-
PDF
- Split View
-
Views
-
Cite
Cite
Eigo Ando, Masato Ohnishi, Yin Wang, Tomonao Matsushita, Aiko Watanabe, Yuki Hayashi, Miho Fujii, Jian Feng Ma, Shin-ichiro Inoue, Toshinori Kinoshita, TWIN SISTER OF FT, GIGANTEA, and CONSTANS Have a Positive But Indirect Effect on Blue Light-Induced Stomatal Opening in Arabidopsis , Plant Physiology, Volume 162, Issue 3, July 2013, Pages 1529–1538, https://doi.org/10.1104/pp.113.217984
- Share Icon Share
Abstract
FLOWERING LOCUS T (FT) is the major regulatory component controlling photoperiodic floral transition. It is expressed in guard cells and affects blue light-induced stomatal opening induced by the blue-light receptor phototropins phot1 and phot2. Roles for other flowering regulators in stomatal opening have yet to be determined. We show in Arabidopsis (Arabidopsis thaliana) that TWIN SISTER OF FT (TSF), CONSTANS (CO), and GIGANTEA (GI) provide a positive effect on stomatal opening. TSF, which is the closest homolog of FT, was transcribed in guard cells, and light-induced stomatal opening was repressed in tsf-1, a T-DNA insertion mutant of TSF. Overexpression of TSF in a phot1 phot2 mutant background gave a constitutive open-stomata phenotype. Then, we examined whether CO and GI, which are upstream regulators of FT and TSF in photoperiodic flowering, are involved in stomatal opening. Similar to TSF, light-induced stomatal opening was suppressed in the GI and CO mutants gi-1 and co-1. A constitutive open-stomata phenotype was observed in GI and CO overexpressors with accompanying changes in the transcription of both FT and TSF. In photoperiodic flowering, photoperiod is sensed by photoreceptors such as the cryptochromes cry1 and cry2. We examined stomatal phenotypes in a cry1 cry2 mutant and in CRY2 overexpressors. Light-induced stomatal opening was suppressed in cry1 cry2, and the transcription of FT and TSF was down-regulated. In contrast, the stomata in CRY2 overexpressors opened even in the dark, and FT and TSF transcription was up-regulated. We conclude that the photoperiodic flowering components TSF, GI, and CO positively affect stomatal opening.
Stomatal pores are surrounded by a pair of guard cells in the plant epidermis and control gas exchange in response to environmental and endogenous signals (Schroeder et al., 2001; Shimazaki et al., 2007). Previous studies have revealed that blue light acts as a signal that induces stomatal opening. Blue light is perceived by the receptor kinase phototropins phot1 and phot2 and activates the plasma membrane H+-ATPase (Kinoshita and Shimazaki, 1999; Kinoshita et al., 2001). Blue light-activated H+-ATPase creates inside-negative electrical potential across the plasma membrane and drives K+ uptake through voltage-gated inward-rectifying K+ channels. As a consequence, changes in guard cell turgor and volume lead to stomatal opening (Kinoshita and Hayashi, 2011). Meanwhile, the plasma membrane anion channel has been shown to play a major role in abscisic acid (ABA)-induced stomatal closure. The anion channel causes depolarization of the plasma membrane itself and drives K+ efflux through voltage-gated outward-rectifying K+ channels (Schroeder et al., 1987, 2001). In addition, the plasma membrane anion channel is reported to be inhibited by blue light in a phototropin-dependent manner (Marten et al., 2007). Despite these recent studies, however, the mechanism controlling stomatal aperture has yet to be completely determined.
Recently, the suppressor of closed-stomata phenotype in phot1 phot2 (scs1-1) mutant was isolated from among ethyl methanesulfonate-treated phot1 phot2 double mutants. The scs1-1 mutant exhibits a constitutive open-stomata phenotype and carries a novel null allele of early flowering3 (elf3; Kinoshita et al., 2011). ELF3 is reported to act as a negative regulator of the florigen FLOWERING LOCUS T (FT) during floral induction (Kim et al., 2005), and the guard cells of scs1-1 (elf3 phot1 phot2 triple mutant) have high levels of FT transcription and H+-ATPase activity. Overexpression of FT in guard cells promotes stomatal opening. In the loss-of-function FT mutant ft-1, both blue light-induced stomatal opening and activation of H+-ATPase were repressed, with no effect on the amounts of phototropins or H+-ATPase. These results suggest that FT provides a positive effect on stomatal opening via the activity of H+-ATPase in guard cells (Kinoshita et al., 2011). Overexpression of APELATA1 (AP1), which is a MADS box transcription factor known to be a downstream target of FT in floral induction (Abe et al., 2005; Wigge et al., 2005), showed an open-stomata phenotype, suggesting that FT-mediated stomatal opening involves transcriptional regulation (Kinoshita et al., 2011).
FT was identified as the floral inducer florigen (Corbesier et al., 2007). FT encodes a small protein of approximately 20 kD that belongs to a subfamily in the phosphatidylethanolamine-binding protein family (Kardailsky et al., 1999; Kobayashi et al., 1999; Ahn et al., 2006). FT is synthesized in the phloem companion cells of leaves in response to an appropriate photoperiod for flowering and is then transported to the shoot apical meristem, where it interacts with FLOWERING LOCUS D (FD), a bZIP-type transcriptional factor, to induce the expression of floral identity genes such as AP1 (Abe et al., 2005; Wigge et al., 2005; Turck et al., 2008). TWIN SISTER OF FT (TSF) is the closest known homolog of FT; it is regulated by photoperiod and induces floral transition redundantly with FT (Yamaguchi et al., 2005).
CONSTANS (CO), a zinc-finger transcription factor, is one of the key regulators of photoperiodic floral transition. CO directly regulates the transcription of FT and probably TSF (Suárez-López et al., 2001; Yamaguchi et al., 2005). In Arabidopsis (Arabidopsis thaliana), CO is transcribed only in the late afternoon under long-day conditions and induces FT and TSF transcription (Yamaguchi et al., 2005; Sawa et al., 2007). Expression of CO is regulated by the circadian clock component GIGANTEA (GI; Fowler et al., 1999; Park et al., 1999). The circadian expression of GI reaches a peak before dusk under long-day conditions (Turck et al., 2008). The GI protein interacts with the FLAVIN-BINDING, KELCH REPEAT, F-BOX1 (FKF1) protein and binds the CO promoter in late afternoon. The GI-FKF1 complex degrades CYCLING DOF FACTOR1, which is a clock-controlled transcriptional repressor of CO. Thus, formation of the GI-FKF1 complex in late afternoon is crucial for the expression of CO with appropriate timing (Sawa et al., 2007). The GI-FKF1 complex also binds the FT promoter and promotes FT transcription in a CO-independent manner (Sawa and Kay, 2011). Moreover, FKF1 stabilizes the CO protein to promote FT transcription (Song et al., 2012). Daylength is sensed by photoreceptors such as phytochromes (phyA and phyB) and cryptochromes (cry1 and cry2; Mockler et al., 2003; Boss et al., 2004). Cryptochromes are known to be involved in the entrainment of the circadian clock (Somers et al., 1998). The regulation of GI protein stability by cry2 and ELF3 confers an accurate circadian rhythm on GI expression (Yu et al., 2008). CO protein is stabilized in late afternoon by cry2 and phyA but is destabilized by phyB in the early morning (Valverde et al., 2004; Liu et al., 2008). Thus, many components are involved in the control of photoperiodic floral transition. The floral transition genes PHYA, PHYB, CRY1, CRY2, GI, and CO are all known to be transcribed in guard cells (Kinoshita et al., 2011), but a potential role for these genes in the regulation of stomatal opening in terms of photoperiodic flowering components had not been studied.
In this study, we show that the FT homolog TSF is also transcribed in guard cells and has a positive effect on stomatal opening. Our results indicate that the flowering regulators GI and CO are involved in stomatal opening, with accompanying changes in the transcription of both FT and TSF. We also show that blue light-induced stomatal opening is suppressed in a cry1 cry2 double mutant with down-regulation of FT and TSF transcription and that CRY2-overexpressing plants show up-regulation of FT and TSF transcription and an open-stomata phenotype even in the dark. From these results, we conclude that stomatal opening is affected by a mechanism similar to that of the photoperiodic floral transition involving photoreceptor cryptochromes, the floral inducers FT and TSF, and the upstream flowering components GI and CO.
RESULTS
TSF Has a Positive Effect on Stomatal Opening
FT is expressed in guard cells and provides a positive effect on stomatal opening (Kinoshita et al., 2011). In Arabidopsis, TSF is the closest known homolog of FT, and it regulates photoperiodic flowering redundantly with FT (Yamaguchi et al., 2005). Transcription of TSF, like FT, was detected in highly purified guard cell protoplasts (GCPs), as shown in Figure 1A. Stomata in wild-type plants opened in response to light, but light-induced stomatal opening was suppressed in ft-2, a loss-of-function mutant of FT (Kinoshita et al., 2011). Moreover, stomatal responses in tsf-1, a T-DNA insertion mutant of TSF (Yamaguchi et al., 2005), were also suppressed (Fig. 1, B and C). These results suggest that TSF also has a positive effect on stomatal opening similar to FT. To test the functional redundancy between TSF and FT, we analyzed the stomatal responses of an ft-2 tsf-1 double mutant. Unexpectedly, we could not detect significant differences between the ft-2 tsf-1 double mutant and the individual single mutants, although the average stomatal aperture in ft-2 tsf-1 was slightly lower than those in the single mutants. Note that the stomata in these mutants opened in response to the fungal toxin fusicoccin (FC), which induces stomatal opening via irreversible activation of H+-ATPase (Kinoshita and Shimazaki, 2001), suggesting that these mutations may not affect signal transduction components downstream of the H+-ATPase.
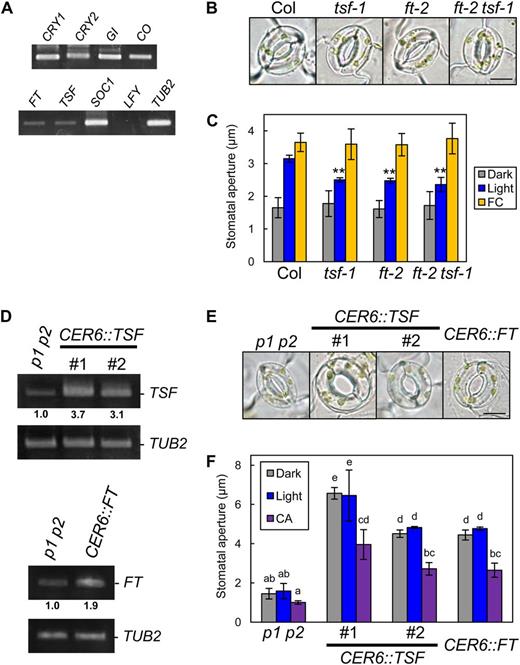
TSF is transcribed in guard cells and provides positive regulation of stomatal opening. A, Reverse transcription (RT)-PCR analyses of flowering components (CRY1, CRY2, COP1, GI, CO, FT, TSF, SOC1, and LFY) in GCPs. TUBELIN BETA CHAIN2 (TUB2) was used as a control. B, Typical stomata in Col, tsf-1, ft-2, and the ft-2 tsf-1 double mutant. The epidermis was illuminated as described below. Bar = 10 µm. C, Stomatal apertures under different treatments. Epidermal fragments were kept in the dark (Dark), illuminated with 10 µmol m–2 s–1 blue light under a background 50 µmol m–2 s–1 red light (Light), or treated with 10 µm FC in the dark (FC) for 3 h. Data represent means of three independent experiments with sd. Asterisks indicate significant differences between each mutant and Col under the same condition (**P < 0.01; Student’s t test). D, RT-PCR analyses of FT, TSF, and TUB2 in leaf epidermal fragments of phot1 phot2 (p1 p2), pCER6::TSF/phot1 phot2 (CER6::TSF), and pCER6::FT/phot1 phot2 (CER6::FT). #, Line number of transgenic plants. Epidermal fragments were isolated at zeitgeber time 12. Numbers below each signal represent the relative expression levels for the same genes. Signal values of each gene were estimated by ImageJ software (National Institutes of Health) and normalized to those of TUB2 and secondarily normalized to corresponding phot1 phot2 values set to 1.0. Similar results were obtained in independent experiments. E, Typical stomata of phot1 phot2 and the transgenic plants described in D. The epidermis was kept in the dark as in C. Bar = 10 µm. F, Stomatal apertures of the transgenic plants under different treatments. Dark or light conditions were the same as in C. CA was at 0.5 µm. The epidermis was treated with CA under light-exposure conditions for 3 h. Data represent means of three independent experiments with sd. Different letters indicate significant differences among means (P < 0.05; Tukey’s test).
We generated transgenic plants with the CER6 promoter (Hooker et al., 2002) to obtain constitutive TSF expression in the epidermis, including the guard cells in a phot1 phot2 background. As expected, TSF-overexpressing plants (pCER6::TSF/phot1 phot2) showed a constitutive open-stomata phenotype, as seen in FT-overexpressing plants (Kinoshita et al., 2011; Fig. 1, D–F). The stomata in TSF-overexpressing plants closed in response to the type 1/type 2A protein phosphatase inhibitor calyculin A (CA; Ishihara et al., 1989), which inhibits the blue light-dependent activation of H+-ATPase in guard cells (Kinoshita and Shimazaki, 1997; Hayashi et al., 2011; Fig. 1F) and ABA (Supplemental Fig. S1). Note that an early-flowering phenotype was observed in TSF-overexpressing plants (Supplemental Fig. S2).
Photoperiodic Flowering Regulators CO and GI Are Involved in FT- and TSF-Mediated Stomatal Opening
We examined whether CO and GI, which are upstream regulators of FT and TSF during the photoperiodic floral transition (Yamaguchi et al., 2005; Turck et al., 2008), are involved in stomatal opening. As shown in Figure 1A, expression of CO and GI was observed in GCPs (Kinoshita et al., 2011). A loss-of-function mutant of CO, co-1 (Robson et al., 2001), showed decreased FT, and TSF transcript levels in the epidermis and light-induced stomatal opening were repressed (Fig. 2). In contrast, the stomata in CO-overexpressing plants (pCER6::CO/phot1 phot2) opened as in TSF-overexpressing plants, with high levels of both FT and TSF transcripts detected in the epidermis (Fig. 3). Similarly, a loss-of-function mutant of GI, gi-1 (Fowler et al., 1999), had low levels of FT and TSF transcripts in the epidermis; light-induced stomatal opening was suppressed, and a constitutive open-stomata phenotype was observed in GI-overexpressing plants (pCER6::GI/phot1 phot2), with high levels of FT and TSF transcripts detected in the epidermis (Figs. 2 and 3). Note that CO- and GI-overexpressing plants showed early-flowering phenotypes and that the transcription of CO was up-regulated in the epidermis of GI-overexpressing plants (Supplemental Fig. S3). In addition, FC induced stomatal opening in gi-1 and co-1 (Fig. 2C), and both CA and ABA induced stomatal closure in the GI- and CO-overexpressing plants (Fig. 3C; Supplemental Fig. S1). These results suggest that GI and CO are also involved in stomatal opening and that the sequence of regulatory action is GI, CO, and then FT/TSF, as it is for the regulation of photoperiodic floral transition. Note that TSF-, GI-, and CO-overexpressing plants and tsf-1, ft-2 tsf-1, co-1, and gi-1 mutants showed similar stomatal index compared with background plants (Supplemental Table S4).
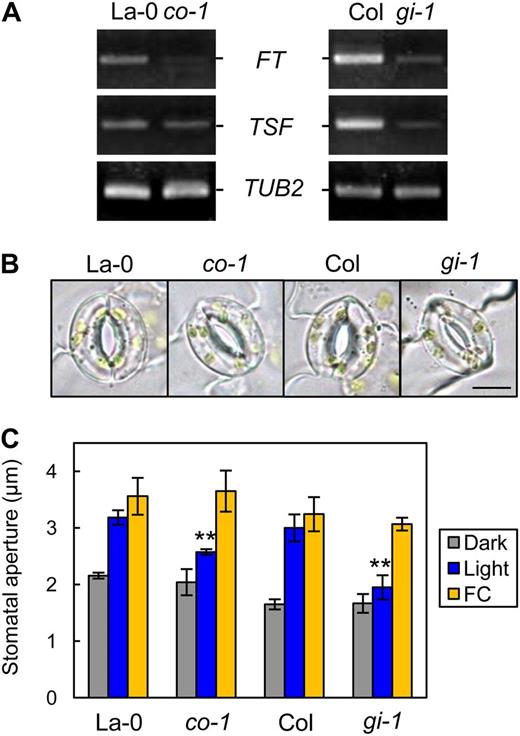
Light-induced stomatal opening was suppressed in co-1 and gi-1, and the transcription of FT and TSF was down-regulated. A, RT-PCR analyses of FT, TSF, and TUB2 in leaf epidermal fragments of La-0, co-1, Col, and gi-1. Epidermal fragments were isolated at zeitgeber time 12. B, Typical stomata of the mutant and corresponding background plants described in A. The epidermis was illuminated as described below. Bar = 10 µm. C, Stomatal apertures under different treatments. Epidermal fragments were kept in the dark (Dark), illuminated with 10 µmol m–2 s–1 blue light under a background 50 µmol m–2 s–1 red light (Light), or treated with 10 µm FC in the dark (FC) for 3 h. Data represent means of three independent experiments with sd. Asterisks indicate significant differences between each mutant and the corresponding wild type under the same conditions (**P < 0.01; Student’s t test).
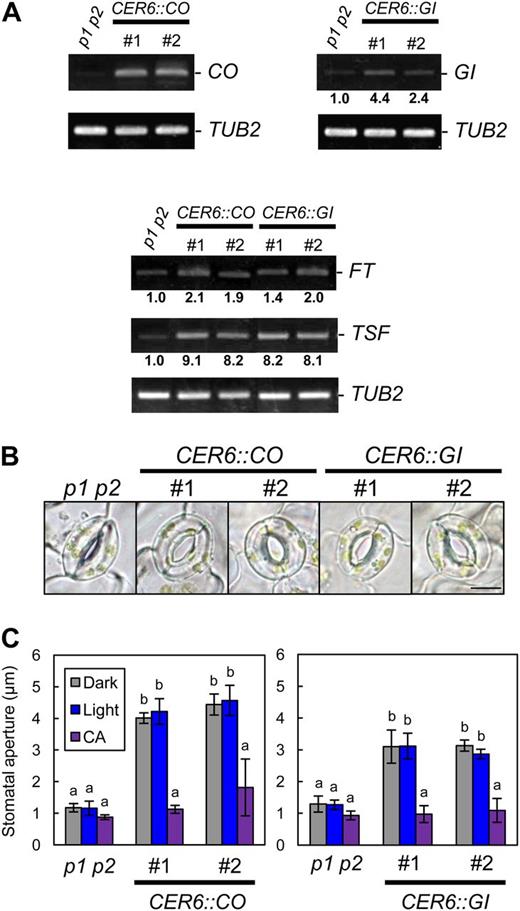
Overexpression of CO or GI promoted stomatal opening and the up-regulation of FT and TSF transcription. A, RT-PCR analyses of GI, CO, FT, TSF, and TUB2 in leaf epidermal fragments of phot1 phot2 (p1 p2), pCER6::CO/phot1 phot2 (CER6::CO), and pCER6::GI/phot1 phot2 (CER6::GI). #, Line number of transgenic plants. Epidermal fragments were isolated at zeitgeber time 4 for the detection of CO and GI and at zeitgeber time 12 for FT and TSF. Signal values are estimated as in Figure 1D. B, Typical stomata of phot1 phot2 and the transgenic plants described in A. The epidermis was kept in the dark for 3 h. Bar = 10 µm. C, Stomatal apertures of the transgenic plants under different treatments. Epidermal fragments were kept in the dark (Dark) or illuminated with 10 µmol m–2 s–1 blue light under a background 50 µmol m–2 s–1 red light (Light) for 3 h. CA was at 0.5 µm. The epidermis was treated with CA under light-exposure conditions for 3 h. Data represent means of three independent experiments with sd. Different letters indicate significant differences between the means of phot1 phot2 and each transgenic plant (P < 0.05; Tukey’s test).
Cryptochromes Affect Stomatal Aperture via the Regulation of FT/TSF Transcription
Cryptochromes are well-understood blue light photoreceptors that regulate photoperiodic floral transition through entrainment of the circadian clock (Somers et al., 1998; Mockler et al., 2003) and by the regulation of GI and CO protein stability (Valverde et al., 2004; Liu et al., 2008; Yu et al., 2008). Cryptochromes are also transcribed in GCPs (Fig. 1A; Kinoshita et al., 2011). We hypothesized that cryptochromes affect stomatal opening through their activity as photoperiod-sensing light receptors. To test this hypothesis, we examined stomatal responses in a cry1 cry2 double mutant. In cry1 cry2, FT and TSF transcript levels decreased in the epidermis and light-induced stomatal opening was suppressed compared with the wild type (Fig. 4, A–C). Overexpression of CRY2 in the cry1 cry2 background (35S::CRY2-GFP/cry1 cry2) gave increased FT and TSF transcript levels in the epidermis, and the stomata of the CRY2-overexpressing plants opened even in the dark (Fig. 4, D–F). These results suggest that cryptochromes most likely affect stomatal aperture via the regulation of FT and TSF transcription in response to photoperiod.
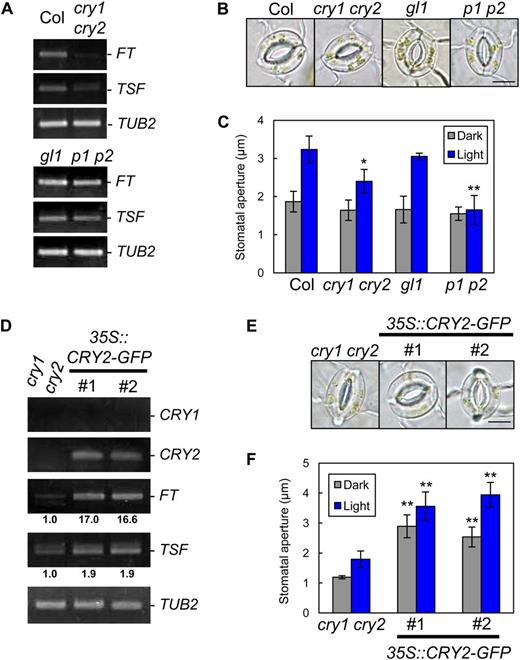
Regulation of stomatal aperture by cryptochromes is likely to be mediated by the regulation of FT and TSF. A, RT-PCR analyses of FT, TSF, and TUB2 in leaf epidermal tissue of Col, cry1 cry2, gl1, and phot1 phot2 (p1 p2). Epidermal fragments were isolated at zeitgeber time 12. B, Typical stomata of the same plants described in A. The epidermis was illuminated as described below. Bar = 10 µm. C, Stomatal apertures under different treatments. Epidermal fragments were kept in the dark (Dark), illuminated with 10 µmol m–2 s–1 blue light under a background 50 µmol m–2 s–1 red light (Light), or treated with 10 µm FC in the dark (FC) for 3 h. Data represent means of three independent experiments with sd. Asterisks indicate significant differences between each mutant and the corresponding background plants under the same conditions (*P < 0.05, **P < 0.01; Student’s t test). D, RT-PCR analyses of CRY1, CRY2, FT, TSF, and TUB2 in leaf epidermal tissue of cry1 cry2 and CaMV35S::CRY2-GFP/cry1 cry2 (35S::CRY2-GFP). #, Line number of transgenic plants. Epidermal fragments were isolated at zeitgeber time 12. Signal values are estimated as in Figure 1D. E, Typical stomata of the same plants described in D. The epidermis was kept in the dark for 3 h. Bar = 10 µm. F, Stomatal apertures of the transgenic plants under different treatments. Epidermal fragments were kept in the dark (Dark) or illuminated with 10 µmol m–2 s–1 blue light under a background 50 µmol m–2 s–1 red light (Light) for 3 h. Data represent means of three independent experiments with sd. Asterisks indicate significant differences between transgenic plants and cry1 cry2 under the same conditions (**P < 0.01; Student’s t test).
Phototropins Are the Blue Light Receptors That Mediate a Fast Stomatal Response to Blue Light
Cryptochromes are suggested to function additively with phototropins in blue light-induced stomatal opening (Mao et al., 2005). However, as shown in Figure 4C, stomata in phot1 phot2 completely failed to open in response to light, even though they expressed cryptochromes at wild-type levels (Kinoshita et al., 2011). The transcription of FT and TSF was not affected in phot1 phot2 (Fig. 4A), indicating that the loss of light-induced stomatal opening in phot1 phot2 was not due to a down-regulation of FT and TSF transcription. To examine the stomatal response to blue light in more detail in phot1 phot2 and a corresponding background plant, glabra1 (gl1), we measured stomatal conductance in intact leaves using a gas-exchange system. As shown in Figure 5, stomatal conductance in the background plant gl1 showed a clear increase in response to blue light illumination for 15 min at 5 µmol m–2 s–1 superimposed on background red light. In contrast, blue light-induced fluctuation of stomatal conductance was completely abolished in phot1 phot2 leaves even under a high fluence rate of blue light (20 µmol m–2 s–1) for 15 min. These results are consistent with those found for stomatal aperture (Fig. 4C) and demonstrate that phototropins, but not cryptochromes, are the only blue light receptors that can directly induce a fast stomatal response to blue light.
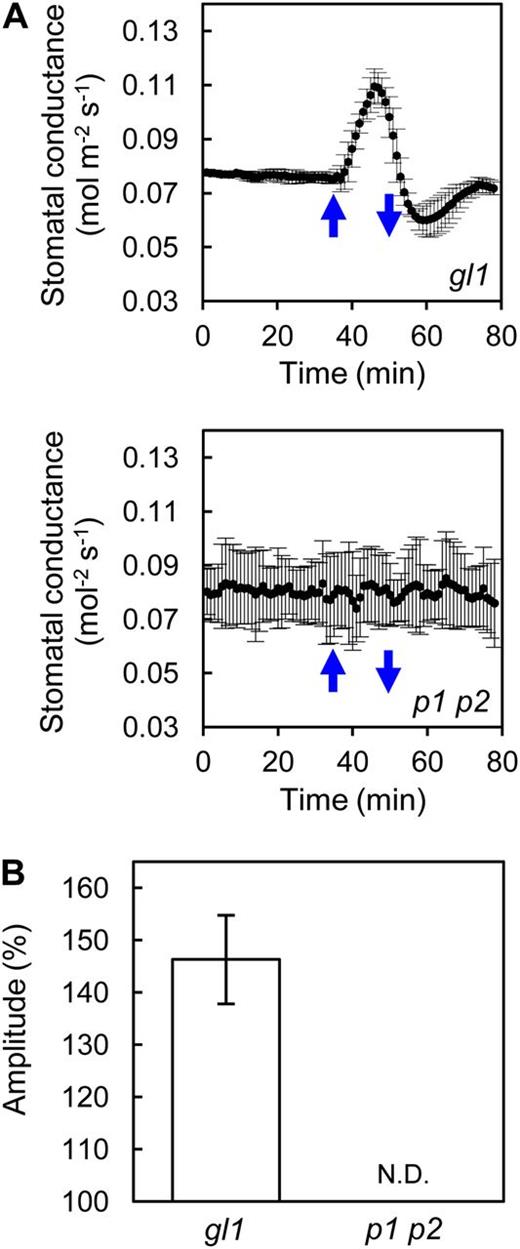
Stomatal conductance in phot1 phot2 in response to short-term blue light irradiation. A, Fluctuation of stomatal conductance in phot1 phot2 (p1 p2) and corresponding background plant (gl1) leaves in response to blue light. Leaves were illuminated with a strong red light (550 µmol m–1 s–1) as a background to stabilize the conductance before illumination with blue light. Upward and downward blue arrows indicate the start and termination, respectively, of illumination for 15 min with blue light (20 µmol m–1 s–1 for phot1 phot2 and 5 µmol m–1 s–1 for gl1). Data represent means of three independent experiments with sd. B, Amplitude of the stomatal conductance in response to blue light. The amplitude is shown as a percentage against a steady state set to 100%. Data represent means of three independent experiments with sd. N.D., Not detected.
DISCUSSION
Roles for Photoperiodic Flowering Regulators in the Regulation of Stomatal Opening
A previous study indicated that the Arabidopsis floral pathway integrator FT is expressed in stomatal guard cells and provides a positive effect on stomatal opening by affecting plasma membrane H+-ATPase activity in the guard cells, and a transcriptional factor, such as AP1, is suggested to be involved in FT-mediated stomatal opening (Kinoshita et al., 2011). In this study, we showed that the FT homolog TSF is also transcribed in guard cells and has a positive effect on stomatal opening (Fig. 1). We also showed that the photoperiodic flowering components GI and CO are involved in stomatal opening, with accompanying changes in the transcription of both FT and TSF, and that cryptochromes, which function in sensing photoperiod, affect FT/TSF transcription and stomatal aperture (Figs. 2–4). Stomatal opening is induced by blue light under background red light; however, it is also stimulated by red light only in both intact leaves and the isolated epidermis (Kinoshita et al., 2001; Shimazaki et al., 2007). In our conditions, stomatal responses to red light in the isolated epidermis were not affected in the mutants used in this study (Supplemental Fig. S4). These results indicate that a regulatory mechanism similar to that employed for photoperiodic floral transition has a positive but indirect effect on blue light-induced stomatal opening. We propose a possible model for stomatal opening directly induced by blue light signaling and indirectly affected by the photoperiodic pathway (Fig. 6). In this model, FT or TSF affects the blue light signaling component(s) between phototropins and H+-ATPase. Note that overexpression of FT or TSF promoted stomatal opening even in the absence of functional phototropins (Figs. 1F and 3C). FT or TSF overexpression might lead to the up-regulation or activation of an unidentified component(s) in blue light signaling independent of the perception of blue light.
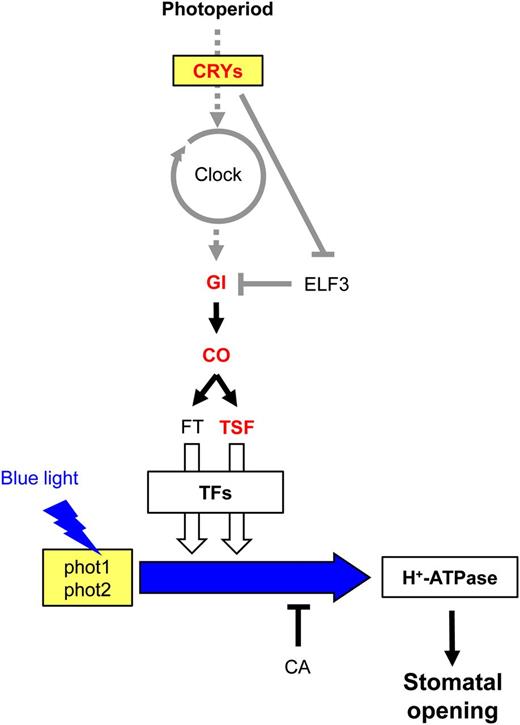
A possible model for FT/TSF-mediated stomatal opening. The photoperiodic pathway is shown with representative components. The blue light signaling pathway between phototropins and the H+-ATPase is shown with a blue arrow. Arrows and T-bars indicate positive and negative regulation, respectively. Dotted arrows represent input and output of the circadian clock. Gene names in red represent components that were directly analyzed in this study. ELF3 and FT were described previously (Kinoshita et al., 2011). TFs represents transcription factors working downstream of FT and TSF, such as an AP1. Gray lines indicate regulatory mechanisms that are reported in previous works. White arrows show a contribution to stomatal opening, and the thickness of the arrows represents the possible degree of the contribution (for details, see text).
In this study, light-induced stomatal opening in tsf-1 was suppressed to the same extent as in ft-2, indicating that the contributions of TSF and FT to stomatal opening are comparable (see white arrows from FT and TSF in Fig. 6). However, we could not detect significant differences between the ft-2 tsf-1 double mutant and the individual single mutants, although the stomatal aperture was slightly smaller in the double mutant than in the single mutants. More detailed investigation is needed to clarify whether TSF and FT have redundant roles in stomatal opening. An unidentified component(s) that affects blue light-induced stomatal opening in parallel to FT and TSF might exist, because the ft-2, tsf-1, and ft-2 tsf-1 mutants exhibited light-induced stomatal opening. SUPPRESSOR OF OVEREXPRESSION OF CONSTANS1 (SOC1) and LEAFY (LFY) are known to function as floral pathway integrators in addition to FT and TSF (Araki, 2001; Simpson and Dean, 2002; Boss et al., 2004; Yamaguchi et al., 2005). Indeed, we detected SOC1 transcripts in wild-type GCPs but not in LFY transcripts (Fig. 1A). Further investigation is needed to clarify the involvement of other floral pathway integrators in stomatal opening.
Molecular Basis for FT- and TSF-Mediated Stomatal Opening
The plasma membrane H+-ATPase functions as a key enzyme in the regulation of stomatal opening in guard cells (Kinoshita and Hayashi, 2011). Activation of the H+-ATPase is mediated by phosphorylation of the penultimate Thr in the C terminus and subsequent binding of the 14-3-3 protein to the phosphorylated C terminus (Kinoshita and Shimazaki, 1999, 2002). We show that the stomata in the loss-of-function mutants of GI, CO, FT, and TSF opened in response to FC (Figs. 1C and 2C), which induces stomatal opening via the accumulation of phosphorylated H+-ATPase in guard cells (Kinoshita and Shimazaki, 2001). This suggests that regulatory components downstream of the H+-ATPase that are responsible for stomatal opening are functional/intact in these mutants. Stomata in transgenic plants overexpressing GI, CO, FT, or TSF can close when they are treated with CA (Figs. 1F and 3C), which inhibits blue light-induced activation/phosphorylation of the guard cell H+-ATPase (Kinoshita and Shimazaki, 1997; Hayashi et al., 2011). A previous study showed that the blue light-induced phosphorylation of H+-ATPase in guard cells was impaired in ft-1, a loss-of-function mutant of FT (Kinoshita et al., 2011). These combined results suggest that GI, CO, and TSF affect stomatal aperture, at least in part, by affecting the phosphorylation status of H+-ATPase in guard cells. Further investigation will be needed to clarify how FT and TSF affect the phosphorylation status of H+-ATPase in guard cells and the molecular mechanism of FT/TSF-mediated stomatal opening. In addition, phototropins are reported to inhibit the plasma membrane anion channels in guard cells (Marten et al., 2007). As anion channels are key components in stomatal closure (Schroeder et al., 2001), it is possible that FT or TSF, in part, negatively affects the anion channel to promote stomatal opening.
Role of Cryptochromes in the Regulation of Stomatal Opening
Our results here indicate that phototropins, but not cryptochromes, directly induce the fast stomatal response to blue light (Figs. 4C and 5). However, light-induced stomatal opening was suppressed in cry1 cry2 even though the stomata still exhibited a light response (Fig. 4C). Overexpression of CRY2 promoted the up-regulation of FT and TSF transcription and enhanced stomatal opening, even in the dark (Fig. 4, D–F). Previous studies have shown that cryptochromes are involved in sensing photoperiod to entrain the circadian clock (Somers et al., 1998; Mockler et al., 2003) and in the stabilization of GI and CO proteins (Valverde et al., 2004; Liu et al., 2008; Yu et al., 2008). Thus, cryptochromes most likely affect phototropin-mediated stomatal opening by acting as light receptors that sense photoperiod as a long-term signal leading to the regulation of FT and TSF transcription (Fig. 6).
A recent study indicated that cryptochromes affect stomatal conductance by controlling the amount of endogenous ABA in leaves (Boccalandro et al., 2012). Endogenous ABA has been suggested to affect the activity of the plasma membrane H+-ATPase in guard cells even in the absence of drought stress (Hayashi et al., 2011). These findings suggest that endogenous ABA may have an important role in the regulation of stomatal aperture even under nonstress conditions. The relationship between photoperiod and ABA content in guard cells is an interesting problem for further investigation.
Physiological Significance of FT/TSF-Mediated Stomatal Opening
Several studies have shown that FT and its orthologs have multiple physiological functions apart from the regulation of floral transition (Pin and Nilsson, 2012). Such functions include the regulation of growth (Gyllenstrand et al., 2007; Shalit et al., 2009), seed germination (Chiang et al., 2009), fruit set (Lifschitz et al., 2006), stomatal opening (Kinoshita et al., 2011), and tuberization (Navarro et al., 2011; González-Schain et al., 2012). Our results suggest that in addition to FT, TSF and the floral regulators GI and CO promote stomatal opening. The promotion of stomatal opening by these components may lead to the acceleration of photosynthesis prior to both flowering and seed development. Plant growth and reproduction are thought to entail the regulation of metabolism, and FT/TSF may play a part via the promotion of photosynthesis. Further study on multiple physiological functions of FT/TSF will provide a better understanding of the mechanisms involved in plant growth and reproduction.
MATERIALS AND METHODS
Plant Materials and Growth Conditions
Arabidopsis (Arabidopsis thaliana) gl1, Columbia (Col), and Landsberg (La-0) plants were used as the background ecotypes of the mutants and overexpression plants. Col is the background ecotype of gi-1, ft-2 (introgressed; Kobayashi et al., 1999), tsf-1 (SALK_087522; Yamaguchi et al., 2005), and cry1-304 cry2-1 (cry1 cry2; Mockler et al., 2003). phot1-5 phot2-1 (phot1 phot2; Kinoshita et al., 2001) is in the gl1 background. La-0 is the background ecotype of co-1. Seeds for gi-1 and co-1 were obtained from the Arabidopsis Biological Resource Center. All plants were grown in soil under a 16-h-white light (50 µmol m–2 s–1)/8-h-dark cycle at 22°C to 24°C in 50% to 70% relative humidity in a growth room. The ft-2 tsf-1 double mutant was obtained by crossing tsf-1 with ft-2.
Measurement of Stomatal Apertures
Epidermal fragments were isolated from mature rosette leaves of 4- to 6-week-old plants as described previously (Inoue et al., 2008). For the analysis of stomatal apertures, epidermal fragments isolated from dark-adapted plants were dipped into a basal buffer {5 mm MES-1,3-bis(tris[hydroxymethyl]methylamino) propane [pH 6.5], 50 mm KCl, and 0.1 mm CaCl2} and illuminated with blue light at 10 µmol m–2 s–1 superimposed on a background red light at 50 µmol m–2 s–1 for 3 h at room temperature. CA (0.5 µm) was added to the basal buffer under light-exposure conditions, and 10 µm FC was added in the dark. CA and FC were dissolved in dimethyl sulfoxide. Twenty-five stomatal apertures (five stomata per epidermal fragment) in the abaxial epidermis were measured microscopically for each independent experiment, treatment, and genotype. All data represent means of three independent experiments with sd.
Gas-Exchange Measurements
Gas-exchange measurements were performed using the Li-6400 system (Li-Cor), and parameters were calculated with the software supplied by the manufacturer. Mature leaves from Arabidopsis plants were clamped in a standard Li-6400 chamber and illuminated from the adaxial side with background red light at 550 µmol m–2 s–1. When the photosynthetic rate and stomatal conductance reached their steady states, a weak blue light (20 µmol m–2 s–1 for phot1 phot2 and 5 µmol m–2 s–1 for gl1) was superimposed on the background red light for 15 min. Flow rate, leaf temperature, relative humidity, and ambient CO2 concentration were kept constant at 500 µmol s–1, 24°C, 30% to 40%, and 400 µL L–1, respectively. Data represent means of three independent experiments with sd.
Construction of Transformation Vectors and Transformation of Plants
All of the plant transformation plasmid vectors listed in Supplemental Table S1 were generated using standard molecular biology procedures and introduced into Agrobacterium tumefaciens (GV3101), which was then transformed into plants using a standard method. Homozygous plants were used for the experiments. Primers are shown in Supplemental Table S2.
Light Source
Light-emitting photodiodes (ISL-150X150-RB and ISC-201-2 power supply; CCS) served as red and blue light sources for the stomatal opening measurements. Halogen projector lamps (150-W and MHAB-150W power supply; Moritex) with red or blue filters (Daiken) were used as red or blue light sources for the gas-exchange measurements. Photon flux densities were determined with a quantum meter (Li-250; Li-Cor).
Reverse Transcription-PCR
Epidermal fragments that included stomatal guard cells were isolated from mature rosette leaves of 4- to 6-week-old plants using a blender equipped with an MC1 mini container (Waring Commercial). Mature rosette leaves (0.1–0.2 g) were blended for approximately 20 s in 35 mL of ice-cold Milli-Q water (Millipore). The epidermal fragments were collected on 58-µm nylon mesh and frozen in a tube with liquid nitrogen. The epidermal fragments were isolated at zeitgeber times as indicated. The GCPs were prepared enzymatically from 4- to 6-week-old plants as described previously (Ueno et al., 2005).
Total RNAs were extracted from epidermal fragments and GCPs with an RNeasy Plant Mini Kit (Qiagen) and on-column DNase treatment using the RNase-Free DNase Set (Qiagen), and first-strand complementary DNAs were synthesized using the PrimeScript II First-Strand cDNA Synthesis Kit (Takara) following the manufacturer’s instructions. In Figure 1A, 35 cycles of PCR were performed for all genes. In Figures 1D, 2A, 3A, and 4, A and D, 25 cycles of PCR were performed for GI, 30 cycles for CRY1, CRY2, CO, and TUB2, and 35 cycles for FT and TSF. Primers are shown in Supplemental Table S3.
Statistical Significance Test
The significance of differences in means between genotypes was assessed by the two-tailed unpaired Student’s t test. Asterisks in the figures indicate significant differences for the samples under the same condition. Differences among means were analyzed by Tukey’s multiple comparison tests. Different letters in the figures indicate significant differences among means.
Sequence data from this article can be found in the GenBank/EMBL data libraries under accession numbers CRY1 (AT4G08920), CRY2 (AT1G04400), GI (AT1G22770), CO (AT5G15840), FT (AT1G65480), TSF (AT4G20370), SOC1 (AT2G45660), LFY (AT5G61850), and TUB2 (AT5G62690).
Supplemental Data
The following materials are available in the online version of this article.
Supplemental Figure S1. ABA-induced stomatal closure in transgenic plants.
Supplemental Figure S2. Phenotypes of TSF-overexpressing plants.
Supplemental Figure S3. Phenotypes of GI- and CO-overexpressing plants.
Supplemental Figure S4. Effect of red light on stomatal opening.
Supplemental Table S1. List of transformation vectors.
Supplemental Table S2. Primer sets for construction.
Supplemental Table S3. Primer sets for RT-PCR.
Supplemental Table S4. Stomatal index in the transgenic plants and mutants.
ACKNOWLEDGMENTS
We thank M. Goto (Nagoya University) for technical assistance. ft-2 (introgressed) was kindly provided by Dr. T. Araki (Kyoto University).
Glossary
- ABA
abscisic acid
- GCPs
guard cell protoplasts
- FC
fusicoccin
- CA
calyculin A
- Col
Columbia
- RT
reverse transcription
- La-0
Landsberg
LITERATURE CITED
Author notes
This work was supported by Grants-in-Aid from the Ministry of Education, Culture, Sports, Science, and Technology, Japan (grant nos. 22119005, 21227001, and 21027014 to T.K.), and by Advanced Low Carbon Technology Research and Development Program from the Japan Science and Technology Agency (to T.K.).
Corresponding author; e-mail [email protected].
The author responsible for distribution of materials integral to the findings presented in this article in accordance with the policy described in the Instructions for Authors (www.plantphysiol.org) is: Toshinori Kinoshita ([email protected]).
The online version of this article contains Web-only data.
Open Access articles can be viewed online without a subscription.