-
PDF
- Split View
-
Views
-
Cite
Cite
Wei Wei, Jian-Jun Tao, Hao-Wei Chen, Qing-Tian Li, Wan-Ke Zhang, Biao Ma, Qing Lin, Jin-Song Zhang, Shou-Yi Chen, A Histone Code Reader and a Transcriptional Activator Interact to Regulate Genes for Salt Tolerance , Plant Physiology, Volume 175, Issue 3, November 2017, Pages 1304–1320, https://doi.org/10.1104/pp.16.01764
- Share Icon Share
Abstract
Plant homeodomain (PHD) finger proteins are involved in various developmental processes and stress responses. They recognize and bind to epigenetically modified histone H3 tail and function as histone code readers. Here we report that GmPHD6 reads low methylated histone H3K4me0/1/2 but not H3K4me3 with its N-terminal domain instead of the PHD finger. GmPHD6 does not possess transcriptional regulatory ability but has DNA-binding ability. Through the PHD finger, GmPHD6 interacts with its coactivator, LHP1-1/2, to form a transcriptional activation complex. Using a transgenic hairy root system, we demonstrate that overexpression of GmPHD6 improves stress tolerance in soybean (Glycine max) plants. Knocking down the LHP1 expression disrupts this role of GmPHD6, indicating that GmPHD6 requires LHP1 functions during stress response. GmPHD6 influences expression of dozens of stress-related genes. Among these, we identified three targets of GmPHD6, including ABA-stress-ripening-induced CYP75B1 and CYP82C4. Overexpression of each gene confers stress tolerance in soybean plants. GmPHD6 is recruited to H3K4me0/1/2 marks and recognizes the G-rich elements in target gene promoters, whereas LHP1 activates expression of these targets. Our study reveals a mechanism involving two partners in a complex. Manipulation of the genes in this pathway should improve stress tolerance in soybean or other legumes/crops.
The plant homeodomain (also called the “PHD finger”) refers to a zinc-binding domain of 50 to 80 amino acids (Bienz, 2006). The PHD finger contains eight conserved metal-binding residues, Cys4HisCys3. The eight residues are divided into four pairs and are connected by variable sequence. Two zinc atoms are anchored in a cross-braced manner; the first zinc atom is anchored by the first and third pairs, whereas the second zinc atom is anchored by the second and the fourth pairs (Kwan et al., 2003).
PHD fingers are usually involved in protein-protein interaction. Normally, their binding partners are epigenetically modified histone-proteins. Various posttranslational modifications (PTMs) of histones throughout the genome constitute complicated histone code. For an example, histone H3 has different types of PTMs, such as methylation, acetylation, and phosphorylation, etc. (Jenuwein and Allis, 2001). Different PTMs lead to the difference in transcriptional states; methylated lysine4 (K4) and K36 are associated with on-state, whereas methylated K9 and K27 are associated with off-state (Jenuwein and Allis, 2001). The histone code is readable and PHD finger is one of these code readers (Mellor, 2006). PHD fingers can recognize different PTMs in a sequence-dependent manner, especially the methylation of Lys (Sanchez and Zhou, 2011). For example, the PHD finger of AIRE1 reads H3K4me0 (unmethylated H3; Org et al., 2008); GmPHD5 reads H3K4me2 (dimethylated H3K4; Wu et al., 2011); Alfin1-like proteins in Arabidopsis (Arabidopsis thaliana) prefer tri- or di-methylated H3K4 (H3K4me3/2; Lee et al., 2009); ING (inhibitor of growth) group proteins bind to H3K4me3 (Peña et al., 2006); PHD1 of CHD4 is reader of both H3K4me0 and H3K9me3; and PHD2 has the preference of H3K9me3 (Mansfield et al., 2011). Even though several PHD fingers read the same code, they may result in different regulatory mechanisms based on the characters of these readers. Two ING group proteins, ING2 and ING4, bind to H3K4me3. ING4 mediates cross talk between trimethylation and acetylation of H3 and leads to activation of target genes (Hung et al., 2009); however, ING2 interacts with H3K4me3 to switch off the active genes (Shi et al., 2006).
PHD finger proteins are ubiquitous in eukaryotes. In plants, they are involved in several important biological processes, such as meiosis, vernalization, anther development, and apical meristem maintenance (Wilson et al., 2001; Yang et al., 2003; Sung et al., 2006; Saiga et al., 2008). Among the plant PHD finger proteins, there is an Alfin1 group. This group is specific in plants and several members are present in various plant species (Lee et al., 2009). Alfin1-like proteins locate in the nucleus, they have transcriptional repression ability, and bind to the G-rich (GTGGNG/GNGGTG) elements in the promoter regions of their target genes. Previously, we have characterized the six Alfin1-like proteins in soybean (Glycine max) and find that the N-terminal domain plays a major role in DNA binding. GmPHD1 to GmPHD5 have transcriptional suppression activity but GmPHD6 does not have this activity (Wei et al., 2009). The Alfin1-like proteins are usually associated with abiotic stress responses (Wu et al., 2011; Song et al., 2013; Wei et al., 2015). They are also involved in elongation of root hairs during P starvation and the seed germination process (Chandrika et al., 2013; Molitor et al., 2014).
In the Alfin1 group, there are several special cases. For instance, Alfin1 found in Medicago sativa was reported to bind to the promoter of MsPRP2 and enhance but not suppress the expression of MsPRP2 (Bastola et al., 1998; Winicov and Bastola, 1999). Besides, AtAL6 from Arabidopsis is unable to bind to the G-rich element, because the N-terminal has two crucial point mutations (Wei et al., 2015). In this study, we reported another special case of Alfin1-like protein, GmPHD6, in soybean, whose transcriptional regulatory ability is somehow missing. The N-terminal domain of this protein can recognize the H3K4me0/1/2 and bind to the promoter of the target gene, whereas its PHD domain can recruit a coactivator LHP1-1 or LHP1-2 for transcriptional regulation. This protein complex would activate expression of downstream genes for stress tolerance in soybean. Our study reveals a specific mechanism for Alfin1-like proteins without direct transcriptional activation ability in response to abiotic stress in plants.
RESULTS
Overexpression of GmPHD6 Improves Salt Stress Tolerance in Transgenic Soybean Composites
Soybean has six Alfin1-like proteins named as GmPHDs. Five of these (GmPHD1 to GmPHD5) have transcriptional suppression activity and GmPHD2 improves stress tolerance. GmPHD6 does not have such transcriptional suppression activity; however, this protein can interact with other GmPHDs except GmPHD2, and the GmPHD6 gene can be induced by stresses (Wei et al., 2009). The GmPHD6 protein was pursued for its possible roles in stress response and the mechanism involved was further investigated. An Agrobacterium rhizogenes-mediated hairy root transformation system was first established, and the K599 strain harboring the GmPHD6-overexpression (G6-OE) and GmPHD6-RNA interference (G6-RNAi) constructs were injected into the hypocotyl of soybean seedlings for generation of transgenic hairy roots (Fig. 1A). The transgenic hairy roots grew out of the infection sites about two weeks later and the original roots were removed before subjecting to stress treatment.
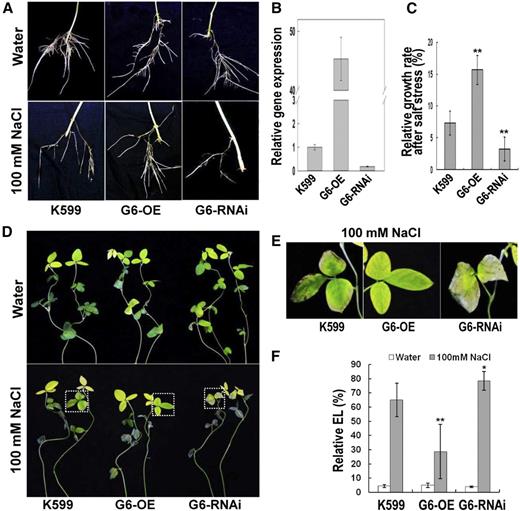
Performance of GmPHD6-transgenic hairy roots and the aerial part of soybean plants in response to stress. A, Performance of transgenic hairy roots after salt treatment. Soybean seedlings with transgenic hairy roots were treated with 100 mm NaCl for 3 d. Hairy roots in water were used as controls. Transgenic hairy roots with K599, GmPHD6-overexpression (G6-OE), and GmPHD6 RNA interference (G6-RNAi) were shown. B, Expression level of GmPHD6 gene in transgenic hairy roots. A soybean tubulin fragment was used as an internal control. Bars indicate sd (n = 3). C, Relative growth rate of transgenic hairy roots after salt stress, RGR (%) = (root elongation in salt) / (root elongation in water). Bars indicate se (n = 30). Asterisks indicate significant difference (**P < 0.01) compared to control hairy roots (K599). D, Performance of the aerial part of soybean plants after salt stress. Plants in water were used as control. E, Enlargement of the parts indicated in lower panels of (D) to show the performance of soybean leaves after salt stress. F, Relative electrolyte leakage in soybean leaves after salt stress. Bars indicate sd (n = 10). Asterisks indicate significant difference (*P < 0.05 and **P < 0.01) compared to leaves of control plants (K599).
Compared to control (K599) hairy roots, G6-OE roots had 45-fold increase of GmPHD6 expression, whereas G6-RNAi roots had approximately 80% reduction of GmPHD6 expression (Fig. 1B). To determine the performance of these hairy roots, relative growth rates were measured and compared. After salt stress with 100 mm NaCl, the relative growth rate of G6-OE hairy roots was apparently higher whereas this parameter in G6-RNAi roots was significantly lower than that in the control roots (Fig. 1C). The leaves of the plants with G6-OE roots also performed better than the control leaves; however, the leaves from the plants with G6-RNAi roots were more wilted than the control ones (Fig. 1, D and E). Relative electrolyte leakage was then measured to evaluate membrane damage of these leaves. After salt stress, leaves from plants with G6-OE roots showed lower leakage whereas the leaves from plants with G6-RNAi roots had higher leakage compared to control plants (Fig. 1F). These results indicate that GmPHD6 confers salt stress tolerance in soybean.
GmPHD6 Interacts with LHP1-1 or LHP1-2 to Activate Gene Expression
GmPHD6 has DNA binding ability and improves salt stress tolerance (Wei et al., 2009; Fig. 1); however, it does not have transcriptional activation and/or suppression ability (Wei et al., 2009). It is possible that GmPHD6 may recruit some cofactors for regulation of downstream genes and affect stress responses. We thus screened the possible proteins interacted with GmPHD6 by yeast two-hybrid assay, and two LHP1 proteins (like heterochromatin protein 1), namely LHP1-1 and LHP1-2, were identified. The interaction of full-length LHP1 and GmPHD6 was revealed by blue colors in yeast cells harboring AD-LHP1 and BD-GmPHD6 on QDO/X/A plates (Fig. 2A). The two LHP1 proteins share about 85% identity at the amino acid level. Both LHP1-1 and LHP1-2 contain a chromo domain (chromatin organization modifier) and a chromo shadow (CSD) domain in the C-terminal (Fig. 2B). Organ-specific expression was analyzed, and GmPHD6, LHP1-1, and LHP1-2 had similar expression levels in roots and shoots/stems, whereas GmPHD6 was expressed higher than the two LHP1 genes in leaves and flowers (Supplemental Fig. S1).
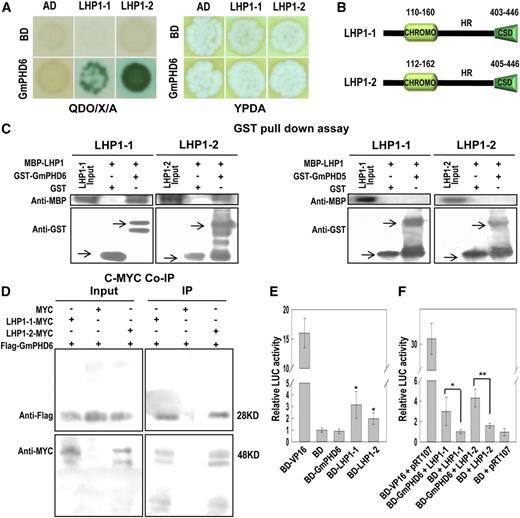
GmPHD6 interacts with LHP1-1 or LHP1-2 and the LHP1s have transcriptional activation activity. A, GmPHD6 interacts with LHP1-1 and LHP1-2 in a yeast two-hybrid assay. Yeast strains were grown on QDO/X/A or YPDA plates for 3 d. Blue colonies indicate positive interactions. B, Domain analysis of LHP1-1 and LHP1-2. C, Western-blotting result of GST pull-down assay. The MBP-LHP1 fusion protein was pulled down by GST-GmPHD6 but not by GST-GmPHD5 or GST. The arrows pointed to the expressed GST or its fusion proteins. D, Western-blotting result of the Co-IP assay. The Flag-GmPHD6 protein was coimmunoprecipitated with the LHP1-MYC protein using antic-Myc agarose beads. E, Transcriptional regulation activity of BD-GmPHD6 and/or BD-LHP1 determined by a DLR assay using an Arabidopsis protoplast. Relative LUC Activity = (Firefly LUC activity) / (Renilla LUC activity). For the plasmid ratio, pTRL:Reporter (LUC):Effector = 1:6:6 (µg/µg/µg). Bars indicate sd (n = 3). Asterisks indicate significant difference (**P < 0.05) compared to negative control (BD). F, The transcriptional regulation activity of the GmPHD6/LHP1 complex is shown. For the plasmid ratio, pTRL:LUC:BD-GmPHD6:35s-LHP1 = 1:6:6:6 (µg/µg/µg/µg). Bars indicate SD (n = 3). Asterisks indicate significant difference (*P < 0.05 and **P < 0.01) compared to BD+LHP1. CHROMO, chromatin organization modifier domain.
To confirm the interaction, we incubated GST or GST-GmPHD6 and MBP-LHP1-1 or MBP-LHP1-2 with Glutathione-Sepharose 4B beads to perform a GST pull-down assay. GST-GmPHD6 but not GST could pull down MBP-LHP1-1 or MBP-LHP1-2 (Fig. 2C). Another soybean PHD protein, GmPHD5, could not pull down any LHP1 protein (Fig. 2C). These results indicate that GmPHD6 could specifically interact with LHP1-1 or LHP1-2 in vitro. The in vivo interaction was further confirmed by the coimmunoprecipitation (Co-IP) assay. Flag-GmPHD6 and MYC-LHP1 were transiently expressed in tobacco (Nicotiana tabacum) leaves for the IP assay. Protein extract was incubated with agarose beads that cross-linked with antic-MYC antibody. A western-blotting assay showed that these agarose beads captured c-Myc-LHP1-1 and Flag-GmPHD6. When empty MYC vector and Flag-GmPHD6 were expressed, the agarose beads could not capture any protein (Fig. 2D). This result indicates that GmPHD6 interacted with LHP1 in vivo.
A transcriptional activation assay was performed to test whether LHP1-1 and LHP1-2 had any transcriptional regulatory effect. In a dual-luciferase reporter (DLR) assay system, a firefly luciferase (LUC) was used as a reporter and a Renilla luciferase was used as an internal control. The LUC gene was driven by a minimal 35S promoter connected by five copies of the GAL4 binding element (Supplemental Fig. S2A). The GAL4 DNA binding domain (BD) could bind to the GAL4 element. If the tested protein has transcriptional activation activity, the BD fusion protein will enhance the expression of LUC gene and thus have a higher relative LUC activity than the control (BD). The result showed that LHP1-1 and LHP1-2 but not GmPHD6 had transcriptional activation ability (Fig. 2E). We further tested the transcriptional regulatory effect of the GmPHD6/LHP1 complex using a modified assay. BD-GmPHD6 and 35S-LHP1 were coexpressed in Arabidopsis protoplasts (Supplemental Fig. S2B). A DLR assay showed that BD-GmPHD6 plus LHP1-1 or LHP1-2 had higher LUC activity than control samples (BD + LHP1-1 or BD + LHP1-2 or BD-GmPHD6 + Actin; Fig. 2F; Supplemental Fig. S2C). These results indicate that BD-GmPHD6 formed a complex with LHP1-1 or LHP1-2 to bind to the GAL4 element through BD-GmPHD6 and to activate the expression of LUC gene through LHP1-1 or LHP1-2 recruited to GmPHD6.
Domain Analysis of GmPHD6 Binding to LHP1, DNA Element, and Histone H3
GmPHD6, like other GmPHD proteins, consists of two conserved domains, the N-terminal domain (N) and the PHD finger, connected by a variable region (V; Fig. 3A). Because GmPHD6 interacted with LHP1-1 or LHP1-2 (Fig. 2), we investigated which domain in GmPHD6 is responsible for the interaction. Each of the single domains, including G6N, G6V, and G6PHD (G6P), or combination of them (G6NV, G6VP), was expressed as GST-tagged proteins and tested in a GST pull-down assay. The results showed that G6P and G6VP could pull down the LHP1-1 in vitro, whereas other domains or combinations could not (Fig. 3A, lower panel), indicating that the PHD finger plays a major role in protein interaction.
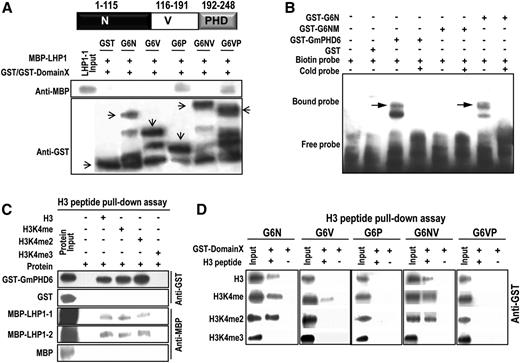
Domain analysis of GmPHD6 binding to LHP1, DNA element, and histone H3. A, Western-blotting result of the GST pull-down assay. Domain arrangement of GmPHD6. The MBP-LHP1 fusion protein was pulled down by GST-G6P or GST-G6VP. The arrows pointed to the expressed GST or its fusion proteins with various domains of GmPHD6. B, EMSA assay of GmPHD6, G6N, and G6NM (V34M, E35V). 4× G-rich box (GTGGAG) elements were used in the EMSA assay. C, Western-blotting result of the peptide pull-down assay. GST-GmPHD6 or MBP-LHP1 fusion protein was pulled down by the biotin-conjugated histone H3 peptide. D, The N-terminal domain of GmPHD6 interacted with a low-methylated histone H3 peptide in a peptide pull-down assay. N: N-terminal domain, amino acids 1 to 115; V: Variable domain, amino acids 116 to 191; PHD finger: amino acids 192 to 248.
Our previous study shows that the N-terminal domains of other GmPHD proteins play major roles in DNA binding (Wei et al., 2009), and mutation of two amino acids in the N-terminal domain caused the functional loss of G-rich element binding activity in Arabidopsis PHD protein AtAL6 (Wei et al., 2015). Thus, we performed an EMSA assay to test the DNA binding property of GmPHD6. The results showed that the full length of GmPHD6 and the N-terminal domain bound to the G-rich (GTGGAG) elements, whereas the mutated N-terminal domain G6NM (V34M, E35V) could not bind to the G-rich elements (Fig. 3B). These results indicate that the N-terminal domain of GmPHD6 is responsible for DNA binding.
Because the PHD domain often interacts with the histone code in chromatin, we tested whether GmPHD6 and LHP1-1 or LHP1-2 could associate with histone H3. A peptide (biotin-conjugated H3 peptide) pull-down assay was performed and the results showed that GmPHD6 could bind to unmethylated (H3), monomethylated (H3K4me), and dimethylated H3 at K4 (H3K4me2) but not the trimethylated H3 at K4 (H3K4me3; Fig. 3C). It should be noted that LHP1-1 and LHP1-2 showed very weak binding intensity toward H3, H3K4me, and H3K4me2 compared to the strong input signals (Fig. 3C). All these results suggest that GmPHD6, but not LHP1, played major roles in H3K4me0/1/2 recognition. Different domains of GmPHD6 were further examined for their interaction with H3 peptide. It is shown that G6N and G6NV, but not G6P or G6VP, could bind to H3K4me0/1/2 (Fig. 3D). Only very weak interaction with the H3K4me was noted when the single V domain was studied (Fig. 3D). These results indicate that N-terminal domain of GmPHD6 may play a major role in ‘histone code’ reading in addition to its DNA binding.
LHP1 Is Required for Salt Stress Tolerance
Because the GmPHD6 confers stress tolerance and interacts with LHP1-1 and LHP1-2, we studied whether the LHP1-1 and LHP1-2 play any roles in salt stress responses. We overexpressed LHP1-1 or LHP1-2 gene in transgenic hairy roots of soybean plants and tested their performance. We found that overexpression of LHP1-1 or LHP1-2 could not enhance stress tolerance of the transgenic hairy roots (Supplemental Fig. S3). We then selected a 379-bp fragment of the LHP1-1 coding region for the RNA interference test. This fragment showed 97% identity with that from LHP1-2 (Supplemental Fig. S4). The expression of LHP1-1 and LHP1-2 in LHP-RNAi hairy roots was simultaneously knocked down to less than 10% of that in control roots (K599; Fig. 4A). After salt stress for 3 d, the LHP-RNAi transgenic hairy roots grew to a shorter length than control roots; the relative growth rate of LHP-RNAi hairy roots was significantly reduced compared to the value in control roots (Fig. 4, B and C). Meanwhile, leaves of soybean plants with LHP-RNAi transgenic roots had more severe damage and higher electrolyte leakage than that of control plants (Fig. 4, D, E, and F). The results demonstrate that decrease of LHP1 gene expression leads to sensitive responses in soybean seedlings, indicating that LHP1 is required for plant tolerance to salt stress.
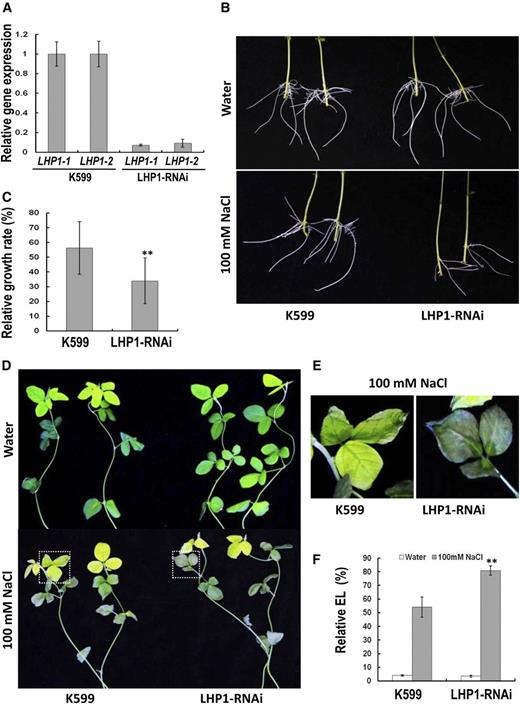
Performance of LHP1-RNAi hairy roots and the aerial part of soybean plants in response to salt stress. A, Expression level of LHP1-1 and LHP1-2 genes in transgenic hairy roots. B, Performance of transgenic hairy roots after salt treatment. Soybean seedlings with transgenic hairy roots were treated with 100 mm NaCl for 3 d. Hairy roots in water were used as controls. C, Relative growth rate of LHP1-RNAi hairy roots after salt stress. Bars indicate se (n = 30). Asterisks indicate significant difference (**P < 0.01) compared to control hairy roots (K599). D, Performance of the aerial part of soybean plants with transgenic hairy roots after salt stress. Plants in water were used as controls. E, Enlargement of the parts indicated in (D) to show the performance of soybean leaves after salt stress. F, Relative electrolyte leakage in leaves of soybean with transgenic roots. Bars indicate sd (n = 10). Asterisks indicate significant difference (**P < 0.01) compared to leaves of control plants (K599).
LHP1 Acts Downstream of GmPHD6 for Salt Tolerance
Considering that overexpression of the LPH1 could not enhance stress tolerance, and GmPHD6 formed complex with LPH1, we tested whether co-over-expression of these two components in transgenic hairy roots would have any effects on stress response. We mixed equal amounts of A. rhizogenes harboring the GmPHD6-overexpression construct and the LHP1-1-overexpression construct and infected soybean hypocotyls for generation of coexpressed transgenic hairy roots. The soybean plants with both GmPHD6- and LHP1-1-overexpressing transgenic hairy roots showed better salt tolerance than the plants with single GmPHD6-transgenic roots or control roots, as judged from the leaf performance and relative electrolyte leakage (Fig. 5, A, B, and C). The expression of GmPHD6 and LHP1-1 was higher in cotransgenic hairy roots than that of the K599 control (Fig. 5D). However, the GmPHD6 level was lower in the cotransgenic roots than the single GmPHD6-trangenic roots (Fig. 5D). These results likely indicate that the cooperation of GmPHD6 and LHP1 in a complex may be important for salt tolerance.
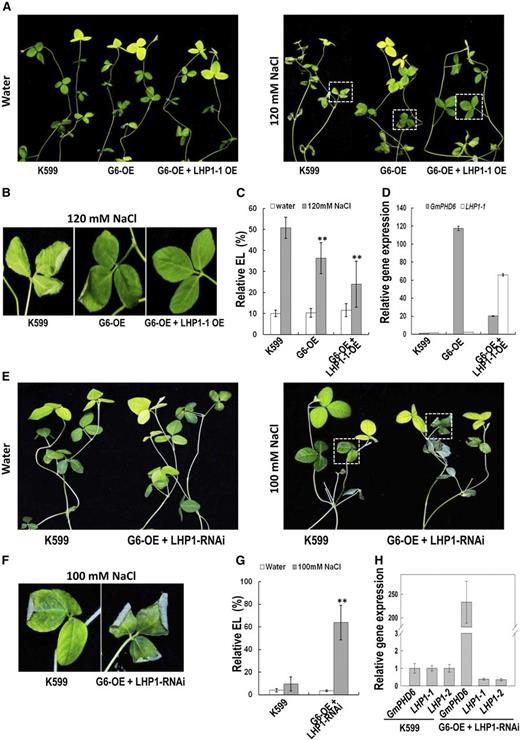
Performance of soybean plants with cotransgenic hairy roots in response to salt stress. A, Performance of the aerial part of soybean plants after salt stress. Soybean seedlings with K599, G6-OE, and G6-OE + LHP1-1-OE transgenic hairy roots were treated with 120 mm NaCl for 3 d. Plants in water were used as control. B, Enlargement of the parts indicated in right panel of (A) to show the performance of soybean leaves after salt stress. C, Relative electrolyte leakage in leaves of soybean with transgenic hairy roots. Bars indicate sd (n = 10). Asterisks indicate significant difference (**P < 0.01) compared to leaves of control plants (K599). D, Expression level of GmPHD6 and LHP1-1 genes in transgenic hairy roots. E, Performance of the aerial part of soybean plants after salt stress. Soybean seedlings with G6-OE and LHP1-RNAi transgenic hairy roots were treated with 100 mm NaCl for 3 d. Plants in water were used as control. F, Enlargement of the parts indicated in right panel of (E) to show the performance of soybean leaves after salt stress. G, Relative electrolyte leakage in leaves of soybean with transgenic hairy roots. Bars indicate sd (n = 10). Asterisks indicate significant difference (**P < 0.01) compared to leaves of control plants (K599). H, Expression level of GmPHD6, LHP1-1, and LHP1-2 genes in transgenic hairy roots.
To study the genetic relationship between GmPHD6 and LHP1, equal amounts of A. rhizogenes harboring GmPHD6-overexpression construct or LHP1-RNAi construct were mixed and coinjected to soybean seedlings for infection and generation of transgenic hairy roots. After salt treatment for 3 d, the soybean seedlings with the cotransgenic hairy roots had leaves more damaged than those from control plants. The electrolyte leakage was also higher in the leaves of cotransgenic composites than those of the control plants (Fig. 5, E, F, and G). The expression of GmPHD6 was much higher in cotransgenic hairy roots than those of control roots; however, the expression of LHP1-1 and LHP1-2 in cotransgenic hairy roots was significantly reduced compared to that in the control roots (Fig. 5H). These results demonstrate that even though GmPHD6 was highly expressed, which is beneficial for salt tolerance, the knock-down of the two LHP1 genes would disrupt the salt tolerance, suggesting either that GmPHD6 depends on LHP1 or that LHP1 acts downstream of GmPHD6 for stress-tolerant response in soybean.
Two Conserved Residues at the N-Terminal Domain Are Necessary for GmPHD6-Mediated Salt Tolerance
The mutation of two amino acids in the N-terminal domain caused the functional loss of G-rich element binding activity in GmPHD6 (Fig. 3B). We then tested whether mutation of the two conserved corresponding amino acids would affect GmPHD6 function. A mutated form of GmPHD6, G6M (V34M, E35V), was generated and transformed into soybean hairy roots (Fig. 6A). The soybean plants with the transgenic hairy roots exhibited worse growth than control plants in 100 mm NaCl (Fig. 6, B and C). Meanwhile, the electrolyte leakage in leaf of soybean seedlings with G6M-OE transgenic roots was significantly higher than that of control plants (Fig. 6D). The transgenic hairy roots had very high expression of this mutated gene (approximately 200-fold; Fig. 6E). These results indicate that overexpression of this mutated GmPHD6 gene caused salt sensitivity in soybean plants.
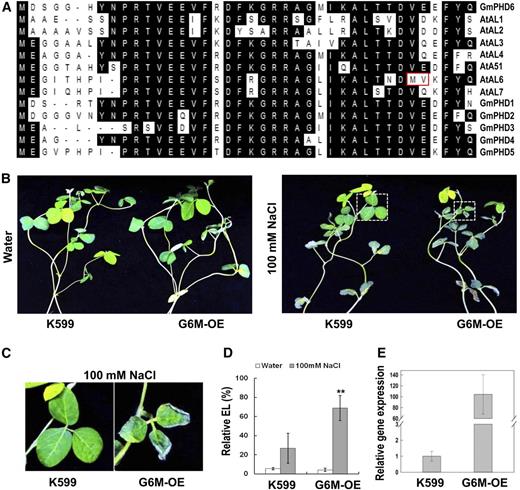
Performance of soybean plants with G6M (V34M, E35V) transgenic roots in response to salt stress. A, Sequence alignment of the first 40 amino acids from the N terminus of soybean and Arabidopsis PHD proteins. The two amino acids that are boxed in red caused the loss of G-rich element binding activity in AtAL6. The Val at the 34th and the Glu at the 35th positions of the GmPHD6 were mutated to Met and Val, respectively. Amino acids shaded in black indicate identity. B, Performance of the aerial part of soybean plants with G6M-OE transgenic roots after salt stress. Soybean seedlings with transgenic hairy roots were treated with 100 mM NaCl for 3 d. Plants in water were used as controls. C, Enlargement of the parts indicated in the right panel of (B) to show the performance of soybean leaves after salt stress. D, Relative electrolyte leakage in soybean leaves. Bars indicate sd (n = 10). Asterisks indicate significant difference (**P < 0.01) compared to leaves of control plants (K599). E, Expression level of the mutated GmPHD6 gene in transgenic hairy roots.
GmPHD6 Specifically Regulates Downstream Genes for Salt Stress Tolerance
To identify the downstream genes that are regulated by GmPHD6, an RNA sequencing analysis was performed. RNA extracted from G6-OE, G6-RNAi, and K599 control hairy roots was used for RNA sequencing. Data of clean reads in RNA sequencing is shown in Supplemental Table S1. Compared to the transcripts of K599 control roots, G6-OE roots had 553 up-regulated genes and 987 down-regulated genes, whereas G6-RNAi roots had 2707 up-regulated genes and 3032 down-regulated genes (Supplemental Fig. S5A). We focused on the downstream genes that were up-regulated in G6-OE roots and down-regulated in G6-RNAi root samples. A Venn diagram showed that there were 59 of these downstream genes (Supplemental Fig. S5B; Supplemental Table S3). We further tested 13 of them using quantitative reverse transcription PCR (RT-PCR). These genes were all up-regulated in G6-OE roots and down-regulated in G6-RNAi roots (Fig. 7A; Supplemental Fig. S5C). The expression of target genes depends on the binding of GmPHD6 toward their promoter regions and the activation effect of LHP1-1 or LHP1-2. These target genes should not be up-regulated in G6M-OE root samples and should be down-regulated in LHP1-RNAi samples. Based on this assumption, four genes out of the 13, namely CYP75B1, CYP71A22, ABA-stress-ripening (ASR), and CYP82C4, were found to have the consistent expression pattern (Fig. 7A).
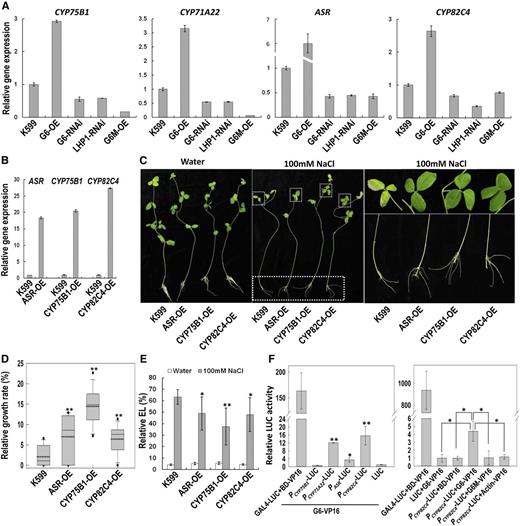
GmPHD6 regulates gene expression and binds to the promoter regions of target genes. A, Expression of downstream genes in G6-OE, G6-RNAi, LHP1-RNAi, and G6M (V34M, E35V)-OE transgenic hairy roots. B, Expression of ASR, CYP75B1, and CYP82C4 genes in their transgenic hairy roots. C, Performance of soybean plants with ASR-OE, CYP75B1-OE, or CYP82C4-OE transgenic roots after salt stress. Plants in water were used as control. Enlargement of the parts is given in the middle photo to show the performance of soybean leaves and roots after salt stress. D, Relative growth rate of transgenic hairy roots after salt stress. Bars indicate se (n = 30). Asterisks indicate significant difference (**P < 0.01) compared to control hairy roots (K599). E, Relative electrolyte leakage in leaves of soybean with transgenic hairy roots. Bars indicate sd (n = 10). Asterisks indicate significant difference (*P < 0.05 and **P < 0.01) compared to leaves of control plants (K599). F, Promoter-LUC activity mediated by GmPHD6 binding. The ability with which GmPHD6 bound to the promoter regions of downstream genes was determined by the DLR assay. Bars indicate sd (n = 3). In the left panel, asterisks indicate significant difference (*P < 0.05 and **P < 0.01) compared to control samples (LUC). In the right panel, asterisks indicate significant difference (*P < 0.05) compared to control samples (LUC plus G6-VP16 or PCYP82C4-LUC plus BD-VP16 or PCYP82C4-LUC plus Actin-VP16) and mutated protein (PCYP82C4-LUC plus G6M-VP16).
We transformed the CYP75B1, ASR, and CYP82C4 into soybean hairy roots (Fig. 7B) to test gene functions in response to salt stress. After salt treatment for 3 d, the soybean seedlings with transgenic hairy roots overexpressing the three genes grew better and had a higher relative growth rate than control roots (Fig. 7, C and D). The aerial part of the transgenic composites also had better performance and their leaves had lower electrolyte leakage compared to control plants (Fig. 7, C and E). These results indicate that the downstream genes play positive roles in salt tolerance of soybean.
Through sequence scanning, we found several G-rich elements (GTGGNG/GNGGTG) in promoter regions of the downstream genes, except for CYP75B1. A promoter LUC assay was performed to test whether the GmPHD6 could bind to these promoter regions. The promoter regions of the four downstream genes were cloned and inserted in front of the LUC gene, replacing the original GAL4 elements and the mini 35S promoter in the GAL4-LUC reporter. A GmPHD6-VP16 fusion construct was used as an effector (Supplemental Fig. S2D). If GmPHD6 can bind to the promoter region, the expression of the LUC gene would be activated and up-regulated by VP16. The result showed that the promoter activity of the CYP71A22, ASR, and CYP82C4 but not CYP75B1 was enhanced, suggesting that GmPHD6 most likely can bind to the promoters of CYP71A22, ASR, and CYP82C4 (Fig. 7F, left).
We further examined the DNA binding ability of the control fusions by replacing GmPHD6 with AtActin7, BD, or G6M in the GmPHD6-VP16 construct (Supplemental Fig. S2D). These new constructs were used as negative controls for G-rich element binding because Actin does not bind to DNA, and BD domain and the mutated GmPHD6 protein G6M (V34M, E35V) cannot bind to G-rich elements. In the presence of the PCYP82C4-LUC reporter, Actin-VP16 and BD-VP16 produced a low LUC activity, which was similar to that in the control sample (LUC + G6-VP16; Fig. 7F, right panel). The mutated GmPHD6 protein, G6M, also generated low LUC activity. In contrast, the combination of PCYP82C4-LUC plus GmPHD6-VP16 produced higher LUC activity compared to the above negative controls (Fig. 7F, right). These results indicate that GmPHD6 specifically binds to the promoter region of CYP71A22, ASR and CYP82C4 for regulation of gene expression.
GmPHD6 Promotes Stomatal Closure upon Salt Treatment
Because the aerial parts of the soybean plants with GmPHD6-overexpressing hairy roots also showed stress tolerant phenotype (Figs. 1 and 5), we further examined the possible mechanisms involved. We found that the stomata opening was significantly reduced in leaves of soybean plants with GmPHD6-overexpressing hairy roots compared to the control plants after salt stress for 1 h (Fig. 8, A and B). In contrast, the stomatal aperture was increased in leaves of soybean plants with GmPHD6-RNAi roots (Fig. 8, A and B). We also measured relative water content (RWC) in all the trifoliate leaves after the plants with transgenic hairy roots were stressed in 100 mm NaCl for 3 d. Leaves from plants with GmPHD6-overexpressing hairy roots had higher RWC than that of control plants, whereas leaves from plants with RNAi hairy roots had lower RWC (Fig. 8C). These results indicate that GmPHD6 from transgenic hairy roots may improve stress tolerance of aerial parts through regulation of stomata closing and water contents in leaves.
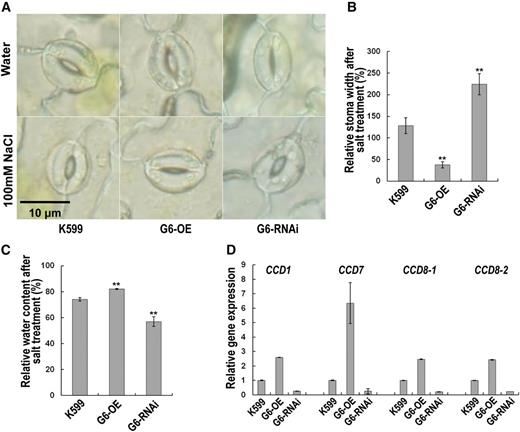
GmPHD6 regulates stomatal closure in salt stress. A, The second trifoliate from the top was chosen for stoma observation. Bar indicates the length that equals 10 μm. B, Relative stoma width (%) equals the stoma width of well-watered plants divided by the stoma width of plants treated by 100 mm NaCl for 1 h. Values indicate means ± sd (n = 60). Stomata from three plants were measured for stoma width with 20 stomata from each plant. C, All the trifoliates were cut to measure RWC. RWC = (water content after salt treatment) / (water content in water; %). Bars indicate sd (n = 3). Each replicate contains five plants. Asterisks indicate significant difference (**P < 0.01) compared to K599. D, Expression of downstream genes in K599, G6-OE, and G6-RNAi transgenic hairy roots. A soybean tubulin fragment was used as an internal control. Bars indicate sd (n = 3).
Usually, the stomata closure is related to the ABA pathway. However, GmPHD6 did not regulate ABA-related genes (Supplemental Fig. S6A). We further analyzed the transcriptome data and found several homologs of carotenoid cleavage dioxygenase (CCD) genes, which take part in strigolactone biosynthesis (López-Ráez et al., 2010). Their expressions had approximately 1.5-fold increases in GmPHD6-overexpressing hairy roots and more than 80% reduction in RNAi hairy roots (Supplemental Fig. S6B). These expression patterns were further confirmed by qRT-PCR (Fig. 8D). Considering that the strigolactone can be transported from roots to shoots, and it is also involved in stomata closure during stress response (Ha et al., 2014), we propose that overexpressing GmPHD6 in hairy roots may confer salt tolerance in the aerial part of the soybean plants at least partially through strigolactone-mediated stomata closure.
DISCUSSION
Typically, a transcription factor/regulator possesses two functional domains, a DNA binding domain and a transcriptional regulatory domain. The regulatory domain has either transcriptional activation or suppression activity. GmPHD6 belongs to a small Alfin1-like group of PHD finger proteins. Normally, Alfin1-like proteins are transcriptional suppressors; they play biological roles through inhibition of their downstream genes (Wei et al., 2009, 2015). However, GmPHD6 somehow loses its suppression activity (Fig. 2E). It is rationally presumed that GmPHD6 may recruit certain transcriptional cofactors, as in the case of REVEILLE (RVE4/8), which interacts with coactivators NIGHT LIGHT-INDUCIBLE AND CLOCK-REGULATED (LNK1/2) for regulation of downstream genes (Xie et al., 2014). Using a yeast two-hybrid system, we identified two chromo domain-containing transcriptional activators, LHP1-1 and LHP1-2, which specifically interacted with GmPHD6 but not GmPHD5 (Fig. 2). GmPHD6 may recruit LHP1-1/2 through its PHD domain to form a transcriptional regulatory complex, with the N-terminal domain of GmPHD6 acting as a DNA binding domain and LHP1-1/2 acting as a transcription-activating domain (Fig. 9).
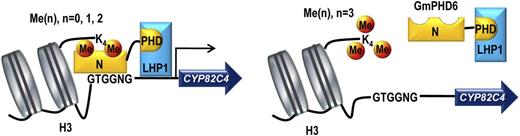
A model of the GmPHD6/LHP1 complex recognizing histone code and mediating expression of downstream genes. Histone H3 can be methylated (orange circles) at the unstructured Lys-4. GmPHD6 (yellow) is recruited to H3K4me0/1/2 marks but not H3K3me3. GmPHD6 recognizes both the histone code and the G-rich box (GTGGNG) in the promoter regions through its N-terminal domain. GmPHD6 interacts with LHP1 (blue) through the PHD finger. LHP1 functions as a transcriptional activator and activates expression of downstream genes.
GmPHD6 enhanced stress tolerance in soybean plants with GmPHD6-overexpressing transgenic hairy roots. This function requires the DNA binding ability of GmPHD6 N-terminal domain because mutation of the two conserved residues in the N-terminal caused the inability of GmPHD6 to bind to the promoter of its downstream genes (Fig. 7F). The mutation version of the GmPHD6 even led to salt sensitivity when overexpressed in transgenic hairy roots (Fig. 6), possibly due to the formation of a nonfunctional complex, where the mutated GmPHD6 may compete with and replace the original GmPHD6 for LHP1 association and further affect stress response.
Although the LHP1-1 and LHP1-2 were associated with GmPHD6, overexpression of LHP1-1 and LHP1-2 could not enhance stress tolerance in transgenic hairy roots (Supplemental Fig. S3). However, the two LHP1 proteins are required for stress tolerance as judged from the fact that soybean seedlings with LHP1-RNAi transgenic roots are sensitive to salt stress (Fig. 4). It is the GmPHD6/LHP1 complex that plays an essential role in salt tolerance, and hence cotransformation of the GmPHD6 and LHP1-1 confers even higher salt tolerance than overexpressing GmPHD6 alone (Fig. 5). Because there are two LHP1 proteins in soybean, sharing a high similarity of about 85% (Supplemental Fig. S4), LHP1 proteins may be excessive compared to GmPHD6 in roots. Consistently, the total expression level of LHP1 (LHP1-1 plus LHP1-2) is about twice of that of GmPHD6 in roots (Supplemental Fig. S1). Without enough GmPHD6, the overexpressed LHP1 proteins would be unbounded and thus ineffective. Moreover, knocking down the LHP1-1/2 expression in the background of GmPHD6 overexpression abolished stress tolerance in soybean, demonstrating that GmPHD6 requires the LHP1-1/2 for stress tolerance (Fig. 5). Because GmPHD6 can bind to a G-rich element but have no transcriptional regulatory activity, it is reasonable that GmPHD6 recruits LHP1-1/2 for transcriptional activation of downstream genes in response to different abiotic stresses.
Previous studies find that the PHD finger could read histone code, especially the trimethylated K4 of histone H3 (H3K4me3; Li et al., 2006; Peña et al., 2006). In this study, GmPHD6 also binds to methylated histone tails, but in a different manner. GmPHD6 could bind to H3K4me0/1/2, but not H3K4me3 (Fig. 3C). It is interesting to note that the N-terminal domain but not the PHD finger of GmPHD6 reads histone code (Fig. 3D). The functions of PHD fingers diverge even in the Alfin1 group. For example, the PHD finger of AtAL1, AtAL4, and AtAL7 could bind to H3K4me2/3, but the PHD finger of AtAL3 did not have this ability (Lee et al., 2009). Meanwhile, another soybean PHD protein, GmPHD5, has the preference of recognizing H3K4me2 (Wu et al., 2011). These differences may be caused by the sequence diversity in PHD fingers. It is also reported that the binding partners of a PHD finger were not necessarily histone proteins; they could be nonhistone proteins instead (Li and Li, 2012). Just like this case of GmPHD6, the PHD finger recognized LHP1 as its binding partner (Fig. 3A). Interestingly, whereas the N-terminal domain of GmPHD6 can bind to histone H3 (Fig. 3D), this domain also binds to the DNA element (Fig. 3B). The N-terminal domain, consisting of about 120 amino acids, is named according to the sequence similarity among Alfin1 family proteins. It is not clear why the N-terminal domain of GmPHD6 can bind to both the histone H3K4me0/1/2 and DNA elements. It is possible that this domain may contain two functional subdomains, with the former part for DNA binding and the latter part for H3 binding. The fact that mutation of the residues at the 34th and 35th positions disrupted DNA binding of N-terminal domain may support the above prediction (Fig. 3B). Further studies should be conducted to test such a possibility.
LHP1 consists of two evolutionarily conserved domains, the chromo domain (CD) near the N-terminal, and the CSD at the C-terminal (Fig. 2B). The CD is known as a methylated histone H3 binding module. Generally, LHP1 is known to bind to the transcriptional repressive mark of H3K9me3 (Stewart et al., 2005). In Arabidopsis, AtLHP1 regulates development through repression of its target genes (Cui and Benfey, 2009; Exner et al., 2009). Here we report different functions of two soybean LHP1 proteins in transcriptional activation (Figs. 2E and 3C). Except for the two conserved domains, the two soybean LHP1s showed very little similarity with AtLHP1. LHP1 generally has a hinge region. The hinge regions are variable among different LHP1 proteins, which may exert different biochemical functions, including RNA binding and transcriptional activation (Hiragami and Festenstein, 2005; Lomberk et al., 2006; Keller et al., 2012). Therefore, soybean LHP1-1 and LHP1-2 play completely different biochemical and biological roles from AtLHP1. Although the CSD shares similarity with the CD, it may function differently as a dimerization module (Mendez et al., 2011; Nishibuchi and Nakayama, 2014). Thus, it is possible that the CSD may interact with the PHD finger of GmPHD6 to form the complex.
Compared to LHP1, GmPHD6 plays a major role in recognizing H3K4me0/1/2 (Fig. 3C). Histone H3K4me0/1/2 may recruit GmPHD6, which further recruits its cofactor LHP1 to form a transcriptional regulatory complex (Fig. 9). GmPHD6 may act as a code reader because it not only reads histone code but also reads the DNA cis-element in the DNA strands (Figs. 3, B and C, and 7F). After GmPHD6 locates the target genes, LHP1 activates expression of them (Figs. 2E and 9). Normally, H3K4me3 is associated with active transcription (Mellor, 2006). However, GmPHD6 prefers low-methylated H3K4 (H3K4me0/1/2), but not H3K4me3 (Fig. 3C). Generally, under normal growth conditions, stress-related genes are not highly expressed. They can be induced by different stresses and in various organs of plants. It is reported that genes associated with H3K4me3 are highly expressed but low tissue-specific, whereas those genes associated with H3K4me and H3K4me2 are highly tissue-specific but not always activated in transcription (Zhang et al., 2009). It is therefore likely that, in response to stresses, GmPHD6 may recognize low-methylated H3K4 and interact with LHP1-1/2 to form a complex, which activates the associated target genes for stress tolerance in a specific manner (Fig. 9). It should be noted that whereas the H3K4me0/1/2-GmPHD6-LHP1 complex is mainly derived from the transgenic root studies, the complex may also work similarly in aerial parts of soybean plants, although further investigation should be performed to corroborate this prediction.
Unlike other Alfin1-like proteins suppressing expression of negative factors during stress response (Wei et al., 2009, 2015), the interaction of GmPHD6 with LHP1 activates transcription of its target genes (Fig. 7A; Supplemental Fig. S5B). These target genes play positive roles in stress tolerance (Fig. 7A; Supplemental Table S2). Three of the four possible targets are cytochrome P450-coding genes. The cytochrome P450 superfamily consists of large numbers of monooxygenase enzymes, which catalyze oxidation/reduction reactions of enormous organic chemicals (Munro et al., 2013; Rendic and Guengerich, 2015). They are also involved in drug metabolism, endocrine activity, and steroid and hormone biosynthesis, as well as other processes (Girvan and Munro, 2016; Guengerich et al., 2016). In plants, CYP genes take part in the biosynthesis or catabolism of several phytohormones, such as cytokinins, ABAs, gibberellins, and brassinosteroids (Saito et al., 2004; Takei et al., 2004; Zhu et al., 2006; Sakamoto et al., 2012; Yoshida, 2012). Recently, increasing evidence showed that P450s confer abiotic stress tolerance in plants (Rai et al., 2015; Tamiru et al., 2015; Wang et al., 2016). Presently, we have identified three CYP genes (CYP75B1, CYP71A22, and CYP82C4) up-regulated by GmPHD6 (Fig. 7B). CYP75B1 encodes an enzyme that mediates synthesis of phenylpropanoid; CYP71A22 is associated with synthesis of furanocoumarins; and CYP82C4 is related to transport of iron ion (Rupasinghe et al., 2003; Murgia et al., 2011; Chen et al., 2014). Overexpression of CYP82C4 and CYP75B1 enhance salt tolerance in soybean plants with transgenic hairy roots (Fig. 7). The relevant regulatory mechanism requires further investigation.
ASR is a transcription factor with an ABA and water-deficit-stress-induced domain at its C-terminal end. This protein is usually associated with ABA transduction and fruit ripening (Jia et al., 2016). ASR genes are also stress-responsive genes (Yang et al., 2005; Arenhart et al., 2013). A wheat ASR gene, TaASR1, was reported to confer drought tolerance in transgenic tobacco through regulating ROS signaling (Hu et al., 2013). Our present findings indicate that ASR is directly regulated by the GmPHD6/LHP1 complex, and promotes salt tolerance in soybean (Fig. 7).
It is interesting to note that although the aerial parts of the soybean plants with GmPHD6-overexpressing hairy roots were nontransgenic, they also exhibited a salt-tolerant phenotype, most likely due to stomatal closure and higher water content being maintained (Figs. 1 and 8). Usually ABA could promote stomatal closure and reduce water loss (Acharya and Assmann, 2009). However, overexpressing GmPHD6 did not enhance expression of ABA-related genes substantially (Supplemental Fig. S6A). Instead, we found expression of several CCD genes was up-regulated by GmPHD6 (Supplemental Fig. S6B; Fig. 8D). Arabidopsis CCD7 and CCD8 take part in strigolactone synthesis (López-Ráez et al., 2010), and strigolactones are root-derived hormones, which could be transported from roots to the aerial parts of plants (Kohlen et al., 2011; Seto and Yamaguchi, 2014). Moreover, strigolactone plays important roles in stress tolerance through mediating stomatal closure (Ha et al., 2014). Another CCD gene, CCD1, was also regulated by GmPHD6 (Fig. 8). CCD1 is involved in the biosynthesis of cyclohexanone but not strigolactone, yet CCD1 is also related to stress response (Sun et al., 2008; Kim et al., 2014). Our findings suggest that overexpressing GmPHD6 in hairy roots may affect strigolactone synthesis and enhance salt tolerance in the aerial parts of soybean through promoting stomatal closure and maintaining water content. Other factors may also be involved in the stress tolerance of the soybean aerial parts (Pan et al., 2016).
Taken together, we propose a working model for the GmPHD6-LHP1-1/2 transcriptional activation complex (Fig. 9). In response to a given stress, GmPHD6 is recruited to low methylated H3 (H3K4me0/1/2). Through the PHD finger, GmPHD6 associates with LHP1, probably at the CSD in the C-terminal end. GmPHD6 reads the histone code and recognizes the G-rich elements in the target gene promoter, whereas LHP1 functions as a transcriptional activator. In this way, the two partners form a transcriptional activation complex to activate target gene expression for stress tolerance in soybean. Our study sheds light on the mechanism of Alfin1-like PHD proteins in stress response. Manipulation of the complex and/or target genes may improve abiotic stress tolerance in soybean and/or other legumes/crops.
MATERIALS AND METHODS
Plant Materials
The soybean cultivar Kefeng1 (Glycine max, KF1) was used for hairy root transformation. Seeds were germinated on vermiculate soil at 28°C and grown for one week before infection.
Yeast Two-Hybrid Assay
A yeast two-hybrid assay was performed following the method described in the Matchmaker Gold yeast two-hybrid system (Clontech). The coding sequence of GmPHD6 was cloned into the BamHI/SalI sites of pGBKT7 and the library of soybean cDNAs were inserted into the pGADT7 vector. The bait vector was transfected into the yeast strain Y2HGold and the library vector was transfected into the yeast strain Y187. After being tested for autoactivation (BD-GmPHD6 has no autoactivation activity) and toxicity, the bait strain was combined with the library and slowly (at 50 rpm) shaken at 30°C for 20 h to 24 h. The mated yeast strains were grown on QDO/X/A (SD/-Ade/-His/-Leu/-Trp/X-a-Gal/AbA) plates for 3 d and blue colonies indicated positive interactions. Plasmids of these positive clones were extracted and sequenced.
Agrobacterium rhizogenes-mediated Transformation of Soybean Hairy Roots and Stress Treatments
For overexpression vector construction, the coding sequences of GmPHD6 (Glyma.09G068500), LHP1-1 (Glyma.16G079900), and LHP1-2 (Glyma.03G094200) were cloned into the BamHI/KpnI sites of the pROKII vector (http://www.arabidopsis.org/). For RNAi vector construction, the sense fragments of GmPHD6, LHP1-1, and LHP1-2 were cloned into the SacI/KpnI sites of pZH01, and the antisense fragments were cloned into the XbaI/SalI sites. The hypocotyls of one-week-old soybean seedlings (KF1) were injected with A. rhizogenes strain K599 harboring the pROKII or pZH01 construct following a method in the literature (Kereszt et al., 2007). For cotransformation, A. rhizogenes harboring 35S-GmPHD6 and LHP1-RNAi or 35S-GmPHD6 and 35S-LHP1-1 was mixed in equal amounts and injected to the hypocotyls of soybean seedlings. The infected seedlings were kept in high humidity for about two weeks until the hairy roots were generated from the wounded sites. The original roots were cut and the seedlings were moved to water for recovery for 3 d. Seedlings with hairy roots of 2 cm to 10 cm were transferred to 100 mm NaCl for 3 d as stress treatments. Leaf phenotypic change was observed and root length was measured before and after the treatment. The relative root growth rate (RGR) was calculated to determine the performance of hairy roots: RGR (%) = (average root elongation in stress) / (average root elongation in water). Relative electrolyte leakage and relative water content were measured to determine the performance of soybean leaves. After salt treatment, all the trifoliates were cut and dried at 60°C for at least 2 d to measure water content: RWC (%) = (water content after salt treatment) / (water content in water).
Quantitative RT PCR
To detect gene expression in transgenic hairy roots, we harvested transgenic hairy roots from 10 to 15 plants for RNA extraction and gene expression analysis. A soybean tubulin fragment was used as an internal control.
Relative Electrolyte Leakage Assay
After treatment in 100 mm NaCl for 3 d, the second trifoliate from the top was cut for the relative electrolyte leakage assay. Soybean leaves were immersed in water, vacuumed for 45 min, and placed at room temperature for 2 h. Conductivity (K1) was then measured. After this, the leaves were autoclaved for 15 min. The samples were shaken at 200 rpm until water temperature dropped to room temperature. Conductivity (K2) was measured again. Relative electrolyte leakage (%) = K1/K2.
Protein Expression and GST or H3 Peptide Pull-down Assay
The coding regions for full-length or various domains of GmPHD6 were inserted into the BamHI/SalI sites of the pGEX4T-1 vector (GE Healthcare) to generate GST fusion proteins. The coding sequences of LHP1-1 and LHP1-2 were cloned into the EcoRI/SalI sites of pMAL-c2x vector to generate MBP fusion proteins. The constructed plasmids were transformed into the Escherichia coli BL21 strain. GST fusion proteins were purified using glutathione Sepharose 4B (GE Healthcare). MBP fusion proteins were purified using amylase resin (New England Biolabs).
For the GST pull-down assay, GST fusion proteins were bound to glutathione Sepharose 4B beads for 1 h at room temperature in PBS buffer (137 mm NaCl, 2.7 mm KCl, 10 mm Na2HPO4, 2 mm KH2PO4, pH 7.4). MBP fusion proteins were then added to the beads and incubated at 4°C overnight. The beads were washed twice with high-salt-washing buffer (25 mm Tris pH 8.0, 10% glycerol, 1 mm EDTA, 500 mm NaCl, 1 mm PMSF, 1 mm DTT, 1% Triton X-100) and six times with low-salt-washing buffer (25 mm Tris pH 8.0, 10% glycerol, 1 mm EDTA, 150 mm NaCl, 1 mm PMSF, 1 mm DTT, 1% Triton X-100). The beads were collected and boiled with SDS loading buffer for 10 min. The eluted proteins were electrophoresed on 4 to 20% SDS PAGE and transferred to a PVDF membrane (Millipore). Anti-GST or Anti-MBP (Abmart) antibody was used as the primary antibody and then goat anti-mouse IgG-HRP (Abmart) was used as the second antibody. The western-blotting signal was detected using a SuperSignal West Pico Chemiluminescent Substrate (Thermo Fisher Scientific).
For the H3 peptide pull-down assay, GST or MBP or their fusion proteins were incubated with 1 μg biotin-conjugated H3 peptide (12-403 for H3, 12-563 for H3K4me, 12-460 for H3K4me2, 12-564 for H3K4me3; Millipore) in binding buffer (50 mm Tris pH 7.5, 300 mm NaCl, 0.1% NP-40) at 4°C overnight. Dynabeads M-280 Streptavidin (Invitrogen) was then applied and incubated for 1 h at 4°C. The beads were washed twice with high-salt-washing buffer and six times with low-salt-washing buffer, as described above, and boiled with SDS-loading buffer. The western blotting described above was performed to detect the eluted proteins.
Co-IP Assay
The coding sequence of GmPHD6 was cloned into a pGWB412 vector to express a Flag-GmPHD6 protein. The coding sequence of LHP1-1 or LHP1-2 was cloned into a pGWB420 vector to express LHP1-MYC proteins. Plasmids were transformed into Agrobacterium tumefaciens strain GV3101. A. tumefaciens harboring pGWB412, pGWB420, and p19 (gene-silencing suppressor) were coinjected into tobacco (Nicotiana tabacum) leaves. After growth at 25°C for 3 d, tobacco leaves were harvested and ground in liquid nitrogen to a fine powder. Nucleus was extracted with nuclei isolation buffer (0.25 m Suc, 15 mm PIPES pH 6.8, 5 mm MgCl2, 60 mm KCl, 15 mm NaCl, 1 mm CaCl2, 0.9% Triton X-100, 1 mm PMSF) and lysed with nuclei lysis buffer (50 mm HEPES pH 7.5, 150 mm NaCl, 1 mm EDTA, 0.1% sodium deoxycholate, and 1% Triton X-100) with protease and phosphatase inhibitors (Thermo Fisher Scientific). A Co-IP assay was performed using a c-MYC-Tag IP/Co-IP Kit (Thermo Fisher Scientific), and was detected by western blotting.
DNA Binding Assay
Single-stranded complementary fragments containing 4× GTGGAG elements were synthesized and labeled with biotin at their 5′-end (Invitrogen). dsDNA probes were obtained by heating complementary fragments at 75°C for 5 min, then slowly cooling at room temperature. The gel-shift assays were performed using the LightShift Chemiluminescent EMSA Kit (Thermo Fisher Scientific).
Stomatal Closure Measurement
The soybean plants were kept in 100 mm NaCl for 1 h and the second trifoliate from the top was chosen for stoma observation. Soybean plants kept in water were used as control samples.
Transcriptional Activation Assay
The transcriptional activation assay was performed using Arabidopsis (Arabidopsis thaliana) protoplast (Yoo et al., 2007) and a Dual-Luciferase Reporter Assay System (Ohta et al., 2001). A pUC19 vector containing five GAL4 binding elements and a firefly LUC gene was used as a reporter plasmid, and a pTRL vector, including a Renilla LUC gene, was used as an internal control. For the transactivation assay of a single gene, coding sequences of GmPHD6, LHP1-1, and LHP1-2 were cloned into a pRT-BD vector and a pRT-BD-VP16 was used as a positive control. For the transactivation assay of a GmPHD6/LHP1 complex, LHP1-1 and LHP1-2 were cloned into a pRT107 vector driven by a 35S promoter (kindly provided by Professor Yan Guo, China Agricultural University). For the promoter-LUC assay, the GAL4 binding elements and TATA-box of the reporter plasmid, in front of a LUC gene, were replaced by the promoter regions of downstream genes. The BD-coding sequence of pRT-BD-VP16 was replaced by GmPHD6 to generate 35S-G6-VP16. A luminescence reader was used to detect the LUC signal (GloMax 20-20 Luminometer; Promega).
RNA Extracting and Sequencing
Transgenic hairy roots were harvested for RNA extraction using the TRNzol Reagent (Tiangen). The RNA-sequencing was performed by a vendor (1gene) using the HiSeq 2500 (Illumina). Each sample contained two replicates. The adaptor sequence and low-quality reads were deleted and the clean reads were mapped to a soybean genome (https://phytozome.jgi.doe.gov/) using the software TopHat (http://ccb.jhu.edu/software/tophat/). Differentially expressed genes were analyzed using the software Cufflinks (http://cole-trapnell-lab.github.io/cufflinks/). Up- or down-regulated transcripts, with fold change ≥ 2, were examined for possible downstream genes using an on-line Venn diagram tool (http://bioinfogp.cnb.csic.es/tools/venny/index.html). Quantitative RT-PCR was performed to test the results of RNA-Seq using RNA extracted from independently generated hairy roots.
Accession Numbers
Sequence data from this article can be found in the Gene Expression Omnibus under accession numbers GSE85077.
Supplemental Data
The following supplemental materials are available.
Supplemental Figure S1. Organ-specific expression of GmPHD6, LHP1-1, and LHP1-2.
Supplemental Figure S2. Effector and reporter constructs used in the dual-luciferase reporter assay system.
Supplemental Figure S3. Performance of LHP1-transgenic hairy roots in response to stress.
Supplemental Figure S4. Nucleotide sequence alignment of LHP1-1 and LHP1-2 regions coding for the protein C-terminal end.
Supplemental Figure S5. Analysis of RNA-sequencing data and quantitative RT-PCR.
Supplemental Figure S6. Expression of ABA signaling-related genes and CCDs.
Supplemental Table S1. Statistics of clean reads in RNA sequencing.
Supplemental Table S2. Annotation of downstream genes.
Supplemental Table S3. Common genes were defined as genes that were up-regulated in G6-OE and down-regulated in G6-RNAi.
Supplemental Table S4. Primers used in this study.
LITERATURE CITED
Author notes
This work is supported by the National Key Research and Development Program of China (under grant no. 2016YFD0100304), the 973 Project (under grant no. 2015CB755702), the Transgenic Research Project (under grant nos. 2016ZX08004002-003 and 2016ZX08009003-002), the National Natural Science Foundation of China (under grant no. 31530004), the Strategic Priority Research Program of the Chinese Academy of Sciences (under grant no. XDA08020106), and the State Key Lab of Plant Genomics.
Address correspondence to [email protected] or [email protected].
The authors responsible for the distribution of materials integral to the findings presented in this article in accordance with the policy described in the instructions for authors are Jin-Song Zhang ([email protected]) and Shou-Yi Chen ([email protected]).
W.W. performed experiments and drafted the initial manuscript; J.-J.T., H.-W.C., and Q.-T.L. gave assistance in some experiments; W.-K.Z., B.M., and Q.L. performed data analysis; J.-S.Z. and S.-Y.C. conceived projects, designed experiments, and revised the paper.
Articles can be viewed without a subscription.