-
PDF
- Split View
-
Views
-
Cite
Cite
Alfredo Ambrosone, Giorgia Batelli, Roberta Nurcato, Vincenzo Aurilia, Paola Punzo, Dhinoth Kumar Bangarusamy, Ida Ruberti, Massimiliano Sassi, Antonietta Leone, Antonello Costa, Stefania Grillo, The Arabidopsis RNA-Binding Protein AtRGGA Regulates Tolerance to Salt and Drought Stress , Plant Physiology, Volume 168, Issue 1, May 2015, Pages 292–306, https://doi.org/10.1104/pp.114.255802
- Share Icon Share
Abstract
Salt and drought stress severely reduce plant growth and crop productivity worldwide. The identification of genes underlying stress response and tolerance is the subject of intense research in plant biology. Through microarray analyses, we previously identified in potato (Solanum tuberosum) StRGGA, coding for an Arginine Glycine Glycine (RGG) box-containing RNA-binding protein, whose expression was specifically induced in potato cell cultures gradually exposed to osmotic stress. Here, we show that the Arabidopsis (Arabidopsis thaliana) ortholog, AtRGGA, is a functional RNA-binding protein required for a proper response to osmotic stress. AtRGGA gene expression was up-regulated in seedlings after long-term exposure to abscisic acid (ABA) and polyethylene glycol, while treatments with NaCl resulted in AtRGGA down-regulation. AtRGGA promoter analysis showed activity in several tissues, including stomata, the organs controlling transpiration. Fusion of AtRGGA with yellow fluorescent protein indicated that AtRGGA is localized in the cytoplasm and the cytoplasmic perinuclear region. In addition, the rgga knockout mutant was hypersensitive to ABA in root growth and survival tests and to salt stress during germination and at the vegetative stage. AtRGGA-overexpressing plants showed higher tolerance to ABA and salt stress on plates and in soil, accumulating lower levels of proline when exposed to drought stress. Finally, a global analysis of gene expression revealed extensive alterations in the transcriptome under salt stress, including several genes such as ASCORBATE PEROXIDASE2, GLUTATHIONE S-TRANSFERASE TAU9, and several SMALL AUXIN UPREGULATED RNA-like genes showing opposite expression behavior in transgenic and knockout plants. Taken together, our results reveal an important role of AtRGGA in the mechanisms of plant response and adaptation to stress.
Abiotic stresses such as salinity and drought account for extensive reductions in yields of agricultural crops. While salt stress has an ionic component specifically brought about by Na+ toxicity, both drought and salinity challenge plants by imposing osmotic stress, caused by a reduction in soil water potential (Maggio et al., 2006). As a result of osmotic stress, a complex response aimed at limiting cellular damages and rescuing a new homeostasis is elicited in plants, which includes a coordination of biochemical and physiological changes, including stomata closure, cell growth alterations, photosynthesis inhibition, flowering time and root architecture modification, and inhibition of seed germination (Zhu, 2002). A key role in the regulation of these processes is played by the hormone abscisic acid (ABA). A major breakthrough in our understanding of the osmotic stress responses has come with the recent identification of the ABA PYRABACTIN RESISTANCE (PYR)/PYRABACTIN RESISTANCE-LIKE1 (PYR1)/REGULATORY COMPONENT OF ABA RECEPTOR (RCAR) receptors and the elucidation of their mechanism of action in ABA-mediated signaling cascades (Fujii et al., 2009; Ma et al., 2009; Park et al., 2009).
Osmotic stress induces an increase in ABA concentration, perceived by the PYR/PYL/RCAR receptors. When ABA is ligated, a conformational change is induced in members of the PYR/PYL/RCAR family of receptors, which become able to bind and inhibit type 2C protein phosphatases, thus releasing SUCROSE NON-FERMENTING1-RELATED PROTEIN KINASE2.2 (SnRK2.2), SnRK2.3, and SnRK2.6 kinases from inhibition. In turn, ABA-activated SnRK2s phosphorylate ABA-responsive element-binding transcription factors such as ABF2 to induce the up-regulation of ABA-responsive genes and, in guard cells, plasma membrane-located ion channels such as SLOW ANION CHANNEL1 and POTASSIUM CHANNEL IN ARABIDOPSIS THALIANA1 potassium channel to promote and maintain stomata closure (Geiger et al., 2009, 2010; Sato et al., 2009; Hubbard et al., 2010; Klingler et al., 2010). ABA-independent pathways, which may involve ABA-unresponsive members of the SnRK2 family (Fujii and Zhu, 2012), also participate in osmotic stress responses and seem to interact and converge with ABA-mediated pathways (Ishitani et al., 1997).
While outstanding progress has been made in the elucidation of perception and signaling cascades resulting in stress-induced modifications of gene expression and channel activation, RNA regulatory mechanisms such as synthesis, processing, transport, translation, storage, stability, and degradation of RNA molecules are emerging as key processes participating in the modulation of cellular responses to stress (Ambrosone et al., 2012; Nakaminami et al., 2012). The importance of mRNA stability mechanisms is known in the case of the Na+/H+ antiporter SALT OVERLY SENSITIVE1 (SOS1) required for tolerance to salt stress. SOS1 mRNA is highly unstable in control conditions, but within 10 min after the imposition of salt stress, SOS1 transcript is stabilized in a process mediated by reactive oxygen species (Shi et al., 2003; Chung et al., 2008). A strong impact of stress on translation efficiency has been shown in the case of hypoxia, where Branco-Price et al. (2008) demonstrated that a mechanism of selective mRNA translation without reduction of transcription coordinated metabolic adjustments to oxygen deprivation. Recently, it was shown that the RNA-binding protein (RBP) OLIGOURIDYLATE BINDING PROTEIN1 participates in the selective mRNA translation mechanism during hypoxia by sequestrating mRNAs in stress granules. Upon reoxygenation, stress granules dissolve and mRNAs return to actively translating polysomes (Sorenson and Bailey-Serres, 2014).
The regulation of RNA metabolism directly or indirectly involves RBPs, which are distinguished based on the presence and organization of several different functional motifs and domains, with the RNA recognition motif (RRM) and K homology domain being the most common in plants (Lorković, 2009). Other domains and motifs include the Tudor SN domain, Arg repeats, glycine-rich domain (GR), zinc finger domain (Burd and Dreyfuss, 1994; Albà and Pagès, 1998; Lorković and Barta, 2002), Arg/Gly motif, and cold shock domain (Nakaminami et al., 2012; Ambrosone et al., 2013). Several RBPs have recently been shown to be involved in plant development and stress responses. Tudor SN (TSN) proteins are RBPs involved in RNA stability control upon salt stress. Double mutants tsn1/tsn2 showed a drastic reduction in germination, growth, survival, and fitness under high-salinity stress (dit Frey et al., 2010). RBPs were also shown to be involved in response to heat and cold stress. REGULATOR OF C-REPEAT/DEHYDRATION-RESPONSIVE ELEMENT BINDING FACTOR GENE EXPRESSION3 is a K homology domain-containing RBP that was shown to be a negative regulator of heat stress response by repressing the expression of several heat stress factors, such as HSFA1a, HSFA1b, and HSFA1d (Guan et al., 2013). The zinc finger-containing glycine-rich RBP AtRZ-1A is induced by cold, and, when overexpressed, increases freezing tolerance in Arabidopsis (Arabidopsis thaliana; Kim et al., 2005). Manipulation of the expression of a GR- and RRM domain-containing protein, AtGRP7, impacts stress tolerance under high salinity, drought, or cold stress. Overexpression of AtGRP7 increased freezing tolerance but also caused delayed germination and seedling growth under salt or dehydration stress (Kim et al., 2008).
In an effort to identify genes important for adaptation to osmotic stress, we isolated StRGGA, a gene encoding a putative RBP, whose expression was induced in potato (Solanum tuberosum) cell cultures gradually adapted to high concentrations of polyethylene glycol (PEG), while no change in StRGGA expression was observed when cells were shocked with PEG (Ambrosone et al., 2011). Here, we present the characterization of the putative RGGA ortholog in Arabidopsis. AtRGGA is expressed in several tissues, including stomata, and transcript abundance is increased in cells and plants exposed to PEG and ABA. AtRGGA encodes a cytosolic protein capable of binding RNA in vitro. Transgenic plants overexpressing AtRGGA are more tolerant to ABA, drought, and salt stress, whereas rgga mutant plants are more sensitive to ABA and osmotic stresses. Manipulation of AtRGGA expression has a severe impact on whole-gene expression, indicating that AtRGGA has an important functional role in planta.
RESULTS
AtRGGA Gene Expression in Response to Stress and ABA Treatments
In a previous study, we isolated StRGGA (GenBank accession no. FM209282), whose gene expression is specifically induced in culture cells of potato gradually adapted to high concentrations of PEG. Exposure of potato cells to abrupt osmotic stress did not elicit a change in StRGGA transcript abundance (Ambrosone et al., 2011; Fig. 1A; Supplemental Table S1). The deduced protein sequence of StRGGA shares 63% sequence homology with the protein encoded by the locus At4g16830 of Arabidopsis (Supplemental Fig. S1), which, therefore, is hypothesized to be the Arabidopsis ortholog (AtRGGA). To investigate whether AtRGGA was also induced by stress treatments in Arabidopsis, we analyzed gene expression in cells and seedlings exposed to NaCl and osmotic (PEG) stress. ABA treatments were also included to assess a possible involvement of the hormone in the regulation of AtRGGA transcript abundance. In MM2D cells (Menges and Murray, 2002), NaCl, ABA, and PEG treatments induced a significant up-regulation of AtRGGA compared with control untreated cells (Fig. 1B). Induction seemed to be highest after NaCl treatment, which, however, also caused a reduction in cell viability (Supplemental Fig. S2). In seedlings, 24-h treatments with different concentrations of NaCl caused a slight down-regulation of AtRGGA expression (Fig. 1C), while there was an up-regulation in seedlings exposed for 2 d to ABA and PEG (Fig. 1D), indicating that AtRGGA transcript abundance is reduced by salt stress in the short term but increased over longer periods of exposure to ABA and osmotic stress.
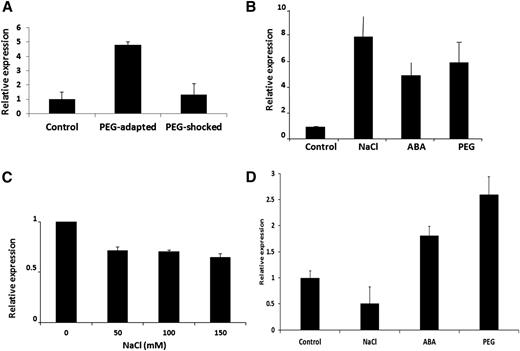
Expression analysis of RGGA in potato and Arabidopsis. A, Expression of RGGA in cells of potato in control conditions and after gradual (PEG-adapted) or abrupt (PEG-shocked) exposure to PEG. B, Gene expression of RGGA in Arabidopsis MM2D cells exposed for 24 h to NaCl (150 mm), ABA (50 μm), or 10% (w/v) PEG. C, AtRGGA expression in 14-d-old seedlings of Arabidopsis treated for 24 h with different concentrations of NaCl as indicated. D, AtRGGA expression in Arabidopsis seedlings after 48 h of exposure to 35% (w/v) PEG, NaCl (120 mm), or ABA (10 μm). Gene expression analyses were conducted by quantitative reverse transcription (qRT)-PCR.
AtRGGA Binds RNA in Vitro
Protein sequence analysis of AtRGGA showed the presence of a Suppressor of Tom1 (Stm1) domain, found at the N-terminal region of the yeast (Saccharomyces cerevisiae) Stm1 nucleic acid-binding protein, and a Hyaluronan-Binding Protein4_Plasminogen Activator Inhibitor-1 mRNA-Binding Protein1 (HABP4_PAI-RBP1) domain, found in RBPs, suggesting that AtRGGA could be an RBP (Fig. 2A). To verify this hypothesis, an RNA electromobility shift assay (EMSA) was performed using recombinant, His-tagged AtRGGA (His-RGGA) and total RNA extracted from control as well as salt-stressed whole seedlings. RNA was labeled with biotin and incubated with or without His-RGGA prior to electrophoresis in native conditions. A recombinant version of the PYR1 ABA receptor, His-PYR1, was used as a negative control. As shown in Figure 2B, an RNA mobility shift was specifically observed when RNA was incubated with AtRGGA, indicating that AtRGGA was capable of binding RNA, and the binding was competed by adding an excess of unlabeled RNA, thus showing that AtRGGA is a bona fide RBP. To assess the specificity of AtRGGA binding to RNA, poly(A+) and poly(A−) RNA fractions were used for RNA EMSA. A band shift after incubation with His-RGGA was observed when poly(A−) RNA was used, indicating that RGGA binds to one or more RNAs contained in the poly(A−) RNA fraction.

A, Schematic representation of Arabidopsis AtRGGA protein domain organization. Gray boxes indicate the locations of the Stm1 N-terminal domain (Stm1; InterPro no. IPR019084) and the hyaluronan/mRNA-binding domain (HABP4_PAI1_RBP1; InterPro no. IPR006861). B, EMSA of Arabidopsis RNA incubated with recombinant AtRGGA (His-RGGA). RNA was extracted from NaCl-treated (Salt Stress RNA) or untreated (Control RNA) plants and labeled with biotin. Unlabeled RNA (160-fold) was used as a competitor. Recombinant PYR1 (His-PYR1) served as a negative control. C, EMSA of Arabidopsis total, poly(A+), and poly(A−) RNA incubated without or with recombinant AtRGGA (His-RGGA). The brackets indicate labeled RNA, and the arrows indicate RGGA-bound RNA.
AtRGGA Promoter Activity in Tissues and Protein Subcellular Localization
To gain insights into the function of AtRGGA in plants, we proceeded to analyze its expression pattern and subcellular localization. Transgenic plants expressing the GUS reporter gene driven by the putative promoter (defined as 2 kb upstream of the protein-coding sequence) of AtRGGA were stained using 5-bromo-4-chloro-3-indolyl glucuronide to visualize the spatial and temporal patterns of activity of the AtRGGA promoter. As shown in Figure 3, GUS activity was visualized both in seedlings and in adult plants in several organs, including leaves, roots, inflorescences, and siliques. Interestingly, within leaves, a strong staining of stomata was observed (Fig. 3D), indicating the expression of AtRGGA in guard cells. In reproductive organs, GUS activity was visualized in pollen grains (Fig. 3, G and H) and tubes of germinating pollen (Fig. 3H) as well as in funiculi attaching seeds to siliques (Fig. 3, M and N). Results gathered by GUS staining assays were generally consistent with publicly available expression data showing the presence of AtRGGA transcript in all analyzed tissues (Supplemental Fig. S3).
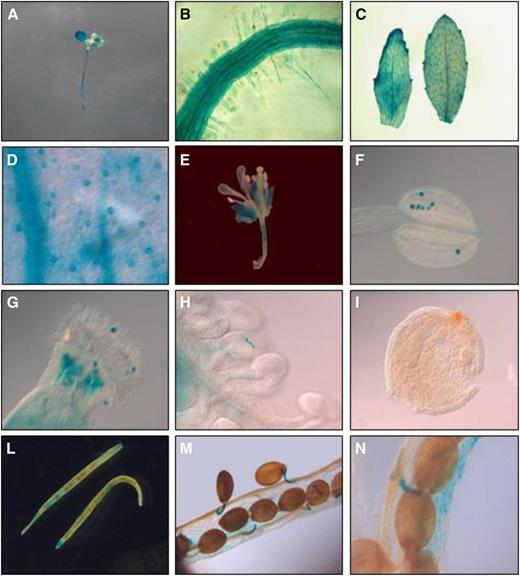
AtRGGA promoter activity in tissues of Arabidopsis. GUS staining was performed in vegetative and reproductive tissues of transgenic Arabidopsis plants expressing the GUS reporter gene under the control of the AtRGGA promoter. Five-day-old seedling (A), root (B), leaves (C), inflorescences (E), and siliques (L–N) were stained. Closeup views of stomata (D), anther (F), stigma (G), ovary (H), and ovule (I) are also shown.
To analyze AtRGGA protein subcellular localization, we generated transgenic plants overexpressing a yellow fluorescent protein (YFP)-RGGA fusion protein. Young seedlings of YFP-RGGA were observed by confocal laser scanning microscopy following a short incubation in propidium iodide to counterstain cell walls. As shown in Figure 4A, in cells of the root apex, where vacuoles are less developed, a clear YFP signal was observed in the cytoplasm. Upon prolonged incubation in propidium iodide solution, minor staining of nuclear DNA could be achieved. In this case, we observed exclusion of YFP-RGGA from the nuclei in cells of the root elongation zone, while strong YFP fluorescence was visualized in the perinuclear region of the cytoplasm (Fig. 4B). In leaf tissues, YFP signal was especially strong in stomata (Fig. 4C). Similar results were obtained using transgenic plants expressing a C-terminal fusion of AtRGGA with YFP (Fig. 4D). Together, the protein subcellular localization studies indicate that AtRGGA localizes in the cytoplasm and the perinuclear region.
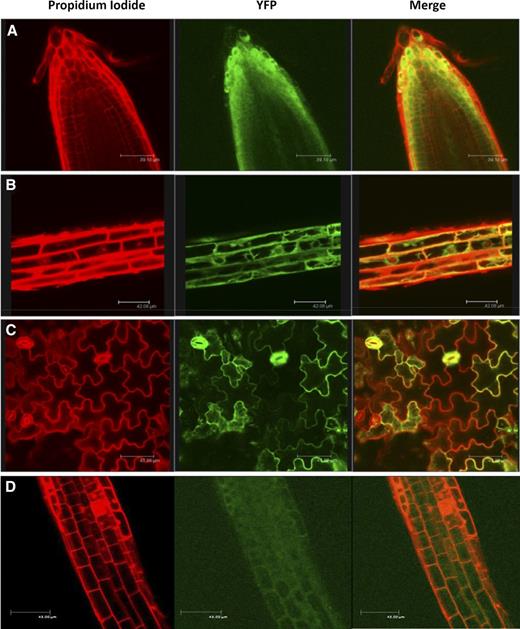
AtRGGA protein localization in Arabidopsis. Confocal microscopy visualization was performed for transgenic Arabidopsis plants expressing a YFP-RGGA or RGGA-YFP (D) fusion protein. Propidium iodide staining, YFP fluorescence, and merged images of root apex (A), root elongation zone (B and D), and leaf epidermal cells (C) are shown.
Functional Analysis of AtRGGA
To characterize the role of AtRGGA in plant responses to salt and drought stress, a transfer DNA (T-DNA) insertion mutant in which AtRGGA gene expression is abolished (SALK_143514; rgga; Fig. 5A; Supplemental Fig. S4) was obtained from the Arabidopsis Biological Resource Center, and transgenic plants overexpressing a FLAG-RGGA fusion protein (Fig. 5B; Supplemental Fig. S4) were generated. In control conditions, rgga plants appeared to have larger rosettes compared with wild-type Columbia-0 (Col-0) plants and showed delayed flowering in a long-day (16 h of light/8 h of darkness) regime (Supplemental Fig. S4).
![Characterization of plants with modified expression of AtRGGA. A, Identification of an RGGA knockout mutant. The top shows a representative model of the At4g16830 locus encoding RGGA in Arabidopsis, showing the location of the T-DNA insertion in SALK_143514 (rgga). The bottom shows semiquantitative reverse transcription-PCR analysis to confirm that the expression of At4g16830 is abolished in the rgga mutant. β-Actin was amplified as an internal standard. B, Immunoblot using α-FLAG antibody of total proteins extracted from Arabidopsis plants transformed to overexpress the fusion protein FLAG-RGGA (35S::FLAG-RGGA). Different transgenic lines (#10, #15, #18, and #20), along with the wild type (Col-0) and controls transformed with the empty binary plasmid (empty vector), are shown. Ponceau staining of Rubisco small subunit (RbcS) served as a loading control. C, Germination analysis of AtRGGA knockout and transgenic plants in the presence of NaCl (120 mm). Germination was scored in terms of fully expanded cotyledons 7 d after stratification. Data reported are means ± sd from three independent experiments. The asterisk denotes a significant difference between Col-0 and rgga (P < 0.05) according to Student’s t test. D, Survival test of 18-d-old seedlings germinated on germination medium (GM; 4.3 g L−1 MS salts, 30% [w/v] Suc, pH 5.7) and transferred to NaCl (180 mm) medium. Survival was scored daily in terms of absence of necrotic or bleached leaves. Data are means ± sd of three independent experiments (n = 30). Asterisks denote statistically significant differences versus Col-0 assessed by χ2 test (*P < 0.05, **P < 0.01).](https://oup.silverchair-cdn.com/oup/backfile/Content_public/Journal/plphys/168/1/10.1104_pp.114.255802/2/m_plphys_v168_1_292_f5.jpeg?Expires=1750333356&Signature=SB23Q4rySVRimgp1YAlB8G~enQTPn2xHy~xy~fAI0PrfDOmtTfjM0rmWd79vUwj43Nz7AeyaEfUCuHB1UCuvqKgn9tCCnQy7SSQUkwpqOAle3HWShb0hYDtuE4B~JTlcKBgIC6vpbB5yEm~uLY4xSBT8wRPzbtu2PIzhgtiKpxkW-4i85urUdx2MyLNN68jyFrikCZ1pRlruojHFcjdj4las9BTzkVkAN2yQ9tEBQ~LC5xOdBfrf2i5mxfy0ZuSc9XTNTOKqS4O4sRK9te3jyz0nGRUEj7I-o6An9tJz9eLAgViRZ1IwuAjgf5SlKMxKzvpdcGGzLVnumn9zNDTuVQ__&Key-Pair-Id=APKAIE5G5CRDK6RD3PGA)
Characterization of plants with modified expression of AtRGGA. A, Identification of an RGGA knockout mutant. The top shows a representative model of the At4g16830 locus encoding RGGA in Arabidopsis, showing the location of the T-DNA insertion in SALK_143514 (rgga). The bottom shows semiquantitative reverse transcription-PCR analysis to confirm that the expression of At4g16830 is abolished in the rgga mutant. β-Actin was amplified as an internal standard. B, Immunoblot using α-FLAG antibody of total proteins extracted from Arabidopsis plants transformed to overexpress the fusion protein FLAG-RGGA (35S::FLAG-RGGA). Different transgenic lines (#10, #15, #18, and #20), along with the wild type (Col-0) and controls transformed with the empty binary plasmid (empty vector), are shown. Ponceau staining of Rubisco small subunit (RbcS) served as a loading control. C, Germination analysis of AtRGGA knockout and transgenic plants in the presence of NaCl (120 mm). Germination was scored in terms of fully expanded cotyledons 7 d after stratification. Data reported are means ± sd from three independent experiments. The asterisk denotes a significant difference between Col-0 and rgga (P < 0.05) according to Student’s t test. D, Survival test of 18-d-old seedlings germinated on germination medium (GM; 4.3 g L−1 MS salts, 30% [w/v] Suc, pH 5.7) and transferred to NaCl (180 mm) medium. Survival was scored daily in terms of absence of necrotic or bleached leaves. Data are means ± sd of three independent experiments (n = 30). Asterisks denote statistically significant differences versus Col-0 assessed by χ2 test (*P < 0.05, **P < 0.01).
Phenotype analyses in the presence of stress were conducted at different developmental stages. At the germination stage, rgga displayed a higher sensitivity to NaCl compared with wild-type Col-0. In particular, while an average of 83% of Col-0 seeds presented fully expanded cotyledons after 7 d of exposure to NaCl (120 mm), only 64% of rgga seeds were germinated (Fig. 5C). In contrast, seeds of overexpressing plants (35S::FLAG-RGGA) did not show any significant differences in their ability to germinate in salt stress medium compared with the wild type or controls transformed with the empty vector (Fig. 5C).
Survival tests instead showed differences in the ability to withstand salt stress conditions of both mutant and overexpressing plants compared with controls. After 7 d of exposure to high-salt conditions, both the knockout mutant and overexpressing plants showed significantly different survival percentages from the wild type. More than 60% of seedlings of three different overexpressing lines did not display any signs of necrosis or bleaching, compared with 36% of Col-0 seedlings, while rgga seedlings were more sensitive to salt stress, with only 7% of plants surviving long-term exposure to NaCl (180 mm; Fig. 5D). Differences in sensitivity to salt stress also could be observed visually (Fig. 6A).
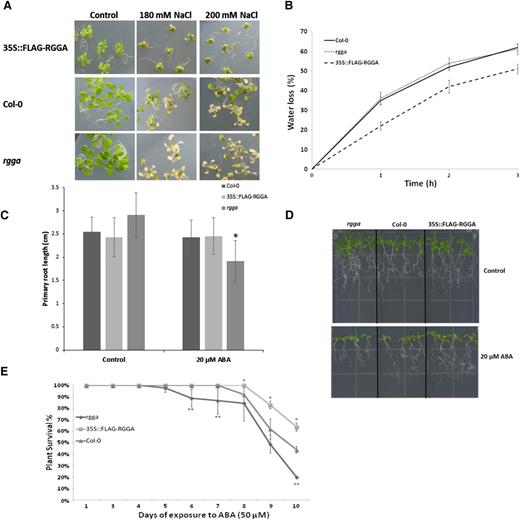
Phenotypes of RGGA knockout and overexpressing plants. A, Phenotypes of Col-0, rgga, and 35S::FLAG-RGGA plants grown on GM for 14 d and exposed for 7 d to NaCl (180 or 200 mm). B, Water loss of leaves detached from Col-0, rgga, and 35S::FLAG-RGGA plants. Data are presented as percentages of initial weight lost at different time points (1, 2, and 3 h). Each point consists of average values ± sd (n = 5 for each line). Data relative to 35S::FLAG-RGGA represent means of three independent transgenic lines. C, Quantification of primary root length of 14-d-old seedlings germinated for 4 d on GM and transferred to control GM medium or medium containing 20 µm ABA. Values are means ± sd (n = 25). The asterisk indicates a statistically significant difference assessed by Student’s t test (P < 0.001). D, Photograph of seedlings grown as described in C. E, Survival test of 18-d-old seedlings germinated on GM and transferred to ABA (50 µm) medium. Survival was scored daily in terms of absence of necrotic or bleached leaves. Data are means ± sd of three independent experiments (n = 30). Asterisks denote statistically significant differences versus Col-0 assessed by χ2 test (*P < 0.05, **P < 0.01).
Closure of stomata when exposed to a dry environment is an important ABA-dependent mechanism contributing to plant stress tolerance, whose efficiency can be inferred by measuring the decline in fresh weight of detached leaves in a time course (Raschke, 1970; Verslues et al., 2006). Therefore, water-loss measurements during the course of 3 h were performed on both rgga mutant and 35S::FLAG-RGGA plants. As shown in Figure 6B, wild-type Col-0 and rgga had lost a similar amount of water (62% and 61% of fresh weight, respectively), while leaves detached from overexpressing plants retained a higher amount of water, having lost 51% of their initial fresh weight, thus suggesting that an increased expression of AtRGGA results in a more efficient closure of stomata in drought stress conditions.
To further assess the role of RGGA in ABA-dependent mechanisms of response to environmental stresses, root growth and survival tests in the presence of ABA were performed. Root growth experiments on plates showed a hypersensitivity of rgga to the presence of ABA in the medium, while overexpressing plants did not display significant differences as compared with Col-0 (Fig. 6, C and D). In terms of survival of ABA exposure, 10 d of treatment could highlight significant differences between genotypes. 35S::FLAG-RGGA plants showed a higher ability to tolerate the presence of 50 µm ABA in the medium, with 60% of plants still surviving after 10 d of exposure to the hormone, while the mutant only had about 20% of individuals still surviving and the wild type had about 47%.
Opposite phenotypes of mutant and transgenic plants in terms of survival of stress conditions were also observed in soil-grown plants. When drought or salt stress was imposed, the rgga mutant appeared more sensitive than the wild type, with a more obvious bleaching of leaves. In contrast, overexpressing plants showed less wilting and bleaching symptoms in the presence of salt and drought stress compared with Col-0 (Fig. 7A). Pro content after prolonged exposure to salt and drought stress was measured in the wild type, rgga, and 35S::FLAG-RGGA. As shown in Figure 7B, levels of Pro in control conditions were similar, with less than 0.2 mmol g−1 fresh weight for all the tested genotypes. After 7 d of exposure to drought or salt stress, there was a dramatic increase in Pro content, with levels quintupling in Col-0 and rgga. In AtRGGA-overexpressing plants, the increase in Pro was less sharp, particularly after drought stress, where plants from three independent transgenic lines accumulated significantly less Pro (Fig. 7B). In salt stress, however, only one of the three tested lines was significantly different from wild-type or rgga levels (Fig. 7B).
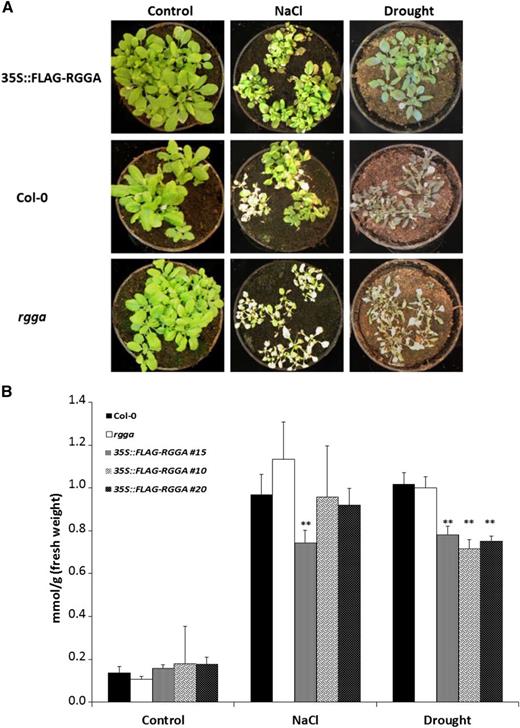
Phenotypes of AtRGGA knockout and overexpressing plants. A, Representative 4-week-old plants of Col-0, rgga, and 35S::FLAG-RGGA genotypes grown in control conditions or after 7-d NaCl (300 mm) or drought treatment. B, Pro concentrations in Col-0, rgga, and 35S::FLAG-RGGA plants treated as described in A. Asterisks indicate statistically significant differences assessed by Student’s t test (P < 0.01).
Gene Expression Analyses in rgga and 35S::FLAG-RGGA Plants
To analyze the impact of a modified expression of AtRGGA on the Arabidopsis transcriptome, microarray analyses were performed on RNA extracted from AtRGGA knockout or overexpressing seedlings grown in control conditions or after exposure to salt stress. The results of microarray analysis were validated using quantitative PCR (qPCR; Supplemental Table S2). In general, an overall perturbation of the transcriptome was observed both in control and stress treatments, with large numbers of genes showing a variation in gene expression in rgga and 35S::FLAG-RGGA (Supplemental Tables S3–S10). An analysis using the Singular Enrichment Analysis tool of agriGO (Du et al., 2010) was performed to identify significantly enriched Gene Ontology (GO) terms in each treatment/genotype. In both mutant and overexpressing plants in control and stress conditions, several GO terms were significantly enriched either in down- and up-regulated genes, with the terms response to stimulus, regulation of cellular process, and biological regulation having among the lowest false discovery rate values in most of the conditions considered (Supplemental Figs. S5 and S6). Genes showing opposite behaviors in the mutant and overexpressing lines in terms of up- or down-regulation as compared with Col-0 are summarized for controls (Table I) and salt stress conditions (Table II). As expected, AtRGGA (At4g16830) was present in both conditions as down-regulated in the knockout plants and up-regulated in overexpressing plants. In control conditions, DEHYDRATION-RESPONSIVE ELEMENT-BINDING PROTEIN2A (DREB2A) and DREB19, key transcription factors involved in drought stress responses, and several heat shock proteins, including HSP21 and HSP22, were down-regulated in rgga and had an opposite behavior in 35S::FLAG-RGGA (Table I). After stress treatment, several genes coding for SAUR-like auxin-responsive proteins were up-regulated in rgga and down-regulated in 35S::FLAG-RGGA (Table II). Among the genes down-regulated in rgga and up-regulated in 35S::FLAG-RGGA, we identified the reactive oxygen species scavengers APX2, a cytosolic member of the l-ascorbate peroxidase gene family, and GSTU9, together with transcription factors WRKY41 and WRKY50, the latter previously shown to be involved in defense responses and the former recently shown to be involved in the modulation of ABSCISIC ACID INSENSITIVE3 transcript abundance and ABA sensitivity at the seed and early seedling stages (Gao et al., 2011; Ding et al., 2014).
Fold change values obtained by microarray analysis compared with wild-type Col-0 of genes showing opposite behavior in rgga and 35S::FLAG-RGGA in control conditions
The locus corresponding to AtRGGA is highlighted in boldface.
Probe . | Locus . | Gene Name . | Genotype . | |
---|---|---|---|---|
rgga . | 35S::FLAG-RGGA . | |||
A_84_P20528 | BARS1 | Baruol synthase | −4.10 | 2.53 |
A_84_P12286 | AT1G19060 | Hypothetical protein | −2.56 | 3.47 |
A_84_P835922 | UGT88A1 | UDP-glucosyltransferase88A1 | −2.32 | 2.20 |
A_84_P225559 | WRKY51 | Putative WRKY transcription factor51 | −2.09 | 3.41 |
A_84_P18337 | AOX1B | Alternative oxidase1B | −10.38 | 2.95 |
A_84_P18276 | AT2G39590 | 40S ribosomal protein S15a-3 | −3.17 | 7.54 |
A_84_P806445 | AT4G16830 | Hyaluronan/mRNA-binding domain-containing protein | −19.86 | 24.09 |
A_84_P15686 | ATHSP22.0 | Heat shock protein22 | −5.20 | 4.74 |
A_84_P13852 | HSP21 | Heat shock protein21 | −5.66 | 3.41 |
A_84_P17108 | AT1G71000 | Chaperone DnaJ domain-containing protein | −2.71 | 8.07 |
A_84_P21479 | AT4G16830 | Hyaluronan/mRNA binding domain-containing protein | −13.40 | 13.50 |
A_84_P822865 | DREB2A | Dehydration-responsive element-binding protein2A | −3.60 | 2.12 |
A_84_P22181 | PMZ | Zinc finger AN1 domain-containing stress-associated protein12 | −2.48 | 2.12 |
A_84_P10708 | AT2G38340 | Dehydration-responsive element-binding protein19 | −3.23 | 2.08 |
A_84_P11439 | AT1G52560 | HSP20-like chaperone | −2.86 | 3.09 |
A_84_P11143 | WRKY38 | Putative WRKY transcription factor38 | −2.50 | 2.60 |
A_84_P807598 | AT5G22430 | Pollen Ole e1 allergen and extensin family protein | 2.82 | −3.57 |
A_84_P799181 | AT1G05660 | Pectin lyase-like protein | 2.32 | −2.25 |
A_84_P22316 | CYP706A7 | Cytochrome P450, family 706, subfamily A, polypeptide 7 | 2.20 | −3.48 |
A_84_P99106 | CLE4 | Protein CLAVATA3/Embryo Surrounding Region-related4 | 4.01 | −3.14 |
A_84_P15932 | TIP2;3 | Aquaporin TIP2-3 | 2.33 | −9.10 |
A_84_P20461 | AT4G25250 | Plant invertase/pectin methylesterase inhibitor domain-containing protein | 4.16 | −3.20 |
A_84_P10835 | AT3G32030 | Terpene cyclase C1 domain-containing protein | 3.59 | −2.69 |
A_84_P15498 | AT3G02620 | Acyl-[acyl-carrier-protein] desaturase | 2.69 | −3.10 |
A_84_P11650 | AT1G07550 | Putative Leu-rich repeat receptor-like Ser/Thr-protein kinase | 2.77 | −2.11 |
A_84_P16139 | CYP702A1 | Cytochrome P450, family 702, subfamily A, polypeptide 1 | 3.25 | −2.79 |
A_84_P15513 | AT3G14540 | Terpene cyclase C1 domain-containing protein | 3.03 | −3.87 |
A_84_P869688 | AGP30 | Arabinogalactan protein30 | 2.69 | −4.71 |
A_84_P14717 | EXPA17 | Putative expansin A17 | 3.58 | −2.63 |
A_84_P12815 | ATGSTF13 | Glutathione S-transferase-like protein | 3.47 | −2.23 |
A_84_P13690 | AT3G46370 | Leu-rich repeat protein kinase-like protein | 2.58 | −2.12 |
A_84_P11899 | SULTR1;1 | Sulfate transporter1.1 | 3.21 | −2.47 |
A_84_P513890 | AT4G11780 | Hypothetical protein | 2.62 | −2.14 |
A_84_P19188 | CYP718 | Cytochrome P450, family 718 | 2.20 | −2.38 |
A_84_P18031 | AT1G05660 | Pectin lyase-like protein | 2.53 | −2.74 |
A_84_P95306 | FUT5 | Xyloglucan fucosyltransferase | 4.46 | −2.10 |
A_84_P759704 | AT3G32030 | Terpene cyclase C1 domain-containing protein | 3.67 | −2.07 |
A_84_P17611 | AT4G22460 | Bifunctional inhibitor/lipid-transfer protein/seed storage 2S albumin-like protein | 2.83 | −5.30 |
A_84_P17872 | AT5G60520 | Late embryogenesis abundant protein-like protein | 2.20 | −2.57 |
A_84_P11794 | AT3G45080 | Sulfotransferase family protein | 2.44 | −2.19 |
A_84_P597193 | AT2G48080 | Oxidoreductase, 2-Oxoglutarate-Fe(II) oxygenase-like protein | 3.83 | −2.61 |
A_84_P13496 | AT2G25150 | HXXXD-type acyltransferase-like protein | 2.14 | −4.90 |
A_84_P18124 | AT1G06330 | Heavy metal transport/detoxification-like protein | 2.99 | −2.53 |
A_84_P22080 | AGP30 | Arabinogalactan protein30 | 2.90 | −4.48 |
A_84_P13966 | AT5G14650 | Polygalacturonase | 4.57 | −3.00 |
A_84_P599045 | AT3G19320 | Leu-rich repeat-containing protein | 4.47 | −4.51 |
A_84_P513281 | AT5G62330 | Hypothetical protein | 4.43 | −5.10 |
A_84_P262640 | AT5G22430 | Pollen Ole e1 allergen and extensin family protein | 2.80 | −4.44 |
A_84_P759732 | AT3G32030 | Terpene cyclase C1 domain-containing protein | 3.86 | −3.09 |
Probe . | Locus . | Gene Name . | Genotype . | |
---|---|---|---|---|
rgga . | 35S::FLAG-RGGA . | |||
A_84_P20528 | BARS1 | Baruol synthase | −4.10 | 2.53 |
A_84_P12286 | AT1G19060 | Hypothetical protein | −2.56 | 3.47 |
A_84_P835922 | UGT88A1 | UDP-glucosyltransferase88A1 | −2.32 | 2.20 |
A_84_P225559 | WRKY51 | Putative WRKY transcription factor51 | −2.09 | 3.41 |
A_84_P18337 | AOX1B | Alternative oxidase1B | −10.38 | 2.95 |
A_84_P18276 | AT2G39590 | 40S ribosomal protein S15a-3 | −3.17 | 7.54 |
A_84_P806445 | AT4G16830 | Hyaluronan/mRNA-binding domain-containing protein | −19.86 | 24.09 |
A_84_P15686 | ATHSP22.0 | Heat shock protein22 | −5.20 | 4.74 |
A_84_P13852 | HSP21 | Heat shock protein21 | −5.66 | 3.41 |
A_84_P17108 | AT1G71000 | Chaperone DnaJ domain-containing protein | −2.71 | 8.07 |
A_84_P21479 | AT4G16830 | Hyaluronan/mRNA binding domain-containing protein | −13.40 | 13.50 |
A_84_P822865 | DREB2A | Dehydration-responsive element-binding protein2A | −3.60 | 2.12 |
A_84_P22181 | PMZ | Zinc finger AN1 domain-containing stress-associated protein12 | −2.48 | 2.12 |
A_84_P10708 | AT2G38340 | Dehydration-responsive element-binding protein19 | −3.23 | 2.08 |
A_84_P11439 | AT1G52560 | HSP20-like chaperone | −2.86 | 3.09 |
A_84_P11143 | WRKY38 | Putative WRKY transcription factor38 | −2.50 | 2.60 |
A_84_P807598 | AT5G22430 | Pollen Ole e1 allergen and extensin family protein | 2.82 | −3.57 |
A_84_P799181 | AT1G05660 | Pectin lyase-like protein | 2.32 | −2.25 |
A_84_P22316 | CYP706A7 | Cytochrome P450, family 706, subfamily A, polypeptide 7 | 2.20 | −3.48 |
A_84_P99106 | CLE4 | Protein CLAVATA3/Embryo Surrounding Region-related4 | 4.01 | −3.14 |
A_84_P15932 | TIP2;3 | Aquaporin TIP2-3 | 2.33 | −9.10 |
A_84_P20461 | AT4G25250 | Plant invertase/pectin methylesterase inhibitor domain-containing protein | 4.16 | −3.20 |
A_84_P10835 | AT3G32030 | Terpene cyclase C1 domain-containing protein | 3.59 | −2.69 |
A_84_P15498 | AT3G02620 | Acyl-[acyl-carrier-protein] desaturase | 2.69 | −3.10 |
A_84_P11650 | AT1G07550 | Putative Leu-rich repeat receptor-like Ser/Thr-protein kinase | 2.77 | −2.11 |
A_84_P16139 | CYP702A1 | Cytochrome P450, family 702, subfamily A, polypeptide 1 | 3.25 | −2.79 |
A_84_P15513 | AT3G14540 | Terpene cyclase C1 domain-containing protein | 3.03 | −3.87 |
A_84_P869688 | AGP30 | Arabinogalactan protein30 | 2.69 | −4.71 |
A_84_P14717 | EXPA17 | Putative expansin A17 | 3.58 | −2.63 |
A_84_P12815 | ATGSTF13 | Glutathione S-transferase-like protein | 3.47 | −2.23 |
A_84_P13690 | AT3G46370 | Leu-rich repeat protein kinase-like protein | 2.58 | −2.12 |
A_84_P11899 | SULTR1;1 | Sulfate transporter1.1 | 3.21 | −2.47 |
A_84_P513890 | AT4G11780 | Hypothetical protein | 2.62 | −2.14 |
A_84_P19188 | CYP718 | Cytochrome P450, family 718 | 2.20 | −2.38 |
A_84_P18031 | AT1G05660 | Pectin lyase-like protein | 2.53 | −2.74 |
A_84_P95306 | FUT5 | Xyloglucan fucosyltransferase | 4.46 | −2.10 |
A_84_P759704 | AT3G32030 | Terpene cyclase C1 domain-containing protein | 3.67 | −2.07 |
A_84_P17611 | AT4G22460 | Bifunctional inhibitor/lipid-transfer protein/seed storage 2S albumin-like protein | 2.83 | −5.30 |
A_84_P17872 | AT5G60520 | Late embryogenesis abundant protein-like protein | 2.20 | −2.57 |
A_84_P11794 | AT3G45080 | Sulfotransferase family protein | 2.44 | −2.19 |
A_84_P597193 | AT2G48080 | Oxidoreductase, 2-Oxoglutarate-Fe(II) oxygenase-like protein | 3.83 | −2.61 |
A_84_P13496 | AT2G25150 | HXXXD-type acyltransferase-like protein | 2.14 | −4.90 |
A_84_P18124 | AT1G06330 | Heavy metal transport/detoxification-like protein | 2.99 | −2.53 |
A_84_P22080 | AGP30 | Arabinogalactan protein30 | 2.90 | −4.48 |
A_84_P13966 | AT5G14650 | Polygalacturonase | 4.57 | −3.00 |
A_84_P599045 | AT3G19320 | Leu-rich repeat-containing protein | 4.47 | −4.51 |
A_84_P513281 | AT5G62330 | Hypothetical protein | 4.43 | −5.10 |
A_84_P262640 | AT5G22430 | Pollen Ole e1 allergen and extensin family protein | 2.80 | −4.44 |
A_84_P759732 | AT3G32030 | Terpene cyclase C1 domain-containing protein | 3.86 | −3.09 |
The locus corresponding to AtRGGA is highlighted in boldface.
Probe . | Locus . | Gene Name . | Genotype . | |
---|---|---|---|---|
rgga . | 35S::FLAG-RGGA . | |||
A_84_P20528 | BARS1 | Baruol synthase | −4.10 | 2.53 |
A_84_P12286 | AT1G19060 | Hypothetical protein | −2.56 | 3.47 |
A_84_P835922 | UGT88A1 | UDP-glucosyltransferase88A1 | −2.32 | 2.20 |
A_84_P225559 | WRKY51 | Putative WRKY transcription factor51 | −2.09 | 3.41 |
A_84_P18337 | AOX1B | Alternative oxidase1B | −10.38 | 2.95 |
A_84_P18276 | AT2G39590 | 40S ribosomal protein S15a-3 | −3.17 | 7.54 |
A_84_P806445 | AT4G16830 | Hyaluronan/mRNA-binding domain-containing protein | −19.86 | 24.09 |
A_84_P15686 | ATHSP22.0 | Heat shock protein22 | −5.20 | 4.74 |
A_84_P13852 | HSP21 | Heat shock protein21 | −5.66 | 3.41 |
A_84_P17108 | AT1G71000 | Chaperone DnaJ domain-containing protein | −2.71 | 8.07 |
A_84_P21479 | AT4G16830 | Hyaluronan/mRNA binding domain-containing protein | −13.40 | 13.50 |
A_84_P822865 | DREB2A | Dehydration-responsive element-binding protein2A | −3.60 | 2.12 |
A_84_P22181 | PMZ | Zinc finger AN1 domain-containing stress-associated protein12 | −2.48 | 2.12 |
A_84_P10708 | AT2G38340 | Dehydration-responsive element-binding protein19 | −3.23 | 2.08 |
A_84_P11439 | AT1G52560 | HSP20-like chaperone | −2.86 | 3.09 |
A_84_P11143 | WRKY38 | Putative WRKY transcription factor38 | −2.50 | 2.60 |
A_84_P807598 | AT5G22430 | Pollen Ole e1 allergen and extensin family protein | 2.82 | −3.57 |
A_84_P799181 | AT1G05660 | Pectin lyase-like protein | 2.32 | −2.25 |
A_84_P22316 | CYP706A7 | Cytochrome P450, family 706, subfamily A, polypeptide 7 | 2.20 | −3.48 |
A_84_P99106 | CLE4 | Protein CLAVATA3/Embryo Surrounding Region-related4 | 4.01 | −3.14 |
A_84_P15932 | TIP2;3 | Aquaporin TIP2-3 | 2.33 | −9.10 |
A_84_P20461 | AT4G25250 | Plant invertase/pectin methylesterase inhibitor domain-containing protein | 4.16 | −3.20 |
A_84_P10835 | AT3G32030 | Terpene cyclase C1 domain-containing protein | 3.59 | −2.69 |
A_84_P15498 | AT3G02620 | Acyl-[acyl-carrier-protein] desaturase | 2.69 | −3.10 |
A_84_P11650 | AT1G07550 | Putative Leu-rich repeat receptor-like Ser/Thr-protein kinase | 2.77 | −2.11 |
A_84_P16139 | CYP702A1 | Cytochrome P450, family 702, subfamily A, polypeptide 1 | 3.25 | −2.79 |
A_84_P15513 | AT3G14540 | Terpene cyclase C1 domain-containing protein | 3.03 | −3.87 |
A_84_P869688 | AGP30 | Arabinogalactan protein30 | 2.69 | −4.71 |
A_84_P14717 | EXPA17 | Putative expansin A17 | 3.58 | −2.63 |
A_84_P12815 | ATGSTF13 | Glutathione S-transferase-like protein | 3.47 | −2.23 |
A_84_P13690 | AT3G46370 | Leu-rich repeat protein kinase-like protein | 2.58 | −2.12 |
A_84_P11899 | SULTR1;1 | Sulfate transporter1.1 | 3.21 | −2.47 |
A_84_P513890 | AT4G11780 | Hypothetical protein | 2.62 | −2.14 |
A_84_P19188 | CYP718 | Cytochrome P450, family 718 | 2.20 | −2.38 |
A_84_P18031 | AT1G05660 | Pectin lyase-like protein | 2.53 | −2.74 |
A_84_P95306 | FUT5 | Xyloglucan fucosyltransferase | 4.46 | −2.10 |
A_84_P759704 | AT3G32030 | Terpene cyclase C1 domain-containing protein | 3.67 | −2.07 |
A_84_P17611 | AT4G22460 | Bifunctional inhibitor/lipid-transfer protein/seed storage 2S albumin-like protein | 2.83 | −5.30 |
A_84_P17872 | AT5G60520 | Late embryogenesis abundant protein-like protein | 2.20 | −2.57 |
A_84_P11794 | AT3G45080 | Sulfotransferase family protein | 2.44 | −2.19 |
A_84_P597193 | AT2G48080 | Oxidoreductase, 2-Oxoglutarate-Fe(II) oxygenase-like protein | 3.83 | −2.61 |
A_84_P13496 | AT2G25150 | HXXXD-type acyltransferase-like protein | 2.14 | −4.90 |
A_84_P18124 | AT1G06330 | Heavy metal transport/detoxification-like protein | 2.99 | −2.53 |
A_84_P22080 | AGP30 | Arabinogalactan protein30 | 2.90 | −4.48 |
A_84_P13966 | AT5G14650 | Polygalacturonase | 4.57 | −3.00 |
A_84_P599045 | AT3G19320 | Leu-rich repeat-containing protein | 4.47 | −4.51 |
A_84_P513281 | AT5G62330 | Hypothetical protein | 4.43 | −5.10 |
A_84_P262640 | AT5G22430 | Pollen Ole e1 allergen and extensin family protein | 2.80 | −4.44 |
A_84_P759732 | AT3G32030 | Terpene cyclase C1 domain-containing protein | 3.86 | −3.09 |
Probe . | Locus . | Gene Name . | Genotype . | |
---|---|---|---|---|
rgga . | 35S::FLAG-RGGA . | |||
A_84_P20528 | BARS1 | Baruol synthase | −4.10 | 2.53 |
A_84_P12286 | AT1G19060 | Hypothetical protein | −2.56 | 3.47 |
A_84_P835922 | UGT88A1 | UDP-glucosyltransferase88A1 | −2.32 | 2.20 |
A_84_P225559 | WRKY51 | Putative WRKY transcription factor51 | −2.09 | 3.41 |
A_84_P18337 | AOX1B | Alternative oxidase1B | −10.38 | 2.95 |
A_84_P18276 | AT2G39590 | 40S ribosomal protein S15a-3 | −3.17 | 7.54 |
A_84_P806445 | AT4G16830 | Hyaluronan/mRNA-binding domain-containing protein | −19.86 | 24.09 |
A_84_P15686 | ATHSP22.0 | Heat shock protein22 | −5.20 | 4.74 |
A_84_P13852 | HSP21 | Heat shock protein21 | −5.66 | 3.41 |
A_84_P17108 | AT1G71000 | Chaperone DnaJ domain-containing protein | −2.71 | 8.07 |
A_84_P21479 | AT4G16830 | Hyaluronan/mRNA binding domain-containing protein | −13.40 | 13.50 |
A_84_P822865 | DREB2A | Dehydration-responsive element-binding protein2A | −3.60 | 2.12 |
A_84_P22181 | PMZ | Zinc finger AN1 domain-containing stress-associated protein12 | −2.48 | 2.12 |
A_84_P10708 | AT2G38340 | Dehydration-responsive element-binding protein19 | −3.23 | 2.08 |
A_84_P11439 | AT1G52560 | HSP20-like chaperone | −2.86 | 3.09 |
A_84_P11143 | WRKY38 | Putative WRKY transcription factor38 | −2.50 | 2.60 |
A_84_P807598 | AT5G22430 | Pollen Ole e1 allergen and extensin family protein | 2.82 | −3.57 |
A_84_P799181 | AT1G05660 | Pectin lyase-like protein | 2.32 | −2.25 |
A_84_P22316 | CYP706A7 | Cytochrome P450, family 706, subfamily A, polypeptide 7 | 2.20 | −3.48 |
A_84_P99106 | CLE4 | Protein CLAVATA3/Embryo Surrounding Region-related4 | 4.01 | −3.14 |
A_84_P15932 | TIP2;3 | Aquaporin TIP2-3 | 2.33 | −9.10 |
A_84_P20461 | AT4G25250 | Plant invertase/pectin methylesterase inhibitor domain-containing protein | 4.16 | −3.20 |
A_84_P10835 | AT3G32030 | Terpene cyclase C1 domain-containing protein | 3.59 | −2.69 |
A_84_P15498 | AT3G02620 | Acyl-[acyl-carrier-protein] desaturase | 2.69 | −3.10 |
A_84_P11650 | AT1G07550 | Putative Leu-rich repeat receptor-like Ser/Thr-protein kinase | 2.77 | −2.11 |
A_84_P16139 | CYP702A1 | Cytochrome P450, family 702, subfamily A, polypeptide 1 | 3.25 | −2.79 |
A_84_P15513 | AT3G14540 | Terpene cyclase C1 domain-containing protein | 3.03 | −3.87 |
A_84_P869688 | AGP30 | Arabinogalactan protein30 | 2.69 | −4.71 |
A_84_P14717 | EXPA17 | Putative expansin A17 | 3.58 | −2.63 |
A_84_P12815 | ATGSTF13 | Glutathione S-transferase-like protein | 3.47 | −2.23 |
A_84_P13690 | AT3G46370 | Leu-rich repeat protein kinase-like protein | 2.58 | −2.12 |
A_84_P11899 | SULTR1;1 | Sulfate transporter1.1 | 3.21 | −2.47 |
A_84_P513890 | AT4G11780 | Hypothetical protein | 2.62 | −2.14 |
A_84_P19188 | CYP718 | Cytochrome P450, family 718 | 2.20 | −2.38 |
A_84_P18031 | AT1G05660 | Pectin lyase-like protein | 2.53 | −2.74 |
A_84_P95306 | FUT5 | Xyloglucan fucosyltransferase | 4.46 | −2.10 |
A_84_P759704 | AT3G32030 | Terpene cyclase C1 domain-containing protein | 3.67 | −2.07 |
A_84_P17611 | AT4G22460 | Bifunctional inhibitor/lipid-transfer protein/seed storage 2S albumin-like protein | 2.83 | −5.30 |
A_84_P17872 | AT5G60520 | Late embryogenesis abundant protein-like protein | 2.20 | −2.57 |
A_84_P11794 | AT3G45080 | Sulfotransferase family protein | 2.44 | −2.19 |
A_84_P597193 | AT2G48080 | Oxidoreductase, 2-Oxoglutarate-Fe(II) oxygenase-like protein | 3.83 | −2.61 |
A_84_P13496 | AT2G25150 | HXXXD-type acyltransferase-like protein | 2.14 | −4.90 |
A_84_P18124 | AT1G06330 | Heavy metal transport/detoxification-like protein | 2.99 | −2.53 |
A_84_P22080 | AGP30 | Arabinogalactan protein30 | 2.90 | −4.48 |
A_84_P13966 | AT5G14650 | Polygalacturonase | 4.57 | −3.00 |
A_84_P599045 | AT3G19320 | Leu-rich repeat-containing protein | 4.47 | −4.51 |
A_84_P513281 | AT5G62330 | Hypothetical protein | 4.43 | −5.10 |
A_84_P262640 | AT5G22430 | Pollen Ole e1 allergen and extensin family protein | 2.80 | −4.44 |
A_84_P759732 | AT3G32030 | Terpene cyclase C1 domain-containing protein | 3.86 | −3.09 |
Fold change values obtained by microarray analysis compared with wild-type Col-0 of genes showing opposite behavior in rgga and 35S::FLAG-RGGA after salt stress treatment (48 h, 180 mm NaCl)
The locus corresponding to AtRGGA is highlighted in boldface.
Probe . | Locus . | Gene Name . | Genotype . | |
---|---|---|---|---|
rgga . | 35S::FLAG-RGGA . | |||
A_84_P756195 | AT2G04070 | Multi Antimicrobial Extrusion Protein efflux family protein | −3.59 | 3.60 |
A_84_P768949 | AT5G35688 | Hypothetical protein | −2.62 | 2.21 |
A_84_P11264 | GSTU9 | GST τ9 | −2.03 | 2.11 |
A_84_P762759 | AT3G55672 | Self-incompatibility S1 family protein | −2.01 | 4.19 |
A_84_P89069 | AT3G63360 | Defensin-like protein11 | −2.32 | 2.74 |
A_84_P84999 | AT3G28580 | AAA-type ATPase family protein | −2.42 | 2.50 |
A_84_P593216 | AT5G60250 | C3H4-type zinc finger protein | −2.61 | 2.17 |
A_84_P75404 | AT5G55150 | Hypothetical protein | −3.91 | 2.19 |
A_84_P55910 | WRKY41 | Putative WRKY transcription factor41 | −2.07 | 5.03 |
A_84_P11156 | WRKY50 | Putative WRKY transcription factor50 | −2.22 | 3.45 |
A_84_P580762 | AT3G43710 | Putative F-box/Kelch-repeat protein | −5.29 | 4.42 |
A_84_P806445 | AT4G16830 | Hyaluronan/mRNA-binding domain-containing protein | −5.34 | 21.32 |
A_84_P271800 | EP1 | Putative Cys-rich receptor-like protein kinase9 | −2.16 | 2.17 |
A_84_P23566 | AT5G64790 | O-Glycosylhydrolase family17 protein | −2.36 | 2.28 |
A_84_P606546 | AT5G55270 | Hypothetical protein | −6.56 | 3.20 |
A_84_P833327 | AT1G69550 | Toll/Interleukin1 Receptor-Nucleotide Binding Site-Leu-rich repeat class disease resistance protein | −2.21 | 2.24 |
A_84_P21479 | AT4G16830 | Hyaluronan/mRNA-binding domain-containing protein | −3.45 | 10.14 |
A_84_P23310 | AT4G27580 | Hypothetical protein | −3.14 | 3.11 |
A_84_P20019 | AT1G17960 | Threonyl-tRNA synthetase | −2.54 | 2.75 |
A_84_P11712 | AT3G02810 | Protein kinase domain-containing protein | −3.58 | 3.48 |
A_84_P539380 | AT2G35075 | Hypothetical protein | −3.75 | 2.24 |
A_84_P17261 | UGT84B1 | UDP-Glc:(indol-3-yl)acetate β-d-glucosyltransferase | −2.19 | 3.44 |
A_84_P525660 | AT5G52940 | Hypothetical protein | −3.96 | 2.31 |
A_84_P17379 | APX2 | l-Ascorbate peroxidase | −2.51 | 2.55 |
A_84_P145639 | AT5G07610 | F-box protein | −7.36 | 4.13 |
A_84_P20216 | AT3G05950 | Germin-like protein subfamily 1, member 7 | −2.09 | 8.36 |
A_84_P787266 | WRKY50 | Putative WRKY transcription factor50 | −2.45 | 3.68 |
A_84_P590126 | AT3G22540 | Hypothetical protein | 3.10 | −2.22 |
A_84_P16241 | AT1G52190 | Putative peptide transporter | 2.87 | −2.43 |
A_84_P22477 | MYB40 | Myb domain protein40 | 3.73 | −2.85 |
A_84_P275660 | AGP14 | Arabinogalactan protein14 | 2.81 | −2.93 |
A_84_P812392 | AT4G33720 | Putative pathogenesis-related protein | 64.60 | −2.05 |
A_84_P94979 | AT5G18060 | SAUR-like auxin-responsive protein | 2.37 | −8.25 |
A_84_P137439 | PAR2 | Phytochrome rapidly regulated2 protein | 2.09 | −6.16 |
A_84_P20461 | AT4G25250 | Plant invertase/pectin methylesterase inhibitor domain-containing protein | 2.22 | −3.57 |
A_84_P751997 | AT1G15630 | Hypothetical protein | 2.21 | −2.88 |
A_84_P544465 | KDR | Basic helix-loop-helix domain-containing protein | 2.36 | −3.64 |
A_84_P79415 | AT2G18300 | Transcription factor basic helix-loop-helix64 | 2.47 | −2.92 |
A_84_P175621 | AGL14 | Agamous-like MADS box protein AGL14 | 2.42 | −2.46 |
A_84_P11420 | AT1G64920 | UDP-glycosyltransferase-like protein | 2.41 | −2.28 |
A_84_P310653 | PDF2.5 | Defensin-like protein6 | 2.54 | −2.42 |
A_84_P12525 | AT2G47880 | Glutaredoxin C13 | 3.54 | −3.34 |
A_84_P20410 | ADS1 | Ɗ-9 acyl-lipid desaturase1 | 2.46 | −2.25 |
A_84_P732491 | AT5G03995 | Hypothetical protein | 4.90 | −2.38 |
A_84_P14346 | SULTR1;3 | Sulfate transporter1.3 | 2.32 | −2.49 |
A_84_P19794 | MYB66 | Transcription factor WEREWOLF | 2.45 | −2.86 |
A_84_P186864 | IQD12 | IQ-domain12 protein | 2.49 | −2.85 |
A_84_P20189 | AT3G03830 | SAUR-like auxin-responsive protein | 2.03 | −6.53 |
A_84_P121222 | AT3G21330 | Transcription factor basic helix-loop-helix87 | 3.68 | −3.68 |
A_84_P500468 | RALFL27 | Protein ralf-like27 | 3.61 | −2.67 |
A_84_P24090 | AT3G45710 | Major facilitator protein | 2.29 | −2.67 |
A_84_P759704 | AT3G32030 | Terpene cyclase C1 domain-containing protein | 2.17 | −3.34 |
A_84_P16734 | SAUR15 | SAUR-like auxin-responsive protein | 3.08 | −2.10 |
A_84_P166743 | AT5G14890 | NHL domain-containing protein | 2.02 | −2.15 |
A_84_P21135 | AT3G03820 | SAUR-like auxin-responsive protein | 3.45 | −2.31 |
A_84_P17378 | GRI | Stigma-specific Stig1 family protein | 2.75 | −2.07 |
A_84_P759796 | AT3G46270 | Receptor protein kinase-like protein | 2.81 | −2.07 |
A_84_P64634 | AT4G10910 | Hypothetical protein | 2.45 | −4.97 |
A_84_P18924 | AT1G52130 | Man-binding lectin-like protein | 2.38 | −6.23 |
A_84_P12394 | AT1G14960 | Major latex-related protein | 2.34 | −4.72 |
Probe . | Locus . | Gene Name . | Genotype . | |
---|---|---|---|---|
rgga . | 35S::FLAG-RGGA . | |||
A_84_P756195 | AT2G04070 | Multi Antimicrobial Extrusion Protein efflux family protein | −3.59 | 3.60 |
A_84_P768949 | AT5G35688 | Hypothetical protein | −2.62 | 2.21 |
A_84_P11264 | GSTU9 | GST τ9 | −2.03 | 2.11 |
A_84_P762759 | AT3G55672 | Self-incompatibility S1 family protein | −2.01 | 4.19 |
A_84_P89069 | AT3G63360 | Defensin-like protein11 | −2.32 | 2.74 |
A_84_P84999 | AT3G28580 | AAA-type ATPase family protein | −2.42 | 2.50 |
A_84_P593216 | AT5G60250 | C3H4-type zinc finger protein | −2.61 | 2.17 |
A_84_P75404 | AT5G55150 | Hypothetical protein | −3.91 | 2.19 |
A_84_P55910 | WRKY41 | Putative WRKY transcription factor41 | −2.07 | 5.03 |
A_84_P11156 | WRKY50 | Putative WRKY transcription factor50 | −2.22 | 3.45 |
A_84_P580762 | AT3G43710 | Putative F-box/Kelch-repeat protein | −5.29 | 4.42 |
A_84_P806445 | AT4G16830 | Hyaluronan/mRNA-binding domain-containing protein | −5.34 | 21.32 |
A_84_P271800 | EP1 | Putative Cys-rich receptor-like protein kinase9 | −2.16 | 2.17 |
A_84_P23566 | AT5G64790 | O-Glycosylhydrolase family17 protein | −2.36 | 2.28 |
A_84_P606546 | AT5G55270 | Hypothetical protein | −6.56 | 3.20 |
A_84_P833327 | AT1G69550 | Toll/Interleukin1 Receptor-Nucleotide Binding Site-Leu-rich repeat class disease resistance protein | −2.21 | 2.24 |
A_84_P21479 | AT4G16830 | Hyaluronan/mRNA-binding domain-containing protein | −3.45 | 10.14 |
A_84_P23310 | AT4G27580 | Hypothetical protein | −3.14 | 3.11 |
A_84_P20019 | AT1G17960 | Threonyl-tRNA synthetase | −2.54 | 2.75 |
A_84_P11712 | AT3G02810 | Protein kinase domain-containing protein | −3.58 | 3.48 |
A_84_P539380 | AT2G35075 | Hypothetical protein | −3.75 | 2.24 |
A_84_P17261 | UGT84B1 | UDP-Glc:(indol-3-yl)acetate β-d-glucosyltransferase | −2.19 | 3.44 |
A_84_P525660 | AT5G52940 | Hypothetical protein | −3.96 | 2.31 |
A_84_P17379 | APX2 | l-Ascorbate peroxidase | −2.51 | 2.55 |
A_84_P145639 | AT5G07610 | F-box protein | −7.36 | 4.13 |
A_84_P20216 | AT3G05950 | Germin-like protein subfamily 1, member 7 | −2.09 | 8.36 |
A_84_P787266 | WRKY50 | Putative WRKY transcription factor50 | −2.45 | 3.68 |
A_84_P590126 | AT3G22540 | Hypothetical protein | 3.10 | −2.22 |
A_84_P16241 | AT1G52190 | Putative peptide transporter | 2.87 | −2.43 |
A_84_P22477 | MYB40 | Myb domain protein40 | 3.73 | −2.85 |
A_84_P275660 | AGP14 | Arabinogalactan protein14 | 2.81 | −2.93 |
A_84_P812392 | AT4G33720 | Putative pathogenesis-related protein | 64.60 | −2.05 |
A_84_P94979 | AT5G18060 | SAUR-like auxin-responsive protein | 2.37 | −8.25 |
A_84_P137439 | PAR2 | Phytochrome rapidly regulated2 protein | 2.09 | −6.16 |
A_84_P20461 | AT4G25250 | Plant invertase/pectin methylesterase inhibitor domain-containing protein | 2.22 | −3.57 |
A_84_P751997 | AT1G15630 | Hypothetical protein | 2.21 | −2.88 |
A_84_P544465 | KDR | Basic helix-loop-helix domain-containing protein | 2.36 | −3.64 |
A_84_P79415 | AT2G18300 | Transcription factor basic helix-loop-helix64 | 2.47 | −2.92 |
A_84_P175621 | AGL14 | Agamous-like MADS box protein AGL14 | 2.42 | −2.46 |
A_84_P11420 | AT1G64920 | UDP-glycosyltransferase-like protein | 2.41 | −2.28 |
A_84_P310653 | PDF2.5 | Defensin-like protein6 | 2.54 | −2.42 |
A_84_P12525 | AT2G47880 | Glutaredoxin C13 | 3.54 | −3.34 |
A_84_P20410 | ADS1 | Ɗ-9 acyl-lipid desaturase1 | 2.46 | −2.25 |
A_84_P732491 | AT5G03995 | Hypothetical protein | 4.90 | −2.38 |
A_84_P14346 | SULTR1;3 | Sulfate transporter1.3 | 2.32 | −2.49 |
A_84_P19794 | MYB66 | Transcription factor WEREWOLF | 2.45 | −2.86 |
A_84_P186864 | IQD12 | IQ-domain12 protein | 2.49 | −2.85 |
A_84_P20189 | AT3G03830 | SAUR-like auxin-responsive protein | 2.03 | −6.53 |
A_84_P121222 | AT3G21330 | Transcription factor basic helix-loop-helix87 | 3.68 | −3.68 |
A_84_P500468 | RALFL27 | Protein ralf-like27 | 3.61 | −2.67 |
A_84_P24090 | AT3G45710 | Major facilitator protein | 2.29 | −2.67 |
A_84_P759704 | AT3G32030 | Terpene cyclase C1 domain-containing protein | 2.17 | −3.34 |
A_84_P16734 | SAUR15 | SAUR-like auxin-responsive protein | 3.08 | −2.10 |
A_84_P166743 | AT5G14890 | NHL domain-containing protein | 2.02 | −2.15 |
A_84_P21135 | AT3G03820 | SAUR-like auxin-responsive protein | 3.45 | −2.31 |
A_84_P17378 | GRI | Stigma-specific Stig1 family protein | 2.75 | −2.07 |
A_84_P759796 | AT3G46270 | Receptor protein kinase-like protein | 2.81 | −2.07 |
A_84_P64634 | AT4G10910 | Hypothetical protein | 2.45 | −4.97 |
A_84_P18924 | AT1G52130 | Man-binding lectin-like protein | 2.38 | −6.23 |
A_84_P12394 | AT1G14960 | Major latex-related protein | 2.34 | −4.72 |
The locus corresponding to AtRGGA is highlighted in boldface.
Probe . | Locus . | Gene Name . | Genotype . | |
---|---|---|---|---|
rgga . | 35S::FLAG-RGGA . | |||
A_84_P756195 | AT2G04070 | Multi Antimicrobial Extrusion Protein efflux family protein | −3.59 | 3.60 |
A_84_P768949 | AT5G35688 | Hypothetical protein | −2.62 | 2.21 |
A_84_P11264 | GSTU9 | GST τ9 | −2.03 | 2.11 |
A_84_P762759 | AT3G55672 | Self-incompatibility S1 family protein | −2.01 | 4.19 |
A_84_P89069 | AT3G63360 | Defensin-like protein11 | −2.32 | 2.74 |
A_84_P84999 | AT3G28580 | AAA-type ATPase family protein | −2.42 | 2.50 |
A_84_P593216 | AT5G60250 | C3H4-type zinc finger protein | −2.61 | 2.17 |
A_84_P75404 | AT5G55150 | Hypothetical protein | −3.91 | 2.19 |
A_84_P55910 | WRKY41 | Putative WRKY transcription factor41 | −2.07 | 5.03 |
A_84_P11156 | WRKY50 | Putative WRKY transcription factor50 | −2.22 | 3.45 |
A_84_P580762 | AT3G43710 | Putative F-box/Kelch-repeat protein | −5.29 | 4.42 |
A_84_P806445 | AT4G16830 | Hyaluronan/mRNA-binding domain-containing protein | −5.34 | 21.32 |
A_84_P271800 | EP1 | Putative Cys-rich receptor-like protein kinase9 | −2.16 | 2.17 |
A_84_P23566 | AT5G64790 | O-Glycosylhydrolase family17 protein | −2.36 | 2.28 |
A_84_P606546 | AT5G55270 | Hypothetical protein | −6.56 | 3.20 |
A_84_P833327 | AT1G69550 | Toll/Interleukin1 Receptor-Nucleotide Binding Site-Leu-rich repeat class disease resistance protein | −2.21 | 2.24 |
A_84_P21479 | AT4G16830 | Hyaluronan/mRNA-binding domain-containing protein | −3.45 | 10.14 |
A_84_P23310 | AT4G27580 | Hypothetical protein | −3.14 | 3.11 |
A_84_P20019 | AT1G17960 | Threonyl-tRNA synthetase | −2.54 | 2.75 |
A_84_P11712 | AT3G02810 | Protein kinase domain-containing protein | −3.58 | 3.48 |
A_84_P539380 | AT2G35075 | Hypothetical protein | −3.75 | 2.24 |
A_84_P17261 | UGT84B1 | UDP-Glc:(indol-3-yl)acetate β-d-glucosyltransferase | −2.19 | 3.44 |
A_84_P525660 | AT5G52940 | Hypothetical protein | −3.96 | 2.31 |
A_84_P17379 | APX2 | l-Ascorbate peroxidase | −2.51 | 2.55 |
A_84_P145639 | AT5G07610 | F-box protein | −7.36 | 4.13 |
A_84_P20216 | AT3G05950 | Germin-like protein subfamily 1, member 7 | −2.09 | 8.36 |
A_84_P787266 | WRKY50 | Putative WRKY transcription factor50 | −2.45 | 3.68 |
A_84_P590126 | AT3G22540 | Hypothetical protein | 3.10 | −2.22 |
A_84_P16241 | AT1G52190 | Putative peptide transporter | 2.87 | −2.43 |
A_84_P22477 | MYB40 | Myb domain protein40 | 3.73 | −2.85 |
A_84_P275660 | AGP14 | Arabinogalactan protein14 | 2.81 | −2.93 |
A_84_P812392 | AT4G33720 | Putative pathogenesis-related protein | 64.60 | −2.05 |
A_84_P94979 | AT5G18060 | SAUR-like auxin-responsive protein | 2.37 | −8.25 |
A_84_P137439 | PAR2 | Phytochrome rapidly regulated2 protein | 2.09 | −6.16 |
A_84_P20461 | AT4G25250 | Plant invertase/pectin methylesterase inhibitor domain-containing protein | 2.22 | −3.57 |
A_84_P751997 | AT1G15630 | Hypothetical protein | 2.21 | −2.88 |
A_84_P544465 | KDR | Basic helix-loop-helix domain-containing protein | 2.36 | −3.64 |
A_84_P79415 | AT2G18300 | Transcription factor basic helix-loop-helix64 | 2.47 | −2.92 |
A_84_P175621 | AGL14 | Agamous-like MADS box protein AGL14 | 2.42 | −2.46 |
A_84_P11420 | AT1G64920 | UDP-glycosyltransferase-like protein | 2.41 | −2.28 |
A_84_P310653 | PDF2.5 | Defensin-like protein6 | 2.54 | −2.42 |
A_84_P12525 | AT2G47880 | Glutaredoxin C13 | 3.54 | −3.34 |
A_84_P20410 | ADS1 | Ɗ-9 acyl-lipid desaturase1 | 2.46 | −2.25 |
A_84_P732491 | AT5G03995 | Hypothetical protein | 4.90 | −2.38 |
A_84_P14346 | SULTR1;3 | Sulfate transporter1.3 | 2.32 | −2.49 |
A_84_P19794 | MYB66 | Transcription factor WEREWOLF | 2.45 | −2.86 |
A_84_P186864 | IQD12 | IQ-domain12 protein | 2.49 | −2.85 |
A_84_P20189 | AT3G03830 | SAUR-like auxin-responsive protein | 2.03 | −6.53 |
A_84_P121222 | AT3G21330 | Transcription factor basic helix-loop-helix87 | 3.68 | −3.68 |
A_84_P500468 | RALFL27 | Protein ralf-like27 | 3.61 | −2.67 |
A_84_P24090 | AT3G45710 | Major facilitator protein | 2.29 | −2.67 |
A_84_P759704 | AT3G32030 | Terpene cyclase C1 domain-containing protein | 2.17 | −3.34 |
A_84_P16734 | SAUR15 | SAUR-like auxin-responsive protein | 3.08 | −2.10 |
A_84_P166743 | AT5G14890 | NHL domain-containing protein | 2.02 | −2.15 |
A_84_P21135 | AT3G03820 | SAUR-like auxin-responsive protein | 3.45 | −2.31 |
A_84_P17378 | GRI | Stigma-specific Stig1 family protein | 2.75 | −2.07 |
A_84_P759796 | AT3G46270 | Receptor protein kinase-like protein | 2.81 | −2.07 |
A_84_P64634 | AT4G10910 | Hypothetical protein | 2.45 | −4.97 |
A_84_P18924 | AT1G52130 | Man-binding lectin-like protein | 2.38 | −6.23 |
A_84_P12394 | AT1G14960 | Major latex-related protein | 2.34 | −4.72 |
Probe . | Locus . | Gene Name . | Genotype . | |
---|---|---|---|---|
rgga . | 35S::FLAG-RGGA . | |||
A_84_P756195 | AT2G04070 | Multi Antimicrobial Extrusion Protein efflux family protein | −3.59 | 3.60 |
A_84_P768949 | AT5G35688 | Hypothetical protein | −2.62 | 2.21 |
A_84_P11264 | GSTU9 | GST τ9 | −2.03 | 2.11 |
A_84_P762759 | AT3G55672 | Self-incompatibility S1 family protein | −2.01 | 4.19 |
A_84_P89069 | AT3G63360 | Defensin-like protein11 | −2.32 | 2.74 |
A_84_P84999 | AT3G28580 | AAA-type ATPase family protein | −2.42 | 2.50 |
A_84_P593216 | AT5G60250 | C3H4-type zinc finger protein | −2.61 | 2.17 |
A_84_P75404 | AT5G55150 | Hypothetical protein | −3.91 | 2.19 |
A_84_P55910 | WRKY41 | Putative WRKY transcription factor41 | −2.07 | 5.03 |
A_84_P11156 | WRKY50 | Putative WRKY transcription factor50 | −2.22 | 3.45 |
A_84_P580762 | AT3G43710 | Putative F-box/Kelch-repeat protein | −5.29 | 4.42 |
A_84_P806445 | AT4G16830 | Hyaluronan/mRNA-binding domain-containing protein | −5.34 | 21.32 |
A_84_P271800 | EP1 | Putative Cys-rich receptor-like protein kinase9 | −2.16 | 2.17 |
A_84_P23566 | AT5G64790 | O-Glycosylhydrolase family17 protein | −2.36 | 2.28 |
A_84_P606546 | AT5G55270 | Hypothetical protein | −6.56 | 3.20 |
A_84_P833327 | AT1G69550 | Toll/Interleukin1 Receptor-Nucleotide Binding Site-Leu-rich repeat class disease resistance protein | −2.21 | 2.24 |
A_84_P21479 | AT4G16830 | Hyaluronan/mRNA-binding domain-containing protein | −3.45 | 10.14 |
A_84_P23310 | AT4G27580 | Hypothetical protein | −3.14 | 3.11 |
A_84_P20019 | AT1G17960 | Threonyl-tRNA synthetase | −2.54 | 2.75 |
A_84_P11712 | AT3G02810 | Protein kinase domain-containing protein | −3.58 | 3.48 |
A_84_P539380 | AT2G35075 | Hypothetical protein | −3.75 | 2.24 |
A_84_P17261 | UGT84B1 | UDP-Glc:(indol-3-yl)acetate β-d-glucosyltransferase | −2.19 | 3.44 |
A_84_P525660 | AT5G52940 | Hypothetical protein | −3.96 | 2.31 |
A_84_P17379 | APX2 | l-Ascorbate peroxidase | −2.51 | 2.55 |
A_84_P145639 | AT5G07610 | F-box protein | −7.36 | 4.13 |
A_84_P20216 | AT3G05950 | Germin-like protein subfamily 1, member 7 | −2.09 | 8.36 |
A_84_P787266 | WRKY50 | Putative WRKY transcription factor50 | −2.45 | 3.68 |
A_84_P590126 | AT3G22540 | Hypothetical protein | 3.10 | −2.22 |
A_84_P16241 | AT1G52190 | Putative peptide transporter | 2.87 | −2.43 |
A_84_P22477 | MYB40 | Myb domain protein40 | 3.73 | −2.85 |
A_84_P275660 | AGP14 | Arabinogalactan protein14 | 2.81 | −2.93 |
A_84_P812392 | AT4G33720 | Putative pathogenesis-related protein | 64.60 | −2.05 |
A_84_P94979 | AT5G18060 | SAUR-like auxin-responsive protein | 2.37 | −8.25 |
A_84_P137439 | PAR2 | Phytochrome rapidly regulated2 protein | 2.09 | −6.16 |
A_84_P20461 | AT4G25250 | Plant invertase/pectin methylesterase inhibitor domain-containing protein | 2.22 | −3.57 |
A_84_P751997 | AT1G15630 | Hypothetical protein | 2.21 | −2.88 |
A_84_P544465 | KDR | Basic helix-loop-helix domain-containing protein | 2.36 | −3.64 |
A_84_P79415 | AT2G18300 | Transcription factor basic helix-loop-helix64 | 2.47 | −2.92 |
A_84_P175621 | AGL14 | Agamous-like MADS box protein AGL14 | 2.42 | −2.46 |
A_84_P11420 | AT1G64920 | UDP-glycosyltransferase-like protein | 2.41 | −2.28 |
A_84_P310653 | PDF2.5 | Defensin-like protein6 | 2.54 | −2.42 |
A_84_P12525 | AT2G47880 | Glutaredoxin C13 | 3.54 | −3.34 |
A_84_P20410 | ADS1 | Ɗ-9 acyl-lipid desaturase1 | 2.46 | −2.25 |
A_84_P732491 | AT5G03995 | Hypothetical protein | 4.90 | −2.38 |
A_84_P14346 | SULTR1;3 | Sulfate transporter1.3 | 2.32 | −2.49 |
A_84_P19794 | MYB66 | Transcription factor WEREWOLF | 2.45 | −2.86 |
A_84_P186864 | IQD12 | IQ-domain12 protein | 2.49 | −2.85 |
A_84_P20189 | AT3G03830 | SAUR-like auxin-responsive protein | 2.03 | −6.53 |
A_84_P121222 | AT3G21330 | Transcription factor basic helix-loop-helix87 | 3.68 | −3.68 |
A_84_P500468 | RALFL27 | Protein ralf-like27 | 3.61 | −2.67 |
A_84_P24090 | AT3G45710 | Major facilitator protein | 2.29 | −2.67 |
A_84_P759704 | AT3G32030 | Terpene cyclase C1 domain-containing protein | 2.17 | −3.34 |
A_84_P16734 | SAUR15 | SAUR-like auxin-responsive protein | 3.08 | −2.10 |
A_84_P166743 | AT5G14890 | NHL domain-containing protein | 2.02 | −2.15 |
A_84_P21135 | AT3G03820 | SAUR-like auxin-responsive protein | 3.45 | −2.31 |
A_84_P17378 | GRI | Stigma-specific Stig1 family protein | 2.75 | −2.07 |
A_84_P759796 | AT3G46270 | Receptor protein kinase-like protein | 2.81 | −2.07 |
A_84_P64634 | AT4G10910 | Hypothetical protein | 2.45 | −4.97 |
A_84_P18924 | AT1G52130 | Man-binding lectin-like protein | 2.38 | −6.23 |
A_84_P12394 | AT1G14960 | Major latex-related protein | 2.34 | −4.72 |
DISCUSSION
In a previous report, we identified StRGGA, a potato gene encoding a putative RBP whose expression was specifically induced in cultured cells adapted to high concentrations of PEG (Ambrosone et al., 2011; Fig. 1). Here, we have provided evidence that the putative Arabidopsis ortholog, AtRGGA, is involved in tolerance to drought and salt stress. AtRGGA is up-regulated both in cells and plants upon long-term exposure to PEG and ABA (Fig. 1). AtRGGA is expressed in several Arabidopsis tissues, including tissues that perceive or respond to osmotic stress conditions, such as roots and stomata, and tissues that undergo extensive dehydration processes, such as pollen (Fig. 3). Finally, the rgga knockout mutant displays a seed germination hypersensitive to NaCl and is less tolerant compared with the wild type to salt stress both at the seedling stage and as adult plants (Figs. 5–7). Conversely, transgenic plants overexpressing AtRGGA appear to be better able to withstand salt and drought stress and lose water at a slower rate in detached leaf assays as compared with control untransformed plants (Figs. 5 and 6), indicating that a higher expression of AtRGGA promotes stomatal closure, a largely ABA-dependent process (Verslues et al., 2006). The hypersensitivity of rgga and the lower sensitivity of 35S::FLAG-RGGA plants to ABA (Fig. 6) further indicate that AtRGGA participates in ABA-dependent mechanisms of response to salt and drought stress. When exposed to drought stress, AtRGGA-overexpressing plants also accumulate less Pro (Fig. 7), suggesting that the higher tolerance observed is independent of Pro accumulation, a feature shared with several tolerant mutants such as reduced salt sensitivity1, stigma specific protein1 (stig1), and photoautotrophic salt tolerance1 that do not hyperaccumulate this compatible osmolyte under stress conditions (Werner and Finkelstein, 1995; Tsugane et al., 1999; Gao et al., 2006).
Microarray experiments showed that an abolished/increased expression of AtRGGA has a profound impact on global gene expression, with a misregulation of several thousand genes in control and stress conditions (Tables I and II; Supplemental Tables S3–S10; Supplemental Figs. S5 and S6). Analysis of significantly enriched GO terms showed that terms such as response to stimulus, regulation of cellular process, and biological regulation were among the ones with lowest false discovery rates, with the exception of genes up-regulated in salt-stressed AtRGGA-overexpressing plants, where only the terms response to salt stress, response to stimulus, and response to biotic stimulus were significantly enriched, indicating that the tolerance of RGGA-overexpressing plants is attributable to proteins involved in protection from biotic and abiotic stress. In rgga, the expression of FLOWERING LOCUS T, encoding the mobile signal translocated from leaves to the shoot apex to initiate flowering (Abe et al., 2005; Wigge et al., 2005; Jaeger and Wigge, 2007), is severely reduced, possibly accounting for the delayed-flowering phenotype observed (Supplemental Fig. S4). In the mutant, an up-regulation of several members of the PURPLE ACID PHOSPHATASE (PAP) family was observed after salt stress treatment. PAPs are metalloenzymes involved in diverse biological processes, such as inorganic phosphate uptake, peroxidation, defense against pathogens, and salt stress, through the alleviation of oxidative damage (Li et al., 2008; Ravichandran et al., 2013). PAP transcript up-regulation, therefore, could be an indication of an increased salt stress-caused oxidative damage in rgga compared with control plants. Analysis of genes showing an opposite behavior in the mutant versus overexpressing plants as compared with the wild type (Tables I and II) showed that, in control conditions, transcripts of several genes involved in stress responses, such as the heat shock proteins HSP22 and HSP21 and the transcription factors DREB19 and DREB2A, are down-regulated in rgga and up-regulated in transgenic plants, indicating that higher prestress levels of these genes may partially account for the higher stress tolerance shown by transgenic plants. After salt stress treatment, several members of the SAUR gene family were up-regulated in rgga and down-regulated in overexpressing plants, including SAUR15, At5g18060, and At3g03830. SAUR genes encode small-M r auxin-responsive proteins whose function is still largely unknown, even though members of the SAUR19 subfamily, comprising SAUR19 to SAUR24, have been shown recently to have a role in auxin-mediated cell expansion by regulating plasma membrane H+-ATPase activity (Spartz et al., 2012, 2014). Transcripts of SAUR genes are highly unstable due to the presence in their 3′ untranslated region of a conserved downstream element responsible for mRNA instability (Newman et al., 1993; Gil and Green, 1996; Spartz et al., 2012), and, in an early report, SAUR mRNAs were shown to be stabilized by the inhibition of protein synthesis (Franco et al., 1990). An interesting hypothesis, therefore, could be that AtRGGA might affect RNA stability.
AtRGGA encodes a cytoplasm-localized protein (Fig. 4) with several Gly/Arg motifs that possesses the Stm1 N-terminal and the HABP4_PAI-RBP1 domains characteristic of RNA and nucleic acid-binding proteins (Fig. 2). Stm1 is a yeast G4 quadruplex and purine motif triplex nucleic acid-binding protein of Saccharomyces cerevisiae that has been shown to associate with telomeric Y’ DNA and ribosomes (Van Dyke et al., 2004). HABP4 binds hyaluronan as well as RNA, while the human PAI-1 mRNA-binding protein binds the type 1 plasminogen activator inhibitor and has been suggested to be involved in the regulation of mRNA stability (Huang et al., 2000; Heaton et al., 2001). As expected from the gene annotation, we show that recombinant AtRGGA is capable of efficiently binding RNA in vitro (Fig. 2). The similarity with the Stm1, HABP4, and PAI1 proteins, together with the subcellular localization, suggest that AtRGGA might affect posttranscriptional regulation mechanisms such as the control of RNA stability, storage, or translation efficiency rather than the synthesis or nuclear processing of RNAs. In humans and yeast, RGG motif-containing proteins have emerged as key players involved in the posttranscriptional regulation of gene expression, affecting RNA stability as well as RNA translation to protein through interaction with the translation initiation factor eIF4G, which recruits ribosomes to mRNAs (Rajyaguru and Parker, 2012; Walsh and Mohr, 2014). While the possibility that poly(A+) RNAs are also bound by AtRGGA could not be ruled out, the observed binding to poly(A−) (Fig. 2) raises the possibility that AtRGGA might interact with one or more RNA components of the ribosomes to modify translation efficiency and/or the stability of ribosome-bound mRNAs. Little evidence suggests that binding to the ribosomes can affect mRNA stability. In bacteria, ribosomes usually protect mRNAs from degradation or, in some instances, promote mRNA decay (Deana and Belasco, 2005). In Arabidopsis, a mutant lacking ribosomal protein S27 shows defects in the ability to degrade selected mRNAs when exposed to genotoxic treatments (Revenkova et al., 1999). More recently, polysome association was correlated to transcript stability during stress conditions in rice (Oryza sativa; Park et al., 2012). Further experiments to identify RNA and protein interactors will assess the hypothesis that AtRGGA binds to ribosomes and discern whether mRNA stability and/or translation efficiency are affected in stress conditions by the presence of AtRGGA.
MATERIALS AND METHODS
Plant Material and Stress Treatments
Potato (Solanum tuberosum ‘Desirèe’) cell cultures were generated, maintained, and gradually or abruptly exposed to 20% (w/v) PEG 8000 as described previously (Leone et al., 1994; Ambrosone et al., 2011). Arabidopsis (Arabidopsis thaliana) MM2D cells (ecotype Landsberg erecta) were maintained as described (Menges and Murray, 2002) in continuous dark conditions. For gene expression analyses, cells were treated for 24 h with NaCl (150 mm), ABA (50 μm), or 10% (w/v) PEG 8000. Cell viability was assessed by adding fluorescein diacetate (2 µL of a 5 mg mL−1 stock) to 100 µL of cell culture. Viability was expressed as the percentage of viable cells in stress versus control conditions.
Arabidopsis plants of the Col-0 ecotype were used throughout this study. A knockout line for AtRGGA (rgga; SALK_143514) was obtained from the Nottingham Arabidopsis Stock Centre, and homozygous plants were selected by PCR. Primers were selected using the T-DNA Express primer design tool (http://signal.salk.edu/tdnaprimers.2.html; Supplemental Table S1). Transgenic plants overexpressing FLAG-tagged RGGA in the Col-0 background were generated by floral dip transformation (Clough and Bent, 1998).
For gene expression studies, 14-d-old seedlings grown on solid GM medium were transferred to plates containing NaCl (50, 100, or 150 mm) and incubated for 24 h or incubated for 7 d on plates containing 35% (w/v) PEG, NaCl (120 mm), or ABA (10 μm). For germination analyses, seeds were sown in the presence of NaCl (120 mm). Germination was scored in terms of fully expanded cotyledons after 7 d of incubation.
Survival tests were carried out using 18-d-old seedlings germinated on GM plates and transferred to NaCl- or ABA-containing medium (180 mm or 50 μm, respectively). Survival was scored daily in terms of absence of necrotic or bleached leaves.
To score root growth in the presence of ABA, 4-d-old seedlings grown on GM medium and showing equal primary root length were transferred to GM medium or medium with 20 µm ABA. Photographs were taken, and root length was scored 10 d after transfer. Stress treatments in soil were performed on 4-week-old plants by water withdrawal for 7 d or by watering with 300 mm NaCl every other day for 7 d. In each irrigation event, the volume of the salt solution was 5% of the pot volume.
For microarray gene expression analyses, 10-d-old seedlings grown on GM plates were transferred to GM or GM + 180 mm NaCl plates for 48 h prior to RNA extraction.
Plasmid Construction
Gateway technology (Life Technologies) was used to obtain binary vectors for promoter and protein localization studies as well as to produce transgenic overexpressing plants. The putative promoter of AtRGGA (corresponding to 2 kb upstream of ATG) was amplified from genomic DNA extracted from Col-0 plant RGGA coding sequence amplified from clone U22150 provided by The Arabidopsis Information Resource. PCR amplifications were carried out using Pfu DNA polymerase (Stratagene). Primers used are listed in Supplemental Table S1. The promoter and coding sequences were cloned into pDONR207 using BP clonase to obtain AtRGGAPromoter::pDONR207 and AtRGGA::pDONR207 entry vectors. LR clonase was used for recombination with destination vectors, which were pMDC164 (Curtis and Grossniklaus, 2003) for promoter studies, pEG101 and pEG104 (Earley et al., 2006) for protein localization studies, and pEG202 (Earley et al., 2006) to produce FLAG-tagged overexpressing plants.
To produce His-tagged AtRGGA in Escherichia coli, the coding sequence was amplified and cloned between SalI and NotI restriction sites of pET28a vector. All constructs were sequenced to rule out the presence of mutations introduced by PCR. The His-tagged AtRGGA from pET28a constructs was overexpressed following transformation in E. coli BL21 (DE3). Cells were recovered and lysed by 1 mg mL−1 lysozyme after growth at 37°C on Luria-Bertani medium supplemented with 50 μg mL−1 kanamycin. Induction was carried out by adding 0.5 mm isopropyl-β-d-thiogalactopyranoside to a culture at an optical density measured at 600 nm of 1 and then incubating the culture at 37°C for 4 h. Protein constructs were purified by nickel affinity chromatography as described by the manufacturer (Qiagen). The purified protein was analyzed by SDS-PAGE as described by Laemmli (1970).
Gene Expression Analyses
qPCR gene expression analyses used 1 µg of DNaseI-treated RNA converted to complementary DNA utilizing SuperScript II Reverse Transcriptase and oligo(dT20) following the manufacturer’s instructions (Life Technologies).
The obtained complementary DNA was diluted 1:20, and 4.5 µL was used for each qRT-PCR, carried out with Platinum SYBR Green qPCR SuperMix and Elongation Factor EF1α as an endogenous control. Primers used (5 µm) are listed in Supplemental Table S1. qRT-PCR was performed with ABI 7900 HT (Applied Biosystems). At least nine technical replicates per gene per biological replicate were performed. Three biological replicates per genotype were tested, and data were analyzed using RQ Manager followed by analysis with Data Assist (Applied Biosystems) to group together the different replicates and biological replicates and perform statistical analysis.
For microarray gene expression studies, RNA extracted using Trizol (Life Technologies) was quantified and quality checked using the Nanodrop-ND 8000 spectrophotometer (Thermo Fisher Scientific) and the 2100 Bioanalyzer (Agilent Technologies), respectively. Samples with the RNA integrity number of 9 and above were chosen for further analysis. Microarray analysis was performed using the Arabidopsis (V4) Gene Expression Microarray, Design ID: 021169 (Agilent Technologies), containing 43,803 Arabidopsis gene probes and 1,417 Agilent control probes. Total RNA (150 ng) was used to prepare cyanine-3-labeled probe with the help of the low-RNA input linear amplification/labeling kit (Agilent Technologies). The dye incorporation and the copy RNA yield were measured using the Nanodrop-ND 8000 spectrophotometer (Thermo Fisher Scientific). Labeled copy RNA probes (1.65 µg) were fragmented using fragmentation buffer (Agilent Technologies) and hybridized to the Arabidopsis arrays in the presence of the gene expression hybridization buffer HI-RPM and blocking agent (Agilent Technologies) for 17 h at 65°C with a 10-rpm rotation speed in a hybridization oven (Agilent Technologies). After the 17-h incubation, the arrays were washed using low-stringency wash buffer 1 (Agilent Technologies) at room temperature for 1 min followed by a high-stringency wash using wash buffer 2 (Agilent Technologies) at 37°C. The arrays were air dried and scanned using the high-resolution array scanner (Agilent Technologies) with the appropriate settings for the one-color gene expression arrays. The signal intensities were extracted from the scanned images with the aid of Feature Extraction Software 10.7.1.1 (Agilent Technologies) and subjected to background subtraction and spatial detrending. The outliers and the abnormal features were flagged, and the data were normalized using intraarray percentile shift normalization (threshold of 75 and above) and median-based interarray normalization. GeneSpring GX (Agilent Technologies) was used to calculate the intensity ratios and fold changes. All the genes with P values below 0.05 and fold change above 2 were chosen for GO enrichment analysis, which was carried out by Sequentia Italia as described below.
Common sets of differentially expressed genes were identified between the different groups by using Venny (http://bioinfogp.cnb.csic.es/tools/venny/index.html; Oliveros, 2007). The identification of enriched GO functional categories was performed by using the Singular Enrichment Analysis tool of agriGO (http://bioinfo.cau.edu.cn/agriGO/analysis.php). Arabidopsis was selected as a supported species, and the suggested background was used. A hypergeometric test was used as a statistical method to identify enriched categories, while the other parameters were left as default. When the number of enriched categories was too much for easy understanding, the software REVIGO (http://revigo.irb.hr; Supek et al., 2011) was used to obtain reduced lists. The dimension of the output list was set to small, and the database of Arabidopsis GO terms was used.
Immunoblotting
To detect the FLAG-RGGA fusion protein in overexpressing lines, immunoblotting was performed using α-FLAG antibody (Sigma-Aldrich). Total proteins were extracted from 100 mg of tissue using extraction buffer (2 m urea, 0.1 m NaH2PO4, and 0.01 m Tris, pH 8) and protease inhibitor cocktail (Sigma-Aldrich). After clarification, protein concentration was estimated using the Bradford reagent assay (Bio-Rad), and 50 µg of total protein was used for SDS-PAGE followed by transfer on nitrocellulose membranes (Amersham, GE Healthcare). A 1:5,000 dilution of α-FLAG antibody was used for immunoblotting following the manufacturer’s instructions.
RNA EMSA
Total RNA (700 ng) extracted from control and salt stress-treated plants was labeled with biotin using the RNA 3′ End Biotinylation Kit (Pierce Biotechnology) following the manufacturer’s instructions. One microliter of a 1:20 dilution (approximately 30 ng) of the labeled RNA was used for each EMSA reaction. Similar amounts of labeled RNA were also used for poly(A+) and poly(A−) RNA, which were prepared using the mRNA Isolation Kit (Roche Applied Science). RNA was incubated with 7.5 µg of His-tagged AtRGGA or His-tagged PYR1. When present, unlabeled RNA was used as a competitor at 560 ng (approximately 160-fold). The RNA EMSA was carried out with the LightShift Chemiluminescent RNA EMSA Kit (Pierce Biotechnology). The binding reaction was analyzed by gel electrophoresis on a native 6% polyacrylamide gel in 0.5× Tris-borate/EDTA buffer and transferred to a nylon membrane.
Pro Content Determination
Pro extraction from Arabidopsis plants grown in soil and subjected to drought stress was carried out as described by Claussen (2005). Briefly, 500 mg of leaves was ground in liquid nitrogen and resuspended in an aqueous solution containing 3% (w/v) 5-sulfosalicylic acid. Suspension was clarified with Ederol round filters (Schleicher & Schull). One milliliter of filtered suspension was diluted with 1 mL of glacial acetic acid and 1 mL of a 2.5% ninhydrin solution (glacial acetic acid:water:orthophosphoric acid, 6:3:1). After incubation at 100°C for 1 h, samples were read at an optical density measured at 546 nm. Three biological replicates were analyzed for each genotype, and each sample was replicated at least three times.
Confocal Imaging
Confocal imaging was carried out with the Leica TCS SP2 system. Propidium iodide staining was carried out as described previously (Sassi et al., 2012). Staining times were as follows: 1 min to counterstain cell walls only, and at least 1 h to achieve minimal nuclear staining.
Supplemental Data
The following supplemental materials are available.
Supplemental Figure S1 . Alignment of deduced RGGA protein sequences of potato and Arabidopsis.
Supplemental Figure S2 . Cell viability measurements of Arabidopsis MM2D cells.
Supplemental Figure S3 . AtRGGA gene expression in Arabidopsis tissues and developmental stages.
Supplemental Figure S4 . Phenotypes of Arabidopsis plants with modified expression of AtRGGA.
Supplemental Figure S5 . Enriched GO categories of differentially expressed genes in 35S::FLAG-RGGA plants.
Supplemental Figure S6 . Enriched GO categories of differentially expressed genes in rgga plants.
Supplemental Table S1 . Primers used in this study.
Supplemental Table S2 . qPCR validation of microarray results.
Supplemental Table S3 . Genes repressed in the rgga mutant in salt stress conditions.
Supplemental Table S4 . Genes induced in the rgga mutant in salt stress conditions.
Supplemental Table S5 . Genes induced in the rgga mutant in control conditions.
Supplemental Table S6 . Genes repressed in the rgga mutant in control conditions.
Supplemental Table S7 . Genes induced in plants overexpressing RGGA in salt stress conditions.
Supplemental Table S8 . Genes repressed in plants overexpressing RGGA in salt stress conditions.
Supplemental Table S9 . Genes induced in plants overexpressing RGGA in control conditions.
Supplemental Table S10 . Genes repressed in plants overexpressing RGGA in control conditions.
ACKNOWLEDGMENTS
We thank the Nottingham Arabidopsis Stock Centre for providing T-DNA mutant seeds and Gaetano Guarino and Rosario Nocerino for excellent technical assistance.
Glossary
- ABA
abscisic acid
- RBP
RNA-binding protein
- RRM
RNA recognition motif
- GR
glycine-rich domain
- PEG
polyethylene glycol
- EMSA
electromobility shift assay
- T-DNA
transfer DNA
- Col-0
Columbia-0
- qPCR
quantitative PCR
- GO
Gene Ontology
- qRT
quantitative reverse transcription
LITERATURE CITED
Oliveros JC (2007) VENNY: an interactive tool for comparing lists with Venn diagrams. http://bioinfogp.cnb.csic.es/tools/venny/index.html (October 2013)
Author notes
This work was supported by the Italian Ministry of University and Research (Fondo per gli Investimenti della Ricerca di Base Project PlantSTRESS and GenoPOM-PRO project no. PON02_00395_3082360).
These authors contributed equally to the article.
Present address: National Research Council of Italy, Istituto di Cibernetica E. Caianiello, Via Campi Flegrei 34, 80078 Pozzuoli, Italy.
Present address: SciGenom Laboratories, Cochin, Kerala 682037, India.
Present address: Laboratoire de Reproduction et Développement des Plantes, École Normale Supérieure de Lyon 46, Allée d’Italie, 69364 Lyon cedex 07, France.
Address correspondence to [email protected].
The author responsible for distribution of materials integral to the findings presented in this article in accordance with the policy described in the Instructions for Authors (www.plantphysiol.org) is: Stefania Grillo ([email protected]).
Articles can be viewed without a subscription.