.9 kb) of the promoter region of AtLAZY1, was amplified with the Sma I site at the 5′ end and the wild-type genomic fragment (1.8 kb) of AtLAZY2 (At1g17400) from start codon to 3′ untranslated region (UTR) was amplified with BamH I site at the 3′ end. The AtLAZY1 promoter was fused with the AtLAZY2 coding region by the overlap-extension PCR method (Ho et al., 1989). The resulting fragment was subcloned and recloned between the Pac I/BamH I sites in the binary vector pEGAD.
To observe the effects of changes to AtLAZY1 on expression and localization in Arabidopsis, the previously described pHSP18.2:AtLAZY1-eGFP in pGEM-T-Easy (Yoshihara et al., 2013) was used. The subcloned pHSP18.2:AtLAZY1-eGFP was subjected to site-directed mutagenesis as described above and recloned between Pac I/BamH I sites in the binary vector pEGAD.
Transient expression in Nicotiana benthamiana leaf epidermal cells was used to investigate the subcellular localization of wild-type and mutated AtLAZY1, wild-type AtLAZY2, the TGA5 nuclear marker, and an N-terminal portion of AtLAZY1. The 35S:AtLAZY2-mRFP construct was generated by three-step PCR (Grandori et al., 1997). The entire AtLAZY2 genomic sequence from the start codon to the 3′ UTR and an mRFP sequence were amplified using the primers in Supplemental Table S1. The mRFP sequence was obtained from the pSAT6-RFP-C1 vector (Tzfira et al., 2005). These amplified fragments were subcloned and combined into the Pac I/BamH I site of pEGAD. To express TGA5 as a nuclear marker in N. benthamiana leaf epidermal cells, 35S:eGFP-TGA5 was constructed. TGA5 was amplified with the Sma I and BamH I sites and subcloned. The Sma I–BamH I fragment of TGA5 was recloned between the Sma I/BamH I sites of pEGAD. The N-terminal part of AtLAZY1 containing region I was fused with eGFP to see the effect of the N-terminal part on AtLAZY1 localization. Oligonucleotides coding N-terminal parts of AtLAZY1 were annealed to make double-stranded DNA and digested with Age I and recloned into the Age I site of pEGAD to obtain the constructs N-terminal-AtLAZY1:eGFP.
An atlazy6 mutant was created using the CRISPR/Cas9 system as described in Fauser et al. (2014). The DNA oligomers containing the protospacer and AtLAZY6 complementary sequence (Supplemental Table S1) were annealed to make double-stranded DNA and the resulting fragment was inserted into the Bbs I site of the pChimera vector, producing the pEn-Chimera. The expression cassette of single-guide RNA created in pEn-Chimera was excised with Avr II and recloned into the Avr II/Avr II site of the pCAS9-TPC binary vector (Fauser et al., 2014). A. tumefaciens cells were transformed with the binary vector. T1 plants were selected on MS plates containing BASTA and T2 seeds were obtained. The genomic DNA of T2 plants corresponding to the target region was sequenced and mutations were detected. A mutant with a thymine insertion that creates an early stop codon in AtLAZY6 was named atlazy6 and used to create a quintuple lazy mutant by crossing. Cleaved amplified polymorphic sequence (CAPS) markers were designed (Neff et al., 2002) to detect the mutation and used for genotyping (Supplemental Table S1).
Transient and Stable Expression in N. benthamiana and Arabidopsis
Arabidopsis plants were transformed using the floral dip method (Clough and Bent, 1998), and stable transformants harboring pHSP18.2:AtLAZY1-eGFP or its variants were obtained. Seedlings were grown on the surface of MS plates supplemented with Suc for 3 d in the dark. Heat shock was given by incubating plates with seedlings in 37°C for 5–6 h. Fluorescence from eGFP was observed right after the heat shock. Leaves from N. benthamiana were injected with A. tumefaciens strain GV3101 harboring expression vectors as described previously (Yoshihara et al., 2013). The overnight culture of Agrobacterium was pelleted by centrifugation and resuspended in deionized distilled water. Resuspended culture was injected into the abaxial side of the leaf and the injected leaves were left attached until observation (for 24–30 h).
Microscopy
A Zeiss LSM 510 laser scanning confocal microscope was used to visualize fluorescent proteins in plant cells. The optics employed were a Plan-Apochromat 20× lens and a C-Apochromat 40× water immersion lens. The sample was excited with the 488-nm laser line for eGFP and the 543-nm laser line for mRFP from a 30-mW argon gas laser. Channel mode detection was used to record the emission of eGFP (excitation 488 nm, emission 500–550 nm) or mRFP (excitation 543 nm, emission 565–615 nm) for subcellular localization.
Auxin Application
Auxin (Indole-3-acetic acid; Sigma-Aldrich) was dissolved in and diluted with ethanol. The dilution series was mixed with lanolin to make up lanolin pastes containing different concentrations of auxin. The final concentration of ethanol in the lanolin pastes was the same for all treatments and <0.1% (w/v). The lanolin paste was applied to one side of the inflorescence stem about 3 cm from the tip as an ∼4-mm-long streak along the long axis. The plants used were 6–7 weeks old, because the inflorescence stems of pAtLAZY1:AtLAZY1L92A/I94A/atlazy1 plants depicted a clearly drooping phenotype at this age.
Transcript Analysis by RT-qPCR
Transcript analysis of IAA5 was performed mostly as described in Taniguchi et al. (2014). Inflorescence stems grown to 22–25 cm were gravistimulated as in the time course imaging experiments described above. After gravistimulation, a segment of stem ∼0.5–5.5 cm below the apical meristem was cut into upper and lower halves along the longitudinal axis under a dissection microscope. Tissue samples from 10–13 stems per genotype and time point were pooled. Total RNA was extracted using an RNeasy Plant Mini Kit (Qiagen), including the treatment with RNase-free DNase (Qiagen). RT-qPCR was carried out using an Mx3000P QPCR system (Stratagene) with the KAPA SYBR FAST One-Step Universal Kit (KAPA Biosystems). Triplicate RT-qPCR assays were performed for each of three independently grown, treated, and harvested samples (biological replicates). ACTIN8 (ACT8) was used as an internal control. Relative amounts of IAA5 transcript were calculated using Pfaffl’s model (Pfaffl, 2001). Primers used were as described in Taniguchi et al. (2014).
Accession Numbers
Sequence data from this article can be found in the GenBank/EMBL data libraries under accession numbers AT5G14090 (LAZY1), AT1G17400 (LAZY2), AT1G19115 (LAZY3), AT1G72490 (LAZY4), AT3G24750 (LAZY5), and AT3G27025 (LAZY6).
SUPPLEMENTAL DATA
The following supplemental materials are available.
Supplemental Figure S1. Effects of mutation in AtLAZY1 conserved regions on subcellular localization: Additional examples to supplement those shown in Figure 3.
Supplemental Figure S2. Measurements from which the PDFs in Figure 3 were derived.
Supplemental Figure S3. Branch angle values in homozygous populations of pAtLAZY1:AtLAZY1L92A/I94A/atlazy1.
Supplemental Figure S4. Subcellular localization of N-terminal AtLAZY1 fused with eGFP.
Supplemental Figure S5. Shape of plants grown upside-down for 4 d and then returned to right-side up for 2 d.
Supplemental Figure S6. Double rotation experiment designed to determine whether reverse gravitropism of L1 and L2 (two lines homozygous for pAtLAZY1:AtLAZY1L92A/I94A/atlazy1;2;3;4 ) induced by 90° rotation would persist and therefore bend away from the plumb line after a second 90° rotation.
Supplemental Figure S7. IAA5 expression in atlazy1;2;3;4, L1/atlazy1;2;3;4, and L2/atlazy1;2;3;4 measured by reverse-transcription quantitative PCR
Supplemental Video S1. Excised wild-type inflorescence stem performing normal (negative) gravitropism.
Supplemental Video S2. Excised L1/atlazy1;2;3;4 inflorescence stem performing reverse (positive) gravitropism.
ACKNOWLEDGMENTS
We thank the Arabidopsis Biological Resource Center (The Ohio State University, Columbus), the European Arabidopsis Stock Centre, and the Institut Jean-Pierre Bourgin in the French National Institute for Agricultural Research for providing vectors and the seeds of Arabidopsis thaliana transfer DNA insertion lines. Microscopy was performed at the Newcomb Imaging Center, Department of Botany, University of Wisconsin–Madison. We also thank Alexys Hoppman, Emma Keel, and Ashley Perry for technical assistance.
LITERATURE CITED
Author notes
This work was supported by the National Science Foundation Plant Genome Research Program (IOS-1031416 to E.P.S.).
Articles can be viewed without a subscription.
Senior author.
The author responsible for distribution of materials integral to the findings presented in this article in accordance with the policy described in the Instructions for Authors (www.plantphysiol.org) is: Edgar P. Spalding ([email protected]).
T.Y. and E.P.S. conceived the investigation of conserved residues; T.Y. performed all of the genetics, molecular biology, and phenotype measurements; E.P.S. developed the bending force assay; T.Y. and E.P.S. performed data analysis; T.Y. and E.P.S. wrote the manuscript.