-
PDF
- Split View
-
Views
-
Cite
Cite
Yohei Takahashi, Yuta Ebisu, Ken-ichiro Shimazaki, Reconstitution of Abscisic Acid Signaling from the Receptor to DNA via bHLH Transcription Factors , Plant Physiology, Volume 174, Issue 2, June 2017, Pages 815–822, https://doi.org/10.1104/pp.16.01825
- Share Icon Share
Abstract
The plant hormone abscisic acid (ABA) confers drought tolerance in plants through stomatal closure and regulation of gene expression. The complex consisting of the ABA receptor PYRABACTIN RESISTANCE/REGULATORY COMPONENTS OF ABA RECEPTOR (PYR/RCAR), type 2C protein phosphatase (PP2C), and SNF1-related protein kinase 2 (SnRK2) has a key role in ABA signaling. Basic helix-loop-helix (bHLH) transcriptional activator ABA-RESPONSIVE KINASE SUBSTRATE1 (AKS1, also known as FBH3) is released from DNA by phosphorylation-induced monomerization in response to ABA in guard cells. Here we reconstituted the release of AKS1 from DNA via the ABA signaling core complex in vitro. We first obtained evidence to confirm that AKS1 is an endogenous substrate for SnRK2s. Phosphorylation of AKS1 and activation of SnRK2 showed the same time course in response to ABA in guard cells. AKS1 was bound to SnRK2.6 in vivo. Three ABA-responsive SnRK2s (SnRK2.2/SRK2D, SnRK2.3/SRK2I, and SnRK2.6/SRK2E/OST1) phosphorylated AKS1 in vitro, and the phosphorylation was eliminated by the triple mutation of SnRK2s in plants. We reconstituted the AKS1 phosphorylation in vitro via the signaling complex containing the ABA receptor PYR1, a PP2C, HYPERSENSITIVE TO ABA1 (HAB1), and a protein kinase, SnRK2.6 in response to ABA. We further reconstituted the release of AKS1 from the target gene of POTASSIUM CHANNEL IN ARABIDOPSIS THALIANA 1 (KAT1) via the complex in response to ABA. These results demonstrate that AKS1 provides a link between the signaling complex and ABA-responsive genes and furnish evidence for a minimal signaling mechanism from ABA perception to DNA.
Plants respond and integrate a wide variety of external and internal stimuli, including light, drought, and plant hormones, and coordinate their growth with developmental and environmental adaptations. They develop signaling networks that transduce these stimuli into molecular responses, including gene regulation in the cells. Thus, transmission of intracellular signals specific to the stimuli to their target genes is critical for plant cell function.
The plant hormone abscisic acid (ABA) plays a central role in the adaptation of plants to drought stress (Finkelstein, 2013). ABA induces stomatal closure to prevent water loss by transpiration and enhances or reduces the expression of numerous genes to aid the plants in adapting to such adverse conditions (Kim et al., 2010; Munemasa et al., 2015). The signaling core complex perceives ABA signal and transmits it to downstream components that induce distinct cellular events (Cutler et al., 2010; Raghavendra et al., 2010; Umezawa et al., 2010). Previous studies have shown that the ABA receptor PYRABACTIN RESISTANCE/REGULATORY COMPONENTS OF ABA RECEPTOR (PYR/RCAR) inhibits type 2C protein phosphatases (PP2Cs) that function as negative regulators for ABA signaling in the core complex in response to ABA (Ma et al., 2009; Melcher et al., 2009; Miyazono et al., 2009; Nishimura et al., 2009; Park et al., 2009). Upon inhibition of PP2C, the SNF1-related protein kinases 2 (SnRK2) are activated. These activated SnRK2s phosphorylate their substrates, such as SLOW ANION CHANNEL-ASSOCIATED 1 (SLAC1) anion channel in the plasma membrane, and basic region/Leu zipper motif (bZIP) type transcription factors of ABA-responsive element (ABRE)-binding proteins (AREBs)/ABRE-binding factors (ABFs; Yoshida et al., 2006; Fujii et al., 2009; Geiger et al., 2009; Lee et al., 2009; Umezawa et al., 2009; Vlad et al., 2009; Kline et al., 2010).
The AREB/ABF gene family, which is the best-known transcription factor family in ABA signaling, is conserved among many species, including rice (Oryza sativa), wheat (Triticum aestivum), barley (Hordeum vulgare), and tobacco (Nicotiana tabacum), as well as Arabidopsis (Arabidopsis thaliana; for reviews, see Yamaguchi-Shinozaki and Shinozaki, 2006; Fujita et al., 2013). The simultaneous mutation of three redundant AREB/ABF genes in Arabidopsis resulted in a drought-sensitive phenotype (Yoshida et al., 2010, 2015). AREB/ABFs are activated by phosphorylation (Furihata et al., 2006); therefore, it is thought that ABA induces gene expression through phosphorylated AREB/ABFs via the signaling core complex. Previously, AREB1 (also known as ABF2) phosphorylation was reconstituted in vitro using these recombinant proteins, and the direct phosphorylation of AREB1 through the minimal core complex has been demonstrated (Fujii et al., 2009). Phosphorylation of AREB/ABFs is suggested to enhance protein stability (Sirichandra et al., 2010), but some studies using plants overexpressing AREB1 pointed to the presence of other processes required for AREB/ABFs activation (Fujita et al., 2005; Furihata et al., 2006). It is possible that AREB/ABFs become bound to specific DNA containing ABRE cis-elements through phosphorylation, thereby inducing the expression of ABA-responsive genes. However, there is no direct evidence for these responses at the biochemical level.
We reported that basic helix-loop-helix (bHLH) transcription factors, named ABA-responsive kinase substrates (AKSs), were phosphorylated in response to ABA in Arabidopsis guard cells (Takahashi et al., 2013). AKS1 facilitates stomatal opening by modulating gene expression, including that of the plasma membrane K+ channel POTASSIUM CHANNEL IN ARABIDOPSIS THALIANA 1 (KAT1) gene that regulates K+ uptake in guard cells in the absence of ABA. We showed that ABA causes the inhibition of KAT1 expression by release of AKS1 from the KAT1 gene, thereby limiting stomatal opening, and the release is elicited by the monomerization of AKSs through phosphorylation (Takahashi et al., 2013, 2016). The stomatal response mediated by the AKS1 release is likely a late process in response to ABA. For example, the inhibition of KAT1 expression may reduce the reopening of stomata in plants that had experienced severe drought. Furthermore, previous studies provided evidence that the phosphorylation of AKSs was eliminated in both snrk2.2/2.3/2.6 (also known as srk2dei) triple mutant and PP2C dominant mutant, suggesting that AKSs were phosphorylated downstream of the signaling core complex (Takahashi et al., 2013; Umezawa et al., 2013; Wang et al., 2013). It was also reported that SnRK2.6 (also known as SRK2E/OST1) phosphorylates AKS1 in vitro (Takahashi et al., 2013; Wang et al., 2013), supporting the functional role of AKSs as substrates of SnRK2 in vivo. Therefore, showing the reconstitution of AKS phosphorylation in the signaling core complex in response to ABA will strengthen our previous findings.
Besides AREB/ABFs and AKSs, many other transcription factors play roles in ABA signaling and forming the network to regulate gene expression (Yamaguchi-Shinozaki and Shinozaki, 2006). Systematic study on transcription factors highlighted the dynamic changes in the ABA-dependent binding of transcription factors to DNA. Among these, AKS1 exhibited a unique behavior that seemed to show dissociation from DNA in response to ABA (Takahashi et al., 2013; Song et al., 2016). A positive relationship between the binding of transcription factors to DNA and gene expression appeared to exist (Song et al., 2016). However, how ABA regulates this binding of transcription factors to DNA remained unknown.
In this study, we showed that AKSs are endogenous substrates of SnRK2. We then reconstituted AKS1 phosphorylation in response to ABA in vitro using recombinant proteins of PYR1, HYPERSENSITIVE TO ABA1 (HAB1), and SnRK2.6 and demonstrated the release of AKS1 from the KAT1 promoter through AKS1 phosphorylation. This is the first reconstituted signaling complex that connects the perception of an ABA signal to the target DNA via the phosphorylation of a transcription factor.
RESULTS
SnRK2.6 Phosphorylates AKS1 in Guard Cells
We determined the activity of SnRK2.6 by in-gel kinase assay with histone as a substrate and the phosphorylation of AKSs by protein blot assay in response to ABA using guard cell protoplasts of Arabidopsis. We found that both reactions were initiated 2 minutes after the addition of 10 µm ABA to guard cell protoplasts and reached maximum within 10 minutes (Fig. 1A). The results suggest that the timeframe in which these reactions take place is similar. To see whether the ABA-activated SnRK2.6 directly phosphorylates AKS1, the in-gel kinase assay was performed using recombinant AKS1 protein as a substrate. A band was visualized at 44 kD, the molecular mass of SnRK2.6, in the extract from wild-type guard cell protoplasts in response to ABA, while the band was not found in the extract from snrk2.6 knockout plants, indicating that the ABA-activated SnRK2.6 phosphorylated AKS1 (Fig. 1B).
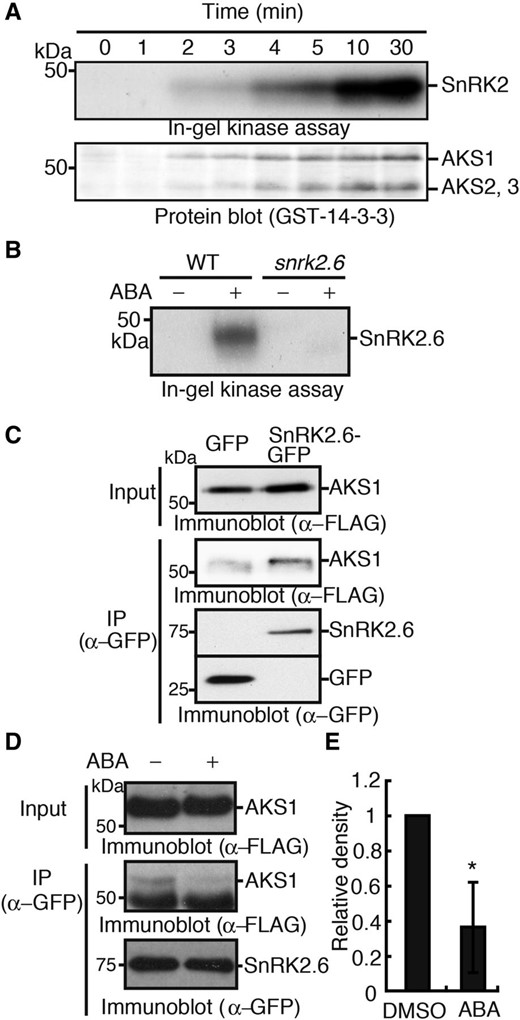
AKS transcription factors interact with and are phosphorylated by SnRK2.6. A, Time courses of activation of SnRK2 and phosphorylation of AKSs in guard cells of Arabidopsis in response to ABA. Guard cell protoplasts from Arabidopsis were incubated with ABA at 10 µm for indicated times. SnRK2 activity and AKSs phosphorylation were determined by in-gel kinase assay with histone and protein-blot analysis with a 14-3-3 protein, respectively. B, ABA-activated SnRK2.6 phosphorylated AKS1. In-gel kinase assay using AKS1 as an in-gel substrate was performed with guard cell protoplasts from Arabidopsis treated with or without ABA at 10 µm for 10 min. C, AKS1 was bound to SnRK2.6 in vivo. SnRK2.6-GFP and FLAG-AKS1 were expressed in mesophyll cell protoplasts from Arabidopsis by a PEG-mediated method. SnRK2.6-GFP was immunoprecipitated by anti-GFP antibodies and the coprecipitated FLAG-AKS1 was detected by immunoblotting with an anti-FLAG antibody. D, Effect of ABA on the interaction between SnRK2 and AKS1. ABA at 10 µm was administered to mesophyll cell protoplasts expressing SnRK2.6-GFP and FLAG-AKS1, and the suspension was incubated for 15 min. Then, SnRK2.6-GFP was immunoprecipitated by GFP antibody. The large band below FLAG-AKS1 that appeared when SDS-PAGE was done at the low concentrations of acryl amide seems to be nonspecific. E, The relative amount of AKS1 coimmunoprecipitated with SnRK2.6-GFP was quantified by ImageJ 1.42q. Values are means ± sd (n = 3, *P < 0.05, unpaired Student’s t test). Each experiment was repeated at least three times on different occasions and gave similar results.
AKS1 Is an Endogenous Substrate of SnRK2
Next, we determined the protein-protein interaction between AKS1 and SnRK2.6 in plants. Both SnRK2.6-GFP and FLAG-AKS1 were transiently expressed in Arabidopsis mesophyll cell protoplasts (Yoo et al., 2007), and SnRK2.6-GFP was immunoprecipitated with antibodies against GFP. We found that FLAG-AKS1 coprecipitated with SnRK2.6-GFP, while much less coprecipitation was detected with GFP (Fig. 1C), suggesting that AKS1 was bound to SnRK2.6 in plant cells. We tested the effect of ABA on the interaction between AKS1 and SnRK2.6. Mesophyll cell protoplasts expressing both SnRK2.6-GFP and FLAG-AKS1 were treated with 10 µm ABA for 15 min, and SnRK2.6-GFP was immunoprecipitated. The amount of coprecipitated AKS1 with SnRK2.6 was reduced by 60% (Fig. 1, D and E). We note that the large band below FLAG-AKS1 seems to be nonspecific. Mobility of the smear band in Figure 1D is slightly different from that in Figure 1C. A difference in acrylamide concentrations (10.5% in Fig. 1C and 7.5% in Fig. 1D) might affect the band mobility. From these results, we conclude that AKS1 is an endogenous substrate of SnRK2, and ABA likely reduces the interaction between AKS1 and SnRK2.6 in vivo.
Three SnRK2s Redundantly Phosphorylate AKSs
Three ABA-responsive SnRK2 isoforms function redundantly in Arabidopsis. The triple knock-out plants of these isoforms were found to exhibit dramatic insensitivity to ABA, but the double and single mutants showed less ABA sensitivity than the triple mutants (Fujii and Zhu 2009; Fujita et al., 2009; Nakashima et al., 2009; Umezawa et al., 2009). We tested whether these three SnRK2s also redundantly phosphorylate AKSs. All three recombinant SnRK2 kinases (SnRK2.2, SnRK2.3, and SnRK2.6) were able to phosphorylate AKS1 in vitro (Fig. 2A) and bind AKS1 as shown by an in vitro pull-down assay (Fig. 2B). We next measured the ABA-induced phosphorylation of AKSs in snrk2.6 single, snrk2.2/2.3 double, and snrk2.2/2.3/2.6 triple mutants (Umezawa et al., 2009). Protein-blot analysis showed that the phosphorylation of endogenous AKSs in snrk2.6 and snrk2.2/2.3 mutants was similar to that in wild type, while no phosphorylation was seen in the triple mutant (Fig. 2C), providing evidence that the three SnRK2s redundantly phosphorylate AKSs in response to ABA in plants.
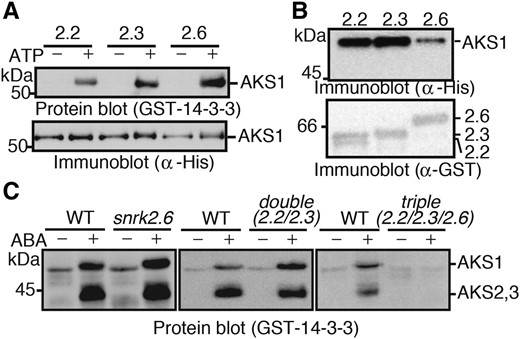
Three ABA-responsive SnRK2s function redundantly to phosphorylate AKSs. A, In vitro phosphorylation of AKS1 by recombinant proteins of SnRK2.2, SnRK2.3, and SnRK2.6. His-AKS1 and GST-SnRK2s were incubated with or without ATP for 30 min, and AKS1 phosphorylation was detected by protein-blot analysis. B, In vitro pull-down of AKS1 by SnRK2s. GST-SnRK2s were incubated with His-AKS1 and collected by glutathione sepharose beads. Coprecipitated His-AKS1 was detected by immunoblotting with an antibody against His-tag. C, Phosphorylation of AKS1 in snrk2 mutant plants. Guard cell protoplasts from snrk2.6, snrk2.2/2.3, or snrk2.2/2.3/2.6 mutants were incubated with or without 10 µm ABA for 10 min. Phosphorylation of AKSs was detected by protein-blot analysis. Each experiment has been repeated at least three times with similar results.
Inhibitory Effect of HAB1 on SnRK2.6-Mediated AKS1 Phosphorylation
Activity of SnRK2 is repressed by dephosphorylation through PP2C in the signaling core complex in the absence of ABA, which prevents substrate phosphorylation by SnRK2 (Umezawa et al., 2009; Vlad et al., 2009). PP2C also dephosphorylates one of the SnRK2 substrates, SLAC1 (Brandt et al., 2012, 2015). To understand whether phosphorylation of AKSs is suppressed by PP2C, the effect of HAB1 (Saez et al., 2004) on AKS1 phosphorylation was investigated. As expected, the recombinant HAB1 inhibited the SnRK2.6-mediated AKS1 phosphorylation in vitro when HAB1 was added before adding ATP (which initiates the kinase reaction) to the reaction mixture (Fig. 3A, lane 4). However, when HAB1 was added to the reaction mixture after the phosphorylation began, the phosphorylation levels of AKS1 were not affected (Fig. 3A, lane 6). As a control, we used the catalytic subunit of cAMP-dependent protein kinase (PKA) and showed that PKA phosphorylated AKS1 in vitro (Fig. 3B, lane 2). However, HAB1 did not inhibit this PKA-mediated AKS1 phosphorylation even when HAB1 was included in the mixture before the addition of ATP (Fig. 3B, lane 4). These results suggest that HAB1 prevents AKS1 phosphorylation through inhibition of SnRK2, but does not directly dephosphorylate AKS1.
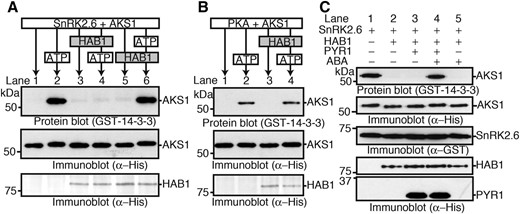
Inhibition of SnRK2.6-mediated AKS1 phosphorylation by HAB1 and in vitro reconstitution of ABA-induced AKS1 phosphorylation. A, In vitro phosphorylation of His-AKS1 by GST-SnRK2.6 in the presence of His-HAB1. The phosphorylation reaction was started by the addition of ATP. His-HAB1 was added 20 min before (lane 4) or 60 min after (lane 6) ATP addition. AKS1 phosphorylation was detected by protein-blot analysis. B, In vitro phosphorylation of AKS1 by PKA in the presence of HAB1. His-HAB1 was added 20 min before (lane 2) or 60 min after (lane 4) ATP addition. C, In vitro reconstitution of AKS1 phosphorylation in response to ABA using recombinant proteins of His-PYR1, His-HAB1, GST-SnRK2.6, and His-AKS1. SnRK2.6 phosphorylated AKS1 (lane1), and HAB1 inhibited the AKS1 phosphorylation (lane2). PYR1 inhibited the HAB1 effect on AKS1 phosphorylation in the presence of ABA (lanes 3 and 4). The ABA effect depended on PYR1 (lane 5). Phosphorylation of AKS1 was detected by protein-blot analysis. Each experiment was repeated at least three times with similar results.
Reconstitution of ABA-Induced AKS1 Phosphorylation in Vitro
Several studies have reported previously that the minimal core mechanisms of ABA signaling can be reconstituted in vitro, and that the core complex phosphorylates both AREB/ABFs and SLAC1 in response to ABA (Fujii et al., 2009; Brandt et al., 2012, 2015). On the basis of results obtained in this study, we attempted to reconstitute the ABA signaling system including AKS1 in vitro. We generated a recombinant ABA receptor protein PYR1 and utilized it for in vitro phosphorylation in combination with HAB1, SnRK2.6, and AKS1 proteins (Fig. 3C). We confirmed the phosphorylation of AKS1 by SnRK2.6 and suppression of the phosphorylation by HAB1 (Fig. 3C, lanes 1 and 2). Addition of PYR1 to these three components had no effect on the reactions, but we found that AKS1 was phosphorylated when ABA was added to the reaction mixture (Fig. 3C, lanes 3 and 4). AKS1 phosphorylation did not occur when PYR1 was omitted from the mixture even if ABA was included (Fig. 3C, lane 5). These results suggest that AKSs are phosphorylated in the signaling core complex in response to ABA in the same way as AREB/ABFs.
HAB1 Inhibits the Release of AKS1 from KAT1 Gene
We previously showed that the binding capacity of AKS1 to DNA was reduced by monomerization of AKS1s through their phosphorylation by SnRK2 (Takahashi et al., 2016). In this study, we tested the effect of HAB1 on AKS1 binding. For this purpose, we produced biotinylated DNA fragments derived from the KAT1 genes and incubated the DNA fragments with recombinant AKS1 proteins. After incubation, we collected the biotinylated KAT1 fragments by binding them to streptavidin-coupled magnetic beads and determined the amount of coprecipitated AKS1 protein by immunoblot analysis. We confirmed the release of AKS1 from DNA by the addition of ATP in the presence of SnRK2.6 (Fig. 4A). To show that the release of AKS1 from the KAT1 fragment is mediated by SnRK2.6 activity, we included staurosporine, an inhibitor of protein kinases, in the reaction mixture showing that staurosporine inhibited the release (Fig. 4A). We then examined the effect of HAB1 on SnRK2.6-mediated AKS1 release and found that HAB1 completely inhibited the release from KAT1 (Fig. 4B).
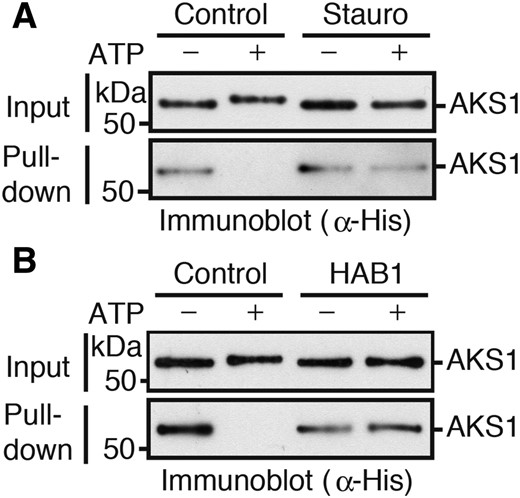
HAB1 suppresses the SnRK2.6-mediated AKS1 release from KAT1 gene. A, In vitro DNA-protein pull-down assay using recombinant His-AKS1, GST-SnRK2.6, and KAT1 gene fragments. The biotin-conjugated KAT1 gene fragment was collected with the streptavidin-coupled beads after 30 min incubation with ATP, and coprecipitated His-AKS1 protein was then detected by immunoblot analysis. A protein kinase inhibitor staurosporine (Stauro) was used to examine the effect of phosphorylation on the DNA-binding ability. B, Effect of HAB1 on AKS1 DNA-binding was examined by in vitro DNA-protein pull-down assay. HAB1 and GST-SnRK2.6 were added to the reaction before the addition of ATP. Each experiment was repeated at least three times with similar results.
Reconstitution of the Signaling from ABA Perception to KAT1 Gene via AKS
Our above results implied the presence of a new signaling framework in which the ABA signaling core complex induces the release of AKS transcription factors from DNA by phosphorylation. To test this model, we attempted to reconstitute ABA signaling in vitro from ABA perception to DNA. We used recombinant proteins of PYR1, HAB1, SnRK2.6, and AKS1, with KAT1 gene fragments as DNA, and then performed the DNA-protein pull-down assay. We mixed these components with the DNA fragments and incubated in the absence or presence of ABA, and confirmed the phosphorylation of AKS1 in response to ABA in the mixture (Fig. 5A, protein blot). We then collected the KAT1 fragments and determined the amount of AKS1 bound to the DNA fragments. We found that the AKS1 bound to KAT1 was greatly reduced by ABA (Fig. 5A, bottom, and 5B), indicating that ABA induces the release of AKS1 from the KAT1 gene through phosphorylation in vitro.
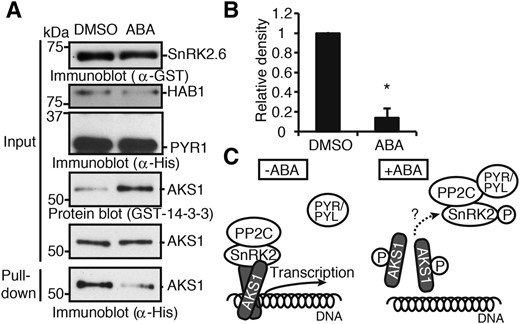
Reconstitution of the release of AKS1 from KAT1 gene in response to ABA in vitro. A, In vitro DNA-protein pull-down using His-AKS1 and biotin-conjugated KAT1 gene fragments in the presence of recombinant proteins of His-PYR1, His-HAB1, and GST-SnRK2.6. The KAT1 fragments were collected with the streptavidin-coupled beads after 30 min incubation with or without 100 µm ABA. Phosphorylation of AKS1 and the amount of AKS1 bound to the KAT1 gene fragment were determined by protein-blot and immunoblot analyses, respectively. B, The relative amounts of AKS1 bound to the KAT1 gene fragment. Relative intensity of immunoblot bands of His-AKS1 in DNA-protein pull-down assay was quantified by ImageJ 1.42q. Values are means ± sd (n = 3, *P < 0.01, unpaired Student’s t test). C, A model for transcription inhibition by release of AKS1 from a target gene in response to ABA. AKS1 is bound to both SnRK2 and its target gene in the nucleus. AKS1 promotes the transcription of target genes including KAT1 in the absence of ABA. SnRK2 is activated and phosphorylates AKS1 in response to ABA, and the phosphorylation causes monomerization of AKS1. The monomerized AKS1 is released from DNA, resulting in the repression of transcription. AKS1 may also dissociate from SnRK2.6 after phosphorylation.
DISCUSSION
We recently showed that the activity of bHLH transcriptional factor AKS1 is inhibited by ABA through phosphorylation-dependent release of AKS1 from the target DNA in guard cells (Takahashi et al., 2013; Takahashi et al., 2016). Our experiments here reproduce the ABA-induced release of AKS1 via the signaling core complex in vitro. ABA-induced phosphorylation of AREB1, the bZIP transcription factor that enhances the expression of ABA-responsive genes, has been reconstituted in vitro in the signaling core complex (Fujii et al., 2009); however, the signaling from AREB1 to the target genes is yet to be established. Here we reconstituted ABA signaling in vitro from the receptor to a target gene, using PYR1, HAB1, SnRK2.6, AKS1, and KAT1 gene fragments as a minimal set of signaling. We suggested that phosphorylation of AKS1 results in the release of the core complex from DNA (Fig. 5C). AKS1 seems to act as a link between the core complex and target DNA and plays a key role in converting ABA signal to regulate gene expression through its phosphorylation.
Since our previous results showed that the ABA-dependent inhibition of KAT1 expression is mediated by AKS1 phosphorylation (Takahashi et al., 2016), these new results provide a relationship between gene expression and protein phosphorylation of AKS1 transcription factor in response to ABA. However, unlike AKS1, which reduces gene expression, AREB/ABFs likely enhance gene expression through phosphorylation in response to ABA. Phosphorylation seems to have opposing effects on the function of AKS1 and AREB/ABFs. Although AREB1, AREB2, and ABF3 form homo- and heterodimers in plant cells (Yoshida et al., 2010), the role of dimerization or the DNA-binding ability of AREB/ABFs has not yet been elucidated with respect to phosphorylation. The N-terminal region of AREB1 is essential for transcriptional activity. An internal inhibitory domain of the AREB1 appears to play an important role in the activation of AREB1 through a conformational change in response to ABA (Fujita et al., 2005). Five phosphorylation sites in AREB1 are distributed across the full-length of AREB1 protein (Furihata et al., 2006), while three phosphorylation sites in AKS1 exist in a clustered state close to the bHLH domain, which facilitates dimerization (Takahashi et al., 2016). These observations imply that AREB/ABFs appear to have different regulatory mechanisms from AKSs with respect to DNA binding. Further investigation is clearly needed to elucidate the mechanisms that lead to such differences.
Components of the core complex exist in the nucleus, cytosol, and plasma membrane in Arabidopsis cells (Rodriguez et al., 2014; Geiger et al., 2009; Lee et al., 2009; Umezawa et al., 2009; Park et al., 2009). PYR/RCAR, PP2C, and SnRK2 likely make a core complex in these distinct sites. ABA modulates both ion channel activity and gene expression in guard cells. Previous studies suggested that ABA-dependent activation of anion channels occurs within a nanodomain in the plasma membranes (Demir et al., 2013). By contrast, it remains unclear where the ABA signal is perceived and how the signal is transmitted to the nucleus in the ABA signaling pathway for gene regulation. Our present results demonstrated that ABA-induced AKS1 release from the KAT1 gene can be reconstituted in vitro (Fig. 5A) and also showed that AKS1 is bound to SnRK2.6 in plant cells (Fig. 1C). Since AKS1 predominantly localizes in the nucleus and is bound to DNA (Takahashi et al., 2013), our results suggest that the ABA signaling core complex also resides adjacent to DNA through AKSs. From these observations, we suggest that ABA signal transduction, from ABA perception to KAT1 transcription inhibition, is likely achieved within the nucleus in guard cells.
Stomatal movement in response to ABA can be categorized as at least two temporally distinct events. Rapid events occur within seconds to promote stomatal closure through physiological cellular responses, including Ca2+ elevation and regulation of ion channels and pump activities by protein phosphorylation and dephosphorylation (Munemasa et al., 2015). Slow events are thought to inhibit stomatal reopening in the late periods after the increase in ABA concentration and are mediated by gene expression, ion channel modifications, and intracellular relocation of the transporter proteins (Sutter et al., 2006, 2007; Eisenach, et al., 2012). AKS1 dissociation from the KAT1 promoter reconstituted in this study most likely mediates late events through a decrease in the population of KAT1 proteins in the plasma membrane in coordination with KAT1 endocytosis in response to ABA (Sutter et al., 2007). Guard cells are shown to have a capability to create “stress memory” to maintain closed stomata, likely through the regulation of gene expression (Virlouvet and Fromm, 2015). Although ABA and SnRK2 kinases are considered to play an important role in this response, the molecular mechanisms are largely unknown. It is interesting to elucidate whether the AKS1 phosphorylation acts in the memory response.
Some important components that mediate AKS1-dependent responses in guard cells have not been identified in response to ABA. For example, the phosphatase(s) that dephosphorylates AKS1 is not identified. PP2Cs do not seem to directly dephosphorylate AKS1 (Fig. 3A). Dephosphorylation of AKS1 is likely responsible for recovering gene expression that is repressed through the AKS1 phosphorylation. Protein phosphatase 2A (PP2A) has been reported to dephosphorylate AKSs, with reduced KAT1 expression in a pp2a b'αβ double mutant (Bu et al., 2017). In coordination with AKS1, other components will be required to define the specificity and the expression of target genes, because bHLH transcription factors recognize very short sequences, the E-box (CAXXTG), which are widely distributed throughout the Arabidopsis genome (Toledo-Ortiz et al., 2003; Heim et al., 2003). Furthermore, numerous genes are repressed in a tissue-specific manner in response to ABA, and the degree of repression is variable and gene dependent (Leonhardt et al., 2004; Wang et al., 2011; Seki et al., 2002). Therefore, identification of additional regulatory components that modify the minimal signaling pathway reported here will advance our understanding of the precise gene regulation of ABA signaling.
MATERIALS AND METHODS
Preparation of Guard Cell Protoplasts
Guard cell protoplasts were prepared enzymatically (Ueno et al., 2005). Epidermal strips were obtained from Arabidopsis (Arabidopsis thaliana) rosette leaves by homogenizing them in the water with a Waring blender for 50 s and collected on nylon mesh (58 µm). The strips were washed with water and digested with first-step cellulase solution, containing 0.5% (w/v) cellulase R-10 (Yakult), 0.04% (w/v) macerozyme R-10 (Yakult), 0.1% (w/v) polyvinylpyrrolidone K-30, 0.2% (w/v) bovine serum albumin (BSA), 0.25 m mannitol, 1 mm CaCl2, and 10 mm MES-KOH, pH 5.4) for 1 h at 24°C. Attached mesophyll cells were removed from the strips in this step. The digested epidermal strips were collected on a 58-µm nylon mesh and again digested in second-step cellulase solution, containing 1.5% (w/v) cellulase RS (Yakult), 0.4% (w/v) macerozyme R-10, 0.2% (w/v) BSA, 0.4 m mannitol, and 1 mm CaCl2, pH 5.4 for 50 to 60 min at 27°C. The protoplasts were detached from the epidermis by pipetting using a glass pipet. Then, the epidermis was removed by filtering them through two layers of nylon mesh of 94 and 58 µm. The filtrate was passed through two layers of 10-µm nylon mesh to remove mesophyll protoplasts. Guard cell protoplasts were collected as a pellet after centrifugation at 600 g for 9 min and washed two times with 0.4 m mannitol containing 1 mm CaCl2. Protoplasts were checked under a microscope and stored in the same solution on ice until use.
In-Gel Kinase Assay and Protein-Blot Analysis for Guard Cell Protoplasts
Guard cell protoplasts of Arabidopsis were suspended in buffer (5 mm MES-KOH, 10 mm KCl, 1 mm CaCl2, 0.4 m mannitol at pH 6.0) and treated with ABA for indicated periods. After ABA treatments, the reactions were stopped by the addition of trichloroacetic acid at 16.7% (w/v), and the precipitated proteins were collected and washed twice with ice-cold acetone. The proteins obtained were dissolved in buffer for SDS-PAGE and heated at 95°C for 3 min. In-gel kinase assays and protein-blot analyses with a GST-14-3-3phi protein were performed as described previously (Takahashi et al., 2007). Histone (Fig. 1A) and recombinant His-AKS1 (Fig. 1B) were used at 0.25 mg/mL as in-gel kinase substrates.
Transient Gene Expression and Immunoprecipitation
Mesophyll cell protoplasts of Arabidopsis were isolated and used for transient gene expression by a PEG-mediated method (Yoo et al., 2007) with transient expression vectors of 35S:SnRK2.6-GFP and 35S:FLAG-AKS1. After 14 h incubation, protoplasts were harvested by centrifugation and disrupted by the addition of extraction buffer (50 mm MOPS-KOH, 2.5 mm EDTA, 100 mm NaCl, 0.1% Triton X-100, 10 mm NaF, 1 mm ammonium molybdate, 2 mm dithiothreitol (DTT), 1 mm PMSF, 100 mm leupeptin at pH 7.5). After 10 min incubation on ice, cell debris was removed by centrifugation at 14,000 rpm for 10 min. SnRK2.6-GFP was immunoprecipitated by adding polyclonal GFP antibodies (Invitrogen) and Dynabeads protein G (Invitrogen) to the supernatant, and the mixture was incubated for 2 h at 4°C. After the incubation, the beads were washed three times with TBS supplemented with 0.01% Triton X-100.
In Vitro Pull-Down Analysis
Pull-down by protein–protein interaction was performed using recombinant GST-SnRK2s and His-AKS1 with glutathione sepharose, as described previously (Takahashi et al., 2016). For pull-down by DNA-protein interaction, we used a biotin-conjugated 300-bp DNA fragment containing the AKS1 binding region of the KAT1 promoter that was amplified by PCR with indicated primers (5′biotin-TTACGCCTTTTCTTCACCATTG and CTTTTTGATGATCTCTAAAGACAAAG). Biotin-conjugated probe of 2.5 ng was bound to streptavidin-coupled beads (Invitrogen) and used for each pull-down assay. The DNA-protein pull-down was performed in DNA binding buffer (10 mm Tris-HCl, pH 7.5, 50 mm NaCl, 1 mm MgCl2, 0.5 mm EDTA, 0.1% Triton X-100, 0.1% BSA, and 2 mm DTT). Short dsDNA of the M13 primer sequence at 25 ng µL−1 was prepared from synthesized complementary oligonucleotides (GTAAAACGACGGCCAG and CTGGCCGTCGTTTTAC), and was added to the reaction mixtures to reduce nonspecific binding. After incubation at room temperature for 30 min, the beads were washed three times by TBS supplemented with 0.01% Triton X-100.
In Vitro Phosphorylation Assay
For the in vitro assay shown in Figure 2, His-AKS1 (at 20 ng µL−1) and GST-SnRK2s (at 2 ng µL−1) were incubated in phosphorylation buffer (50 mm Tris-HCl, 10 mm MgCl2, 1 mm EGTA, 0.1% Triton X-100, 1 mm DTT, pH 7.5) for 30 min at room temperature. For the reconstitution of ABA-dependent AKS1 phosphorylation in vitro, His-AKS1 (at 10 ng µL−1), GST-SnRK2.6 (at 5 ng µL−1), His-HAB1 (at 1 ng µL−1), and His-PYR1 (at 10 ng µL−1) were incubated in phosphorylation buffer (50 mm Tris-HCl, 10 mm MgCl2, 0.1% TritonX-100, 1 mm DTT, pH7.5) for 8 min followed by the addition of 100 µm ATP to initiate the phosphorylation reaction for 30 min with or without 100 µm ABA at 30°C. The reactions were stopped by the addition of SDS-PAGE sample buffer. For the reconstitution of ABA-dependent release of AKS1 from DNA in vitro, 45 µL of the reaction mixture used above was added to 155 µL of DNA binding buffer that contained the biotin-conjugated DNA probe bound to streptavidin-coupled beads. DNA-protein pull-down was performed as described above.
ACKNOWLEDGMENTS
We thank Drs Kazuo Shinozaki (RIKEN) and Taishi Umezawa (Tokyo University of Agriculture and Technology) for providing snrk2.2/2.3 and snrk2.2/2.3/2.6 triple mutants. We also thank Dr. Julian I. Schroeder (University of California, San Diego) for helpful comments on the manuscript. We thank Mr. Andrew Cooper (University of California, San Diego) for correction of linguistic errors throughout the manuscript.
Glossary
LITERATURE CITED
Author notes
This work was financially supported by JSPS KAKENHI grant no. 21227001 (to K.S.).
Present address: Cell and Developmental Biology Section, Division of Biological Sciences, University of California, San Diego, La Jolla, CA 92093.
Address correspondence to [email protected].
The author responsible for distribution of materials integral to the findings presented in this article in accordance with the policy described in the Instructions for Authors (www.plantphysiol.org) is: Yohei Takahashi ([email protected]).
Y.T. conceived the research plan and performed most of the experiments; Y.E. designed and performed some experiments; K.S. proposed the experimental design.
Articles can be viewed without a subscription.