-
PDF
- Split View
-
Views
-
Cite
Cite
Jun Yang, Lei Tian, Ming-Xi Sun, Xue-Yong Huang, Jun Zhu, Yue-Feng Guan, Qi-Shi Jia, Zhong-Nan Yang, AUXIN RESPONSE FACTOR17 Is Essential for Pollen Wall Pattern Formation in Arabidopsis , Plant Physiology, Volume 162, Issue 2, June 2013, Pages 720–731, https://doi.org/10.1104/pp.113.214940
- Share Icon Share
Abstract
In angiosperms, pollen wall pattern formation is determined by primexine deposition on the microspores. Here, we show that AUXIN RESPONSE FACTOR17 (ARF17) is essential for primexine formation and pollen development in Arabidopsis (Arabidopsis thaliana). The arf17 mutant exhibited a male-sterile phenotype with normal vegetative growth. ARF17 was expressed in microsporocytes and microgametophytes from meiosis to the bicellular microspore stage. Transmission electron microscopy analysis showed that primexine was absent in the arf17 mutant, which leads to pollen wall-patterning defects and pollen degradation. Callose deposition was also significantly reduced in the arf17 mutant, and the expression of CALLOSE SYNTHASE5 (CalS5), the major gene for callose biosynthesis, was approximately 10% that of the wild type. Chromatin immunoprecipitation and electrophoretic mobility shift assays showed that ARF17 can directly bind to the CalS5 promoter. As indicated by the expression of DR5-driven green fluorescent protein, which is an synthetic auxin response reporter, auxin signaling appeared to be specifically impaired in arf17 anthers. Taken together, our results suggest that ARF17 is essential for pollen wall patterning in Arabidopsis by modulating primexine formation at least partially through direct regulation of CalS5 gene expression.
In angiosperms, the pollen wall is the most complex plant cell wall. It consists of the inner wall, the intine, and the outer wall, the exine. The exine is further divided into sexine and nexine layers. The sculptured sexine includes three major parts: baculum, tectum, and tryphine (Heslop-Harrison, 1971; Piffanelli et al., 1998; Ariizumi and Toriyama, 2011; Fig. 1A). Production of a functional pollen wall requires the precise spatial and temporal cooperation of gametophytic and sporophytic tissues and metabolic events (Blackmore et al., 2007). The intine layer is controlled gametophytically, while the exine is regulated sporophytically. The sporophytic tapetum cells provide material for pollen wall formation, while primexine determines pollen wall patterning (Heslop-Harrison, 1968).

Schematic representation of the pollen wall and primexine development. A, The innermost layer adjacent to the plasma membrane is the intine. The bacula (Ba), tectum (Te), and tryphine (T) make up the sexine layer. The nexine is located between the intine and the sexine layers. The exine includes the nexine and sexine layers. B, Primexine (Pr) appears between callose (Cl) and plasma membrane (Pm) at the early tetrad stage (left panel). Subsequently, the plasma membrane becomes undulated (middle panel) and sporopollenin deposits on the peak of the undulated plasma membrane to form bacula and tectum (right panel).
After meiosis, four microspores were encased in callose to form a tetrad. Subsequently, the primexine develops between the callose layer and the microspore membrane (Fig. 1B), and the microspore plasma membrane becomes undulated (Fig. 1B; Fitzgerald and Knox, 1995; Southworth and Jernstedt, 1995). Sporopollenin precursors then accumulate on the peak of the undulated microspore membrane to form the bacula and tectum (Fig. 1B; Fitzgerald and Knox, 1995). After callose degradation, individual microspores are released from the tetrad, and the bacula and tectum continue to grow into exine with further sporopollenin deposition (Fitzgerald and Knox, 1995; Blackmore et al., 2007).
The callose has been reported to affect primexine deposition and pollen wall pattern formation. The peripheral callose layer, secreted by the microsporocyte, acts as the mold for primexine (Waterkeyn and Bienfait, 1970; Heslop-Harrison, 1971). CALLOSE SYNTHASE5 (CalS5) is the major enzyme responsible for the biosynthesis of the callose peripheral of the tetrad (Dong et al., 2005; Nishikawa et al., 2005). Mutation of Cals5 and abnormal CalS5 pre-mRNA splicing resulted in defective peripheral callose deposition and primexine formation (Dong et al., 2005; Nishikawa et al., 2005; Huang et al., 2013). Besides CalS5, four membrane-associated proteins have also been reported to be involved in primexine formation: DEFECTIVE EXINE FORMATION1 (DEX1; Paxson-Sowders et al., 1997, 2001), NO EXINE FORMATION1 (NEF1; Ariizumi et al., 2004), RUPTURED POLLEN GRAIN1 (RPG1; Guan et al., 2008; Sun et al., 2013), and NO PRIMEXINE AND PLASMA MEMBRANE UNDULATION (NPU; Chang et al., 2012). Mutation of DEX1 results in delayed primexine formation (Paxson-Sowders et al., 2001). The primexine in nef1 is coarse compared with the wild type (Ariizumi et al., 2004). The loss-of-function rpg1 shows reduced primexine deposition (Guan et al., 2008; Sun et al., 2013), while the npu mutant does not deposit any primexine (Chang et al., 2012). Recently, it was reported that Arabidopsis (Arabidopsis thaliana) CYCLIN-DEPENDENT KINASE G1 (CDKG1) associates with the spliceosome to regulate the CalS5 pre-mRNA splicing for pollen wall formation (Huang et al., 2013). Clearly, disrupted primexine deposition leads to aberrant pollen wall patterning and ruptured pollen grains in these mutants.
The plant hormone auxin has multiple roles in plant reproductive development (Aloni et al., 2006; Sundberg and Østergaard, 2009). Knocking out the two auxin biosynthesis genes, YUC2 and YUC6, caused an essentially sterile phenotype in Arabidopsis (Cheng et al., 2006). Auxin transport is essential for anther development; defects in auxin flow in anther filaments resulted in abnormal pollen mitosis and pollen development (Feng et al., 2006). Ding et al. (2012) showed that the endoplasmic reticulum-localized auxin transporter PIN8 regulates auxin homeostasis and male gametophyte development in Arabidopsis. Evidence for the localization, biosynthesis, and transport of auxin indicates that auxin regulates anther dehiscence, pollen maturation, and filament elongation during late anther development (Cecchetti et al., 2004, 2008). The role of auxin in pollen wall development has not been reported.
The auxin signaling pathway requires the auxin response factor (ARF) family proteins (Quint and Gray, 2006; Guilfoyle and Hagen, 2007; Mockaitis and Estelle, 2008; Vanneste and Friml, 2009). ARF proteins can either activate or repress the expression of target genes by directly binding to auxin response elements (AuxRE; TGTCTC/GAGACA) in the promoters (Ulmasov et al., 1999; Tiwari et al., 2003). The Arabidopsis ARF family contains 23 members. A subgroup in the ARF family, ARF10, ARF16, and ARF17, are targets of miRNA160 (Okushima et al., 2005b; Wang et al., 2005). Plants expressing miR160-resistant ARF17 exhibited pleiotropic developmental defects, including abnormal stamen structure and reduced fertility (Mallory et al., 2005). This indicates a potential role for ARF17 in plant fertility, although the detailed function remains unknown. In addition, ARF17 was also proposed to negatively regulate adventitious root formation (Sorin et al., 2005; Gutierrez et al., 2009), although an ARF17 knockout mutant was not reported and its phenotype is unknown.
In this work, we isolated and characterized a loss-of-function mutant of ARF17. Results from cytological observations suggest that ARF17 controls callose biosynthesis and primexine deposition. Consistent with this, the ARF17 protein is highly abundant in microsporocytes and tetrads. Furthermore, we demonstrate that the ARF17 protein is able to bind the promoter region of CalS5. Our results suggest that ARF17 regulates pollen wall pattern formation in Arabidopsis.
RESULTS
A Knockout Mutant of ARF17 Is Male Sterile
We screened for male-sterile plants from the pool of transfer DNA (T-DNA)-tagged lines in Arabidopsis ecotype Columbia-0 (Col-0; Qin et al., 2003). One completely sterile mutant without any apparently vegetative defects was identified (Fig. 2, A and B). Thermal asymmetric interlaced (TAIL)-PCR (Liu et al., 1995) and sequencing of the flanking sequence revealed that a T-DNA was inserted in the first exon of the ARF17 gene (Fig. 2C). Genotype analysis showed that the sterile plants from the F2 progeny of selfed F1 were all homozygous (n ≥ 100), while the fertile plants were either heterozygous or without insertion (n ≥ 100). This indicates that the T-DNA insertion in ARF17 was linked to the sterile phenotype. The expression of ARF17 could not be detected in flower buds (Fig. 2D).
![Characterization of the Arabidopsis arf17 mutant. A, A 35-d-old wild-type plant. B, A 35-d-old arf17 mutant plant. C, Structure of the ARF17 (At1g77850) gene. Exons are shown as black boxes. Introns, the promoter, and untranslated regions are shown as gray lines. T-DNA is inserted in the first exon. LP, Left border primer; RP, right border primer. D, RT-PCR analysis of ARF17 in wild-type (WT) and arf17 buds using primer pair LP/RP. Tubulin (TUB) was amplified as an internal control. Similar results were obtained in three independent experiments. E and F, Comparison of flowers from the wild type (E) and arf17 (F). G and H, Alexander’s staining of anthers of the wild type (G) and arf17 (H). Arrows indicate degraded debris. Bars = 2 cm (A and B), 500 μm, (E and F), and 200 μm (G and H). [See online article for color version of this figure.]](https://oup.silverchair-cdn.com/oup/backfile/Content_public/Journal/plphys/162/2/10.1104_pp.113.214940/2/m_plphys_v162_2_720_f2.jpeg?Expires=1747912250&Signature=o7i72TLxP~JffWvwqVNDuyw1MugayFB1ESken29X-RFpYv36FSogVndja9t9d6IZnObfihgrE8CjPEwf~lN5UuZwA5OEtbFSubO-3Sg1h-5TPy5IxiV3rDTl8Iqhmlqd4nz44LC~gi3EqwqV2Hveu2aCkWlgoSAaqM5qgz9vEnu3nP-0R-R7ZFQtXqhNhrSR-1PJq2CXjOn2a3XpaAbBHbg6mZ-leEQAae9gyWLwYUt7sr9J3O0qnibNmxA-lxMR7clIVDp3BZGVPLrDA8fo7bQf0stk1lUq-QEBXN6HpsP-Ke89WWm05UveSABgJXItJWOUjhvV3IGR8I7g1SdhQw__&Key-Pair-Id=APKAIE5G5CRDK6RD3PGA)
Characterization of the Arabidopsis arf17 mutant. A, A 35-d-old wild-type plant. B, A 35-d-old arf17 mutant plant. C, Structure of the ARF17 (At1g77850) gene. Exons are shown as black boxes. Introns, the promoter, and untranslated regions are shown as gray lines. T-DNA is inserted in the first exon. LP, Left border primer; RP, right border primer. D, RT-PCR analysis of ARF17 in wild-type (WT) and arf17 buds using primer pair LP/RP. Tubulin (TUB) was amplified as an internal control. Similar results were obtained in three independent experiments. E and F, Comparison of flowers from the wild type (E) and arf17 (F). G and H, Alexander’s staining of anthers of the wild type (G) and arf17 (H). Arrows indicate degraded debris. Bars = 2 cm (A and B), 500 μm, (E and F), and 200 μm (G and H). [See online article for color version of this figure.]
Genetic complementation was performed to confirm whether knocking out ARF17 is responsible for the sterile phenotype. A genomic fragment of ARF17 including 1,615 bp upstream of the translation start site, the intact genomic coding region, and 567 bp downstream of the stop codon was cloned and introduced into plants heterozygous for the ARF17 mutation (Supplemental Fig. S1A). We obtained a total of 20 independent T1 transgenic lines. Genotyping analysis showed that four of them were homozygous for the ARF17 mutation (Supplemental Fig. S1C). Three of these plants were fully fertile (Supplemental Fig. S1D), indicating that ARF17 was able to complement the sterile phenotype. The failed complementation of another T1 plant could have been due to a position effect of the transgene (Matzke and Matzke, 1998). Collectively, these results demonstrate that knockout of ARF17 is responsible for the sterile phenotype of this mutant.
To distinguish whether male or female defects cause the sterility in arf17, we first pollinated the arf17 stigma with wild-type pollen grains. Normal siliques could be obtained, indicating that the female fertility in arf17 was not affected (data not shown). We then observed the open flowers from the wild type and the arf17 mutant using a dissecting microscope. Multiple pollen grains could be found on the anther surface and the stigma of the wild type (Fig. 2E). In contrast, no pollen grains were observed in the arf17 flower (Fig. 2F). Further Alexander staining of pollen viability (Alexander, 1969) showed that the wild-type anthers contained purple-staining viable pollen grains (Fig. 2G), while only degraded pollen remnants were observed in the arf17 anther (Fig. 2H). These data indicate that knocking out ARF17 leads to aborted pollen development and male sterility.
Expression Patterns of ARF17
ARF17 is widely expressed in Arabidopsis tissues, including the roots, leaves, stems, siliques, seedlings, and buds (Mallory et al., 2005). To analyze the spatiotemporal expression of ARF17 during anther development, we performed an RNA in situ hybridization assay with an ARF17-specific antisense probe. Anther development can be divided into 14 stages (Sanders et al., 1999). At stage 5, no obvious hybridization signal was detected (Fig. 3A). Apparent hybridization signals were observed in microsporocytes at stage 6 (Fig. 3B). Expression of ARF17 was reduced in tetrads at stage 7 (Fig. 3C) and remained detectable in the microspores until stage 9 (Fig. 3, D and E). In mature pollen, no ARF17-specific hybridization signal was detected (Fig. 3, F and G). As a control, the ARF17 sense-strand probe did not yield any hybridization signal (Fig. 3H).
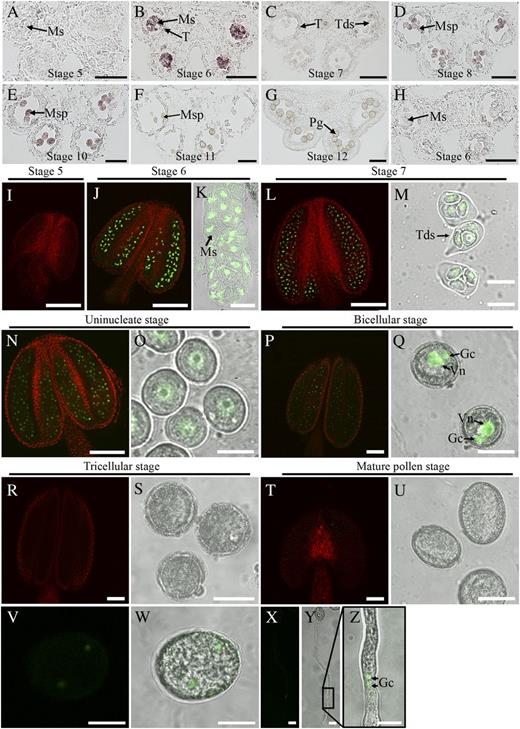
Expression pattern of ARF17. A to H, In situ hybridization analysis of ARF17 during anther development. Wild-type anthers from stages 5 (A), 6 (B), 7 (C), 8 (D), 9 (E), 10 (F), and 11 (G) hybridized with the antisense probe are shown. H, Stage 6 anther hybridized with the sense probe. I to Y, Fluorescence confocal images of the ARF17-GFP fusion protein. I, Stage 5 anther. J and K, Stage 6 anther and microsporocyte. L and M, Stage 7 anther and tetrads. N and O, Stage 8 anther and uninucleate microspore. P and Q, Bicellular stage anther and microspore. R and S, Tricellular stage anther and pollen grains. T and U, Dehiscent anther and mature pollen. V and W, Germinated pollen grain. X to Z, Germinated pollen tubes. Z shows an enlarged image of the boxed area in Y. Gc, Germ cell; Ms, microsporocyte; Msp, microspore; Pg, pollen grain; T, Tapetum; Tds, tetrads; Vn, vegetative nuclei. Bars = 50 μm (A–J, L, N, P, R, and T), 20 μm (K, M, O, Q, S, and U), and 10 μm (V–Z).
We further investigated the distribution of the ARF17 protein. The genomic fragment of ARF17 was fused with the gene for GFP (Supplemental Fig. S1B), and this construct was introduced into the arf17 mutant (Supplemental Fig. S1C). The ARF17-GFP fusion protein was shown to be functional because it was able to rescue the male-sterile phenotype in the arf17 mutant (Supplemental Fig. S1E). Confocal microscopy of GFP allows detection of the respective ARF17-GFP fusion proteins. At stage 5, no GFP fluorescence was observed (Fig. 3I). At stage 6, significant GFP signals were observed in microsporocytes (Fig. 3, J and K). At stage 7, ARF17-GFP fluorescence could be detected within microspores in tetrads (Fig. 3, L and M). GFP expression was also found in the microspores at the uninucleate pollen stage (Fig. 3, N and O). In bicellular pollen, GFP signals were present in both the germ cell and the vegetative nuclei (Fig. 3, P and Q). No apparent GFP signal was detected at either the tricellular or mature pollen stage (Fig. 3, R–U), which is consistent with a previous male gametophyte transcriptome (Honys and Twell, 2003). When ARF17-GFP pollen grains were germinated in vitro, GFP could also be detected in the imbibed pollen grain (Fig. 3, V and W) and pollen tube (Fig. 3, X–Z); however, the expression level was lower than that observed from stage 6 to the bicellular microspore stage.
Primexine Is Absent in the arf17 Mutant
In order to understand the defects in arf17 in detail, we analyzed cross sections prepared from wild-type (Col-0) and mutant inflorescences. Up to stage 6, no detectable differences could be observed between the wild type and the arf17 mutant (Fig. 4, A, B, E, and F). 4,6-Diamidino-2-phenylindole (DAPI) staining showed that meiosis is normal in arf17 (Supplemental Fig. S2). At stage 7, meiosis is completed and tetrads have formed in the wild type (Fig. 4C). In contrast, the shape of arf17 tetrads appeared to be distorted (Fig. 4G). At stage 8, individual microspores could be observed in both wild-type and arf17 anthers, indicating the normal release of free microspores (Fig. 4, D and H). From stage 9 to 11, wild-type microspores developed an obvious exine layer (Fig. 4, I and J). In arf17, however, microspores failed to form an exine layer and became vacuolated and degraded (Fig. 4, L and M). Eventually, the pollen wall developed successfully and mature pollen grains were formed in the wild type (Fig. 4K), whereas all arf17 microspores degenerated completely, leaving only aborted pollen debris (Fig. 4N).
![Comparison of transverse anther sections. Anther cross sections from the wild type (A–D and I–K) and arf17 (E–H and L–N) were stained by toluidine blue. A and E, Anthers at stage 5 from the wild type (A) and arf17 (E). B and F, Anthers at stage 6 from the wild type (B) and arf17 (B). C and G, Stage 7 anthers from the wild type (C) and arf17 (G). D and H, Stage 8 anthers from the wild type (D) and arf17 (H). I and L, Stage 9 anthers from the wild type (I) and arf17 (L). J and M, Stage 10 anthers from the wild type (J) and arf17 (M). K and N, Stage 12 anthers from the wild type and arf17 (N). Dmsp, Degraded microspore; Dpg, degraded pollen grain; E, epidermis; En, endothecium; Ml, middle layer; Ms, microsporocyte; Msp, microspore; Pg, pollen grain; Sp, sporopollenin; T, Tapetum; Tds, tetrads. Bars = 20 μm. [See online article for color version of this figure.]](https://oup.silverchair-cdn.com/oup/backfile/Content_public/Journal/plphys/162/2/10.1104_pp.113.214940/2/m_plphys_v162_2_720_f4.jpeg?Expires=1747912250&Signature=dwNKF1~BeEamLbFcFF34RgDh61muIy~0rGNkc4mcVgC3NgOjcTj55wG0oRe15co43~PRfNrXvLEDEm4icnLaprLmw39k4ICWPHmgXFZHjR0XcDv6Z3FHaFOQAhifo-BbqVHbbix~TLNVxQbaGayNvql0zztckksWeisuG2J0sKZw2-zrn95wIPeeU4WfFZy3GkHpcm7Kz11eeV7CdQ1E9p8-QMInyxgtjti7GrlVyp4wH6bVJXFARR9ddglJZvzySNTzTxsec6YXGKbm6DfINBf94b6bvTkIb-ieIjZ-ajxXPSiUKpkbKTh8IRXlB2VYXW9TipBPSU~PSVr~ZyM-Rw__&Key-Pair-Id=APKAIE5G5CRDK6RD3PGA)
Comparison of transverse anther sections. Anther cross sections from the wild type (A–D and I–K) and arf17 (E–H and L–N) were stained by toluidine blue. A and E, Anthers at stage 5 from the wild type (A) and arf17 (E). B and F, Anthers at stage 6 from the wild type (B) and arf17 (B). C and G, Stage 7 anthers from the wild type (C) and arf17 (G). D and H, Stage 8 anthers from the wild type (D) and arf17 (H). I and L, Stage 9 anthers from the wild type (I) and arf17 (L). J and M, Stage 10 anthers from the wild type (J) and arf17 (M). K and N, Stage 12 anthers from the wild type and arf17 (N). Dmsp, Degraded microspore; Dpg, degraded pollen grain; E, epidermis; En, endothecium; Ml, middle layer; Ms, microsporocyte; Msp, microspore; Pg, pollen grain; Sp, sporopollenin; T, Tapetum; Tds, tetrads. Bars = 20 μm. [See online article for color version of this figure.]
To further investigate the detailed abnormalities in arf17 exine development, transmission electron microscopy (TEM) observation was employed. In the wild-type locules at stage 6, callose was deposited around microsporocytes with medium electron-dense staining (Fig. 5A). The callose in arf17 was much reduced compared with the wild type (Fig. 5B). At stage 7, the wild-type tetrad developed primexine between microspore membrane and the callose layer; microspore membrane became undulated (Fig. 5C). In contrast, the callose in the arf17 mutant seemed thinner; the primexine and membrane undulation were not observed (Fig. 5D). At the uninucleate and the bicellular microspore stages, sporopollenin continued to accumulate on the surface of the microspore to form the bacula, tectum, and exine in the wild type (Fig. 5, E and G). However, in arf17, the sporopollenin randomly accumulated around the microspore surface, and no exine could be observed for those shriveled pollen grains (Fig. 5, F and H). TEM observations revealed that arf17 pollen had an absence of primexine, leading to subsequent aborted exine pattern and microspore degradation.
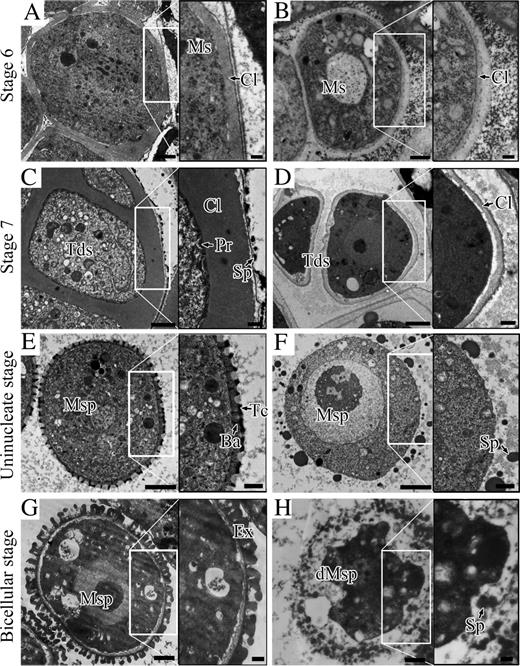
Ultrastructure of pollen wall development. Cross sections from the wild type (A, C, E, and G) and arf17 (B, D, F, and H) are shown. The black boxed image on the right of each panel was enlarged from the corresponding white boxed region. A and B, Microsporocyte at stage 6 from the wild type (A) and arf17 (B). C and D, Tetrad of the wild type (C) and arf17 (D). The primexine is absent in arf17. E and F, Uninucleate microspore stage. Bacula and tectum are visible in the wild-type anther (E) but not in the arf17 mutant (F). Sporopollenin is randomly deposited around the arf17 microspore. G and H, Bicellular stage. The exine layer is established in the wild type (G). Microspore exine is absent and the microspore is degenerate in the arf17 mutant (H). Ba, Bacula; Cl, callose; dMsp, degenerate microspore; Ex, exine; Ms, microsporocyte; Msp, microspore; Pr, primexine; Sp, sporopollenin; Tc, tectum; Tds, tetrads. Bars = 2 μm.
Callose Deposition around the Tetrad Is Significantly Reduced in arf17
The above observations indicated that callose deposition was decreased in the arf17 mutant. To confirm this, we stained tetrads from both arf17 and wild-type anthers with toluidine blue. Under light microscopy, both wild-type and ARF17-GFP complemented plants showed an apparent callose layer in tetrads (Fig. 6, A and C; n > 150). By contrast, the callose layer of arf17 tetrads was ambiguous (n = 295; Fig. 6B). We then analyzed callose fluorescence by aniline blue staining. Both aniline blue staining and fluorescence analysis showed that the fluorescence of the callose in arf17 was obviously weaker than that in wild-type and ARF17-GFP complemented plants (Fig. 6, D–I). These results confirmed that arf17 tetrads had a reduced callose deposition.
![Analysis of callose deposition and CalS5 expression. A to C, Toluidine blue staining of tetrads from wild-type (A), arf17 (B), and ARF17-GFP complemented (Comp; C) plants. D to F, Aniline blue staining of the callose from wild-type (D), arf17 (E), and Comp (F) plants. G, Quantitative analysis of fluorescence intensity from wild-type (WT), arf17, and Comp plants. Asterisks indicate statistically significant differences compared with the wild type and Comp by Student’s t test (P < 0.05; n ≥ 100). H and I, Aniline blue staining of anther cross sections from the wild type (H) and arf17 (I) at stage 7. J, Real-time PCR analysis of CalS5 expression in wild-type and arf17 buds. The level of CalS5 was normalized to Tubulin and compared with the wild-type control. Error bars indicate sd and were calculated from three biological replicates. K to R, In situ hybridization analysis of CalS5 expression in wild-type (K–N) and arf17 (O–R) anthers. K and O, Anthers at stage 5 from the wild type (K) and arf17 (O). L and P, Anthers at stage 6 from the wild type (L) and arf17 (P). M and Q, Anthers at stage 7 from the wild type (M) and arf17 (Q). N and R, Anthers at stage 6 from the wild type (N) and arf17 (R) hybridized with the sense probe. Anther developmental stages are numbered according to Sanders et al. (1999). Ms, Microsporocyte; T, Tapetum; Tds, tetrads. Bars = 20 μm (A–F) and 50 μm (H, I, and K–R). [See online article for color version of this figure.]](https://oup.silverchair-cdn.com/oup/backfile/Content_public/Journal/plphys/162/2/10.1104_pp.113.214940/2/m_plphys_v162_2_720_f6.jpeg?Expires=1747912250&Signature=MP98iwbNSThqg~Ss1KuUWrSRc0kqS0Wqg-riYRIBv59vLQNy5X5kO24DzPeueyEn26-b1p5VfwQmdCpGwda0q1h2fmI5MOQwvZ3xQ~7GJHN~3FebUDsin08ZnFJ1QDFtvIjd~H8mu4x3GlhLX7-weybnvQDIOiPqmoSL2MvrhJFq3kcjn6ErvNSxKnuA2tD7Dke1~5P~F4PItUaMrm7LQYj5aBu8OhNkBHft88Hme6q24YBAsj1wapuw7zmUwMNHdy3Aeqfpgovyq~cr2CRNauBUnGsFBvDcukQpd4LqNwoJkejYMi3PhfeAQssC2-~wadTPN7XaWVAdHgG9wLNGvA__&Key-Pair-Id=APKAIE5G5CRDK6RD3PGA)
Analysis of callose deposition and CalS5 expression. A to C, Toluidine blue staining of tetrads from wild-type (A), arf17 (B), and ARF17-GFP complemented (Comp; C) plants. D to F, Aniline blue staining of the callose from wild-type (D), arf17 (E), and Comp (F) plants. G, Quantitative analysis of fluorescence intensity from wild-type (WT), arf17, and Comp plants. Asterisks indicate statistically significant differences compared with the wild type and Comp by Student’s t test (P < 0.05; n ≥ 100). H and I, Aniline blue staining of anther cross sections from the wild type (H) and arf17 (I) at stage 7. J, Real-time PCR analysis of CalS5 expression in wild-type and arf17 buds. The level of CalS5 was normalized to Tubulin and compared with the wild-type control. Error bars indicate sd and were calculated from three biological replicates. K to R, In situ hybridization analysis of CalS5 expression in wild-type (K–N) and arf17 (O–R) anthers. K and O, Anthers at stage 5 from the wild type (K) and arf17 (O). L and P, Anthers at stage 6 from the wild type (L) and arf17 (P). M and Q, Anthers at stage 7 from the wild type (M) and arf17 (Q). N and R, Anthers at stage 6 from the wild type (N) and arf17 (R) hybridized with the sense probe. Anther developmental stages are numbered according to Sanders et al. (1999). Ms, Microsporocyte; T, Tapetum; Tds, tetrads. Bars = 20 μm (A–F) and 50 μm (H, I, and K–R). [See online article for color version of this figure.]
CalS5 is the major enzyme responsible for peripheral callose synthesis in the tetrad in Arabidopsis (Dong et al., 2005). We analyzed the expression of CalS5 in arf17. Real-time PCR revealed that the expression of CalS5 was dramatically decreased in the arf17 mutant compared with the wild type (Fig. 6J). To further verify these results at the cellular level, we performed RNA in situ hybridization using a CalS5-specific probe. The CalS5 signal reached its peak in microsporocytes and tapetum cells at stage 6 (Fig. 6, K–M), consistent with a previous report (Nishikawa et al., 2005). In the arf17 mutant, CalS5 transcript abundance was much less than in the wild type (Fig. 6, O–Q), confirming reduced CalS5 expression.
We then compared the expression of other primexine-related genes, including CDKG1, DEX1, NEF1, RPG1, and NPU, in arf17 and wild-type inflorescences. Both reverse transcription (RT)-PCR and real-time PCR showed that CDKG1, DEX1, NEF1, and NPU expression were not altered in arf17 (Supplemental Fig. S3, A and B), whereas RPG1 expression in arf17 was reduced to 30% of that in wild-type plants (Supplemental Fig. S3, A and B).
ARF17 Directly Regulates CalS5 Expression
ARF17 is an ARF transcription factor and would be expected to regulate gene expression by binding to the AuxRE of target gene promoters. An AuxRE (GAGACA) was found between −863 and −858 in the CalS5 promoter (Fig. 7A). To corroborate the interaction of the ARF17 protein with the CalS5 promoter in vivo, we performed a chromatin immunoprecipitation (ChIP) assay using buds from a transgenic Arabidopsis line that expressed the functional GFP-tagged ARF17 (Fig. 3). Real-time PCR results indicated that one DNA fragment, pCalS5-2, containing the predicted ARF protein-binding site (GAGACA) was enriched when the GFP antibodies were used (Fig. 7B). However, when three other fragments of the CalS5 promoter were amplified, no obvious enrichment was observed (Fig. 7B).
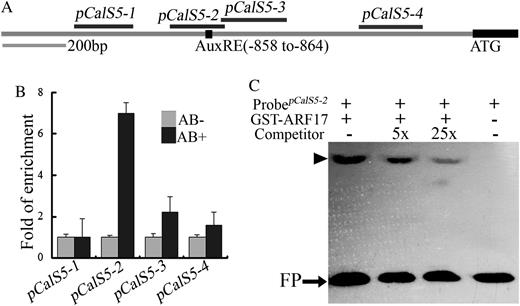
CalS5 is a direct target of ARF17. A, Potential ARF-binding sites in the 1.5-kb CalS5 promoter. The black box indicates the position of the canonical AuxRE sequence (GAGACA). The black lines at top indicate fragments amplified in Chip-PCR assays. B, Real-time PCR of promoter fragments in ChIP using ARF17-GFP flower buds. Enrichment was only observed when the fragment containing the AuxRE was amplified when affinity-purified GFP antibody was used. C, EMSA for ARF17 binding to the CalS5 gene promoter. GST-ARF17 protein was mixed with a biotin-labeled 64-bp probe containing the AuxRE and 5- or 25-fold unlabeled probe as competitor. The arrowhead indicates a shift band. FP, Free probe.
In order to confirm the physical interaction between the ARF17 protein and the CalS5 promoter region, we performed an electrophoretic mobility shift assay (EMSA) using recombinant ARF17 proteins fused to glutathione S-transferase (GST-ARF17) and a 64-bp CalS5 promoter fragment containing an AuxRE motif. The EMSA result showed that the recombinant ARF17 protein is able to bind this DNA fragment (Fig. 7C). The binding specificity was further confirmed by using unlabeled promoter fragment as a competitor. When the unlabeled DNA fragment was added, the excess CalS5 competitor probe reduced the signal in a concentration-dependent manner (Fig. 7C). These results indicate that ARF17 directly binds to the CalS5 promoter to regulate its expression.
The arf17 Mutation Affects Pollen Tube Growth
In the F2 population, only about 12% (62 of 493) of the progeny displayed the male-sterile phenotype. This ratio was stable over five consecutive generations. The segregation ratio deviated significantly from the expected 25% (1:3; Table I), suggesting that the gametophyte function may also be affected in arf17/ARF17 (arf17/+) plants. We pollinated arf17/+ stigmas with wild-type pollen. The segregation ratio of the T-DNA in the F1 progeny was approximately 1:1 (111:114; Table I), indicating that the transmission of the T-DNA through the female gametophyte is not affected and that the arf17 ovule is fertile. When wild-type stigmas were pollinated with arf17/+ pollen, the T-DNA ratio of the F1 progeny was 24.9% (n = 289), instead of the expected 50% (1:1; Table I), indicating that male gametophyte transmission is reduced in the arf17/+ mutant.
Segregation analysis of the arf17 mutant
FTE, Female transmission efficiency; MTE, male transmission efficiency; ND, not detected.
Parental Genotype (Male × Female) . | Phenotype of Progeny . | Genotype of Progeny . | Transmission Efficiency . | ||
---|---|---|---|---|---|
Fertile . | Sterile . | Bar(+) . | Bar(−) . | ||
arf17/+ × arf17/+ | 426 | 69 | ND | ND | ND |
ARF17/+ × arf17/+ | 225 | 0 | 111 | 114 | 49.3% (FTE) |
arf17/+ × ARF17/+ | 283 | 0 | 72 | 217 | 24.9% (MTE) |
Parental Genotype (Male × Female) . | Phenotype of Progeny . | Genotype of Progeny . | Transmission Efficiency . | ||
---|---|---|---|---|---|
Fertile . | Sterile . | Bar(+) . | Bar(−) . | ||
arf17/+ × arf17/+ | 426 | 69 | ND | ND | ND |
ARF17/+ × arf17/+ | 225 | 0 | 111 | 114 | 49.3% (FTE) |
arf17/+ × ARF17/+ | 283 | 0 | 72 | 217 | 24.9% (MTE) |
FTE, Female transmission efficiency; MTE, male transmission efficiency; ND, not detected.
Parental Genotype (Male × Female) . | Phenotype of Progeny . | Genotype of Progeny . | Transmission Efficiency . | ||
---|---|---|---|---|---|
Fertile . | Sterile . | Bar(+) . | Bar(−) . | ||
arf17/+ × arf17/+ | 426 | 69 | ND | ND | ND |
ARF17/+ × arf17/+ | 225 | 0 | 111 | 114 | 49.3% (FTE) |
arf17/+ × ARF17/+ | 283 | 0 | 72 | 217 | 24.9% (MTE) |
Parental Genotype (Male × Female) . | Phenotype of Progeny . | Genotype of Progeny . | Transmission Efficiency . | ||
---|---|---|---|---|---|
Fertile . | Sterile . | Bar(+) . | Bar(−) . | ||
arf17/+ × arf17/+ | 426 | 69 | ND | ND | ND |
ARF17/+ × arf17/+ | 225 | 0 | 111 | 114 | 49.3% (FTE) |
arf17/+ × ARF17/+ | 283 | 0 | 72 | 217 | 24.9% (MTE) |
To determine whether the reduced transmission was derived from impaired pollen development, pollen germination, or pollen tube growth, homozygous arf17 plants were pollinated with qrt1 pollen (Preuss et al., 1994) to generate arf17/+qrt1/qrt1 plants. In the qrt1 plant, the four microspores remain associated as tetrads (Preuss et al., 1994; Francis et al., 2006). DAPI and Alexander staining showed that arf17/+ pollen grains developed normally (n = 300; Supplemental Fig. S4). We next carried out an in vitro pollen germination assay to test whether the pollen tube performance of arf17/+ was affected. After 6 h, 78% (n = 760) of pollen grains from arf17/+ plants germinated (Fig. 8, A and C), in contrast to 82% (n = 560) of wild-type pollen grains. This indicated that pollen grain germination in arf17/+ may not be affected (P > 0.05). However, the average pollen tube length from arf17/+ pollen grains was dramatically reduced, and more short pollen tubes were observed compared with the wild type (Fig. 8, A, B, and D). Statistical analysis showed that 36% of arf17/+ pollen tubes were in the range of 10 to 200 μm, whereas only 9% of wild-type pollen tubes were in this range; 19% of arf17/+ pollen tubes were more than 400 μm long, while 46% of wild-type pollen tubes were in this range (Fig. 8E). This indicates that the arf17 mutation affected pollen tube growth.
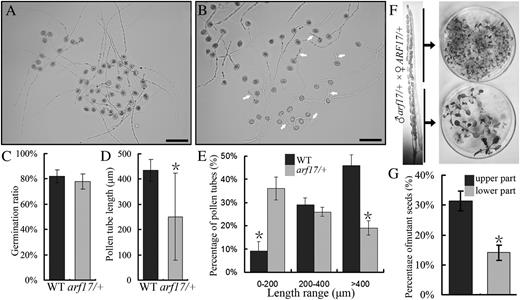
Analysis of pollen germination and pollen tube growth. A, Pollen grains from the wild type germinated in vitro. B, Pollen grains from the arf17/+ heterozygote germinated in vitro. White arrows indicate short pollen tubes. Bars = 100 μm. C, Percentage germination of pollen grains from the wild type (WT) and arf17/+. D, Average lengths of pollen tubes from the wild type and arf17/+. E, Length distribution of wild-type and arf17/+ pollen tubes after germination for 6 h. Means of at least 500 pollen grains from three independent experiments are shown. F, Basta resistance analysis of seeds from crosses between arf17/+ pollen and wild-type pistils. G, Qualitative analysis of Basta resistance of seeds from crosses between arf17/+ pollen and wild-type pistils. Asterisks indicate statistically significant differences by Student’s t test (P < 0.05). Similar results were obtained from at least three independent experiments.
The pollen tube growth defect was further validated in vivo. We pollinated wild-type stigmas in emasculated flowers with arf17/+ pollen grains and examined the distribution of wild-type and mutant seeds in the upper and lower parts of crossed siliques using a Basta herbicide screen. The ratio of mutant seeds from the upper part was obviously higher than that from the lower part (Fig. 8F). Seeds from the upper part of the siliques were 31.3% mutant (n = 147), while seeds from lower part were only 14.1% mutant (n = 135; P < 0.05; Fig. 8G), indicating that wild-type and heterozygous arf17/+ pollen tubes are not evenly distributed within the ovary. Collectively, the in vitro and in vivo results showed that arf17 pollen tubes grow slower than wild-type tubes.
Expression of an Auxin Reporter Gene Is Impaired in arf17 Anthers
The ARF family proteins are involved in auxin signaling and can directly regulate the transcription of auxin response genes. To investigate the role of ARF17 in the auxin response, we introduced the synthetic auxin response promoter DR5 (Ulmasov et al., 1997), driving the expression of the GFP gene (DR5::GFP), into the arf17 mutant by crossing. In 5-week-old wild-type plants, GFP fluorescence could be detected in the root tip (Fig. 9A), leaf tips (Fig. 9C), and anthers at later stages when mature pollen is formed inside (Fig. 9E). In arf17, GFP signals could also be observed in the root tip and leaf tips (Fig. 9, B and D; n = 24). However, the expression of DR5::GFP was barely detected in arf17 late-stage anthers (Fig. 9F; n = 24). Since ARF17 functions at the tetrad stage, we chose to observe the DR5::GFP signal at this stage. In the wild type, apparent GFP signals could be observed at the anther apex and the joint between the anther and filament (Fig. 9G; n = 30). However, GFP signal was not observed in tetrads, which is consistent with a previous report (Cecchetti et al., 2008). In arf17, a similar pattern of GFP expression was detected to that in the wild type (Fig. 9H; n = 30).
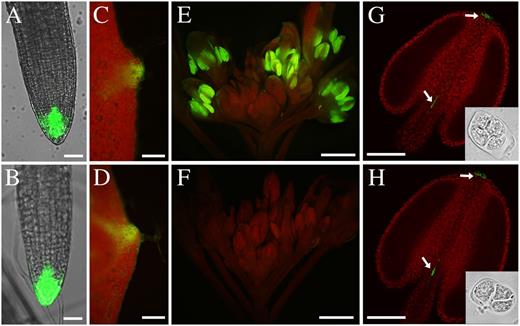
Expression of the auxin response marker DR5::GFP. DR5::GFP expression was observed in root tips in the wild type (A) and the arf17 mutant (B), and GFP fluorescence was observed in leaf tips of the wild type (C) and arf17 (D). E, Wild-type anther showing strong GFP fluorescence. F, GFP fluorescence was severely reduced in arf17 mutant anthers. G and H, Anthers at the tetrad stage (indicated by the insets) from the wild type (G) and arf17 (H) showing similar GFP signals at the tips of the anther and filament (white arrows). Bars = 100 μm (A and B), 500 μm (C–F), and 20 μm (G and H).
DISCUSSION
In this work, we showed that in the arf17 mutant, primexine deposition within tetrads is completely absent. Sporopollenin seems to be synthesized in arf17 (Fig. 5). Exine and pollen wall patterning fail to form; pollen grain degradation and male infertility were observed at later stages (Fig. 4). Because primexine has a central role in exine formation and pollen wall patterning, it was suggested that the male-sterile phenotype of the arf17 mutant is caused by absent primexine. ARF17 is expressed in microsporocytes and tetrads, supporting its role for primexine formation. Currently, several genes have been reported to affect primexine formation and pollen wall patterning. In cals5 (Dong et al., 2005; Nishikawa et al., 2005), dex1 (Paxson-Sowders et al., 2001), nef1 (Ariizumi et al., 2004), and rpg1 (Guan et al., 2008), primexine is reduced, while in npu, primexine is absent (Chang et al., 2012). The CalS5 gene is a callose synthase, and RPG1 functions as a sugar transporter (Dong et al., 2005; Chen et al., 2010). DEX1, NEF1, and NPU are membrane-associated proteins; however, their biochemical roles remain undiscovered. ARF17 belongs to the ARF family of transcription factors, which are considered to be a component of the auxin signaling pathway. Therefore, our results provide a new mechanism that determines primexine formation as well as subsequent pollen wall formation.
ARF proteins can regulate the expression of genes with an AuxRE in their promoter (Ulmasov et al., 1999). The CalS5 promoter contains one AuxRE (−863 to −858). Both ChIP and EMSA showed that ARF17 can directly bind to the promoter region of CalS5. The expression of ARF17 and CalS5 in microsporocytes and tetrads supports the binding of ARF17 to the CalS5 promoter. The expression of CalS5 in the arf17 mutant was approximately 10% of the level in the wild type (Fig. 6). In arf17, the peripheral callose layer of the tetrad was detected, although the amount was significantly reduced compared with the wild type (Fig. 6). Therefore, these results show that ARF17 directly regulates CalS5 expression in callose synthesis. Recently, Huang et al. (2013) showed that CDKG1 can interact with the splicing factor to regulate CalS5 splicing and pollen wall formation. In addition, CalS5 expression is also decreased in rpg1 and npu mutants (Chang et al., 2012; Sun et al., 2013), indicating that CalS5 expression is regulated at multiple levels.
The cals5 knockout mutants can produce a reduced amount of primexine and are able to generate several seeds (Dong et al., 2005; Nishikawa et al., 2005), whereas arf17 is completely sterile and shows no primexine deposition (Fig. 4). This suggests that there are additional genes that can be regulated by ARF17 in primexine formation. RPG1 is down-regulated in arf17 (Fig. 6). However, no AuxRE is present in the RPG1 promoter; it is likely that ARF17 regulates RPG1 expression through an unknown intermediate transcription factor. Therefore, reduced expression of both CalS5 and RPG1 should partially contribute to the primexine defect in arf17. The expression of DEX1, NEF1, and NPU was not altered in the arf17 mutant (Fig. 6), indicating that ARF17 does not regulate their expression at the transcription level. We propose that there are some novel genes required for primexine formation that can also be regulated by ARF17. Further identification of direct targets of ARF17 could help us to understand the regulatory role of ARF17 and the underlying mechanism controlling primexine formation and pollen wall development.
The expression of ARF17 in germinated pollen grains and pollen tubes, and the reduced male gametophyte transmission efficiency growth in heterozygous plants, indicated that ARF17 plays a role in pollen tube growth. It was reported that IAA could stimulate pollen tube growth in Torenia fournieri (Wu et al., 2008). ARF17 may act as an important component in auxin signaling to regulate pollen tube growth in Arabidopsis. ARF17 is essential for pollen wall formation (Fig. 5). However, the genetic analysis showed that arf17 pollen can grow and transmit, although the pollen tube growth is slower than the wild type (Table I; Fig. 8). This suggests that other ARFs may also be involved in pollen tube growth.
Evidence for the localization, biosynthesis, and transport of auxin indicates that auxin regulates dehiscence, pollen maturation, and filament elongation during late anther development (Cecchetti et al., 2008). The expression of an auxin-sensitive reporter marker gene (DR5::GUS/GFP) in anthers also supports the involvement of auxin in these biological processes (Aloni et al., 2006; Feng et al., 2006; Cecchetti et al., 2008). In anther, high-level expression of DR5::GUS/GFP could be detected after the release of the microspores from the tetrads, at which time ARF17 was also expressed. The reduced expression of DR5::GFP in arf17 anthers indicated that ARF17 is important for anther auxin signaling and the expression of auxin response genes. DR5::GFP expression in wild-type tetrads was not observed (Fig. 9), which is consistent with a previous report (Cecchetti et al., 2008). However, ARF17 is present at this stage. The failure to detect DR5::GFP in the tetrad could be due to its low level of expression or a lack of necessary cofactors at this stage (Nakamura et al., 2003).
DR5::GFP expression can be detected in the arf17 anther tip at the tetrad stage, indicating that many other ARFs could also function at this stage. ARF6 and ARF8 are expressed in anther filaments, the style, transmitting tract, and funiculus, and they regulate multiple aspects of flower development (Nagpal et al., 2005; Goetz et al., 2006; Wu et al., 2006). It seems that ARF6 and ARF8 are dispensable for pollen development, since treatment with exogenous jasmonic acid could cause the release of viable pollen grains in the arf6arf8 double mutant (Nagpal et al., 2005). ARF2 is expressed in the sepals and stamen. The predominant morphological defect of arf2 mutant flowers is the significantly elongated gynoecium and sepals, which result in tightly closed buds (Okushima et al., 2005a). Compared with these studied ARF proteins, the ARF17 protein is likely to have a unique function in the ARF family in controlling pollen wall pattern formation. Our results support that ARF family genes are expressed in different plant tissues/organs and exhibit specific or redundant functions in cellular processes during plant development (Wang et al., 2007).
MATERIALS AND METHODS
Plant Materials and Growth Conditions
Arabidopsis (Arabidopsis thaliana) wild-type and mutant plants in this study were in the Col-0 ecotype background. The arf17 mutant was isolated from the pSKI15 activation-tagging T-DNA mutant pools (Qin et al., 2003). Seeds were sown on vermiculite and allowed to imbibe for 3 d at 4°C. Plants were grown under long-day conditions (16 h of light/8 h of dark) in a growth room at approximately 22°C.
Phenotype Characterization
Plants were photographed with a Nikon D700 digital camera. Alexander staining was performed as described (Alexander, 1969). For cross sections, flower buds were fixed overnight in 50% (v/v) ethanol, 5.0% (v/v) acetic acid, and 3.7% (v/v) formaldehyde, dehydrated in a graded ethanol series (50% [×2], 60%, 70%, 80%, 90%, 95%, and 100% [×2]), and embedded in resin with a low-viscosity kit (PELCO). Transverse sections of 1.5 μm thickness were stained with 0.5% toluidine blue and observed with an Olympus BX51 microscope (http://www.olympus-global.com). For TEM examination, inflorescences from the wild type and arf17 were fixed and embedded as described (Zhang et al., 2007). The images were taken by a Hitachi H-600 transmission electron microscope (http://www.hitachi.com). For the pollen in vitro germination assay, mature pollen grains were spread onto Arabidopsis pollen medium consisting of 18% (m/v) Suc, 0.01% (m/v) boric acid, 1 mm CaCl2, 1 mm Ca(NO3)2, 1 mm MgSO4, and 0.5% (m/v) agar (Li et al., 1999) in a humid chamber at approximately 22°C. Single images were obtained with the Olympus BX51 microscope.
Fluorescence Microscopy
DAPI staining was preformed as described (Jiang et al., 2009). For callose staining, unopened buds were fixed for 3 h with Carnoy’s fluid (3:1 ethanol and acetic acid) at 4°C. Anthers at the tetrad stage were squeezed onto the slide and stained with toluidine blue (100 μg L−1 in 10 mm KPO4 buffer, pH 7.2) or aniline blue solution (0.1 g L−1 in 50 mm KPO4 buffer, pH 7.5). After covering with cover glasses, the slides stained by toluidine blue were observed under transmitted light. Slides stained by aniline blue were observed under UV illumination using an Olympus BX51 fluorescence microscope. ARF17-GFP and DR5::GFP in the root tip and anther tip were observed using a Carl Zeiss confocal laser scanning microscope (LSM 5 PASCAL; http://www.zeiss.com). Images of DR5::GFP in the leaf tip and inflorescence were taken with the Olympus BX51 fluorescence microscope.
Molecular Cloning of the ARF17 Gene
T-DNA insertion in the arf17 mutant plants was validated using primers that specifically amplify the BAR gene: Bar-F and Bar-R. For TAIL-PCR, genomic DNA of the mutant plants served as the template, using the T-DNA left border primers AtLB1, AtLB2, and AtLB3 (Supplemental Table S1) and arbitrary degenerate primers. The TAIL-PCR procedure and arbitrary degenerate primers used were as described (Liu et al., 1995). Linkage analysis of the T-DNA insertion with the mutant phenotype was analyzed with primer AtLB3 and the ARF17-specific primers LP and RP (Supplemental Table S1). The LP/RP primers were also used for detecting ARF17 gene expression.
Genetic Complementation
For complementation, a 4.9-kb ARF17 genomic fragment, which included 1,615 bp of upstream and 567 bp of downstream sequence, was amplified from wild-type genomic DNA using the primer pair CMP-F and CMP-R. For GFP fusion, the ARF17 genomic fragment without a stop codon was cloned into the modified GFP-pCAMBIA1300 vector using primer pair CMP-F and CMP-F/FU (Supplemental Table S1). All amplified DNA fragments were cloned into pMD18-T (Takara) for sequencing and subcloning. After verification by sequencing, the fragments were released by restriction enzyme digestion and subcloned into the binary vector pCAMBIA1300 (CAMBIA). The resulting constructs were introduced into heterozygous arf17 plants using the flower dip method (Clough and Bent, 1998) with Agrobacterium tumefaciens strain GV3101 (pMP90). Hygromycin-resistant transformants were identified in the arf17 background using primer pairs MC-F/RP and MC-F/AtLB3 (Supplemental Table S1).
RT-PCR and Real-Time PCR Analysis
Total RNA was isolated from floral buds using the TRIzol kit (Invitrogen). After treatment with DNase (Promega), first-strand complementary DNA (cDNA) was synthesized from 2 μg of RNA using a poly(dT)12-18 primer and avian myeloblastosis virus reverse transcription according to the manufacturer’s instructions (Toyobo). Transcripts were quantified by RT-PCR or real-time RT-PCR analyses using 1/100 of the resulting cDNA as template under the following conditions: denaturation for 30 s at 94°C, annealing for 30 s at 58°C, and extension for 40 s at 72°C, followed by a final extension for 5 min. Real-time PCR was performed using the ABI 7300 System (Applied Biosystems; http://www.appliedbiosystems.com) with the SYBR Green Real-Time PCR Master Mix (Toyobo). A positive control was provided by a parallel analysis based on the tubulin gene, and three replicates were performed per experiment. The relative expression levels were calculated according to the cycle number. Relevant primer sequences are listed in Supplemental Table S1.
In Situ Hybridization
The probe fragments were amplified from wild-type Col-0 cDNA using gene-specific primers (CalS5in-F and CalS5in-R; ARF17in-F and ARF17in-R). After sequencing, the PCR products were cloned into the pBluescript SK−vector. Plasmid DNA was completely digested with EcoRI or BamHI. The digestion products were used as templates for transcription into sense and antisense probes by T3 and T7 RNA polymerases, respectively (Roche). Images were taken using the Olympus BX-51 microscope.
ChIP
The ChIP procedure was performed on 35-d-old flower buds as described by Haring et al. (2007) with some modifications. Flower buds (0.8–1.0 g) were cross linked with 37 mL of 1% formaldehyde in a vacuum for 15 min, and the reaction was stopped by the addition of 1.85 mL of 2 m Gly. After rinsing with water, buds were ground in liquid nitrogen, the powder was suspended in 25 mL of extraction buffer I (0.4 m Suc, 10 mm Tris-HCl, pH 8, 10 mm MgCl2, 5 mm β-mercaptoethanol, 0.1 mm phenylmethylsulfonyl fluoride [PMSF], and 1× protease inhibitor; Sigma-Aldrich) and then filtered through a 40-μm cell strainer (BD Falcon). The filtrate was centrifuged at 2,900g at 4°C for 20 min, and the pellet was resuspended in 1 mL of extraction buffer II (0.25 m Suc, 10 mm Tris-HCl, pH 8, 10 mm MgCl2, 1% Triton X-100, 5 mm β-mercaptoethanol, 0.1 mm PMSF, and 1× protease inhibitor) and centrifuged at 16,000 rpm at 4°C for 10 min. The pellet was resuspended in 300 μL of extraction buffer III and loaded on top of an equal amount buffer III (1.7 m Suc, 10 mm Tris-HCl, pH 8, 0.15% Triton X-100, 2 mm MgCl2, 5 mm β-mercaptoethanol, 0.1 mm PMSF, and 1× protease inhibitor), then centrifuged at 16,000g for 60 min. The resulting nuclear pellet was resuspended in nuclear lysis buffer (50 mm Tris-HCl, pH 8.0, 10 mm EDTA, 1% SDS, and 1× Complete protease inhibitor; Roche) and sonicated to give 0.3- to 0.8-kb DNA fragments. The soluble chromatin solution was diluted 10-fold with ChIP dilution buffer (1.1% Triton X-100, 1.2 mm EDTA, 16.7 mm Tris-HCl, pH 8.0, and 167 mm NaCl) after preclearing with protein A-Dynabeads (Invitrogen), and 1 μL of GFP antibody (Sigma-Aldrich) was added to 1 mL of chromatin solution and incubated overnight at 4°C. The immunocomplexes were extracted by incubating with 100 mL of 50% protein G-Sepharose beads for 3 h at 4°C. After several washes, immunocomplexes were eluted with elution buffer (1% SDS and 0.1 m NaHCO3), then reverse cross linked at 65°C overnight. After treatment with proteinase K, DNA was purified. The pellet was dissolved with 100 μL of water and used for real-time PCR analysis using relevant primers listed in Supplemental Table S1.
EMSA
To obtain purified ARF17 protein for the EMSA experiments, the full-length fragment of the ARF17 gene was amplified using the primer pairs ARF17GST-F and ARF17GST-R and cloned into the pGEX-4T vector (GE Healthcare; http://www.gehealthcare.com) to produce the GST-ARF17 construct. Expression and purification of the fusion protein were performed according to the manufacturer’s instructions. The DNA fragment containing the AuxRE (GAGACA) in the ARF17 regulatory region was generated by PCR amplification with the following specific primers (EMSAbio-F/EMSAbio-R and EMSAcomp-F/EMSAcomp-R) that were used to generate biotin-labeled and competitor probes, respectively. The EMSA was performed with a LightShift Chemiluminescent EMSA Kit (Thermo Scientific; http://www.thermoscientific.com). The binding reactions containing binding buffer [10 mm Tris-HCl, pH 7.5, 50 mm NaCl, 1 mm EDTA, 5% glycerol, and 0.05 mg mL−1 poly(dI-dC)], 0.5 μg of GST-ARF17 recombinant fusion protein, and 10 fmol of biotin-labeled DNA were performed at room temperature for 20 min. The subsequent processes were performed according to the manufacturer’s instructions.
Sequence data from this article can be found in the GenBank/EMBL data libraries under accession numbers ARF17 (AT1G77850), CalS5 (AT2G13680), CDKG1 (AT5G63370), DEX1 (AT3G09090), NEF1 (AT5G13390), NPU (AT3G51610), RPG1 (AT5G40260), and TUB (AT5G23860).
Supplemental Data
The following materials are available in the online version of this article.
Supplemental Figure S1. Genetic complementation of the arf17 mutant.
Supplemental Figure S2. Meiosis observation in arf17 anther.
Supplemental Figure S3. Expression analysis of primexine-related genes in the wild type and arf17.
Supplemental Figure S4. Analysis of pollen development in arf17/+qrt1 plants.
Supplemental Table S1. List of primers used in this study.
Glossary
- AuxRE
auxin response element
- TAIL
thermal asymmetric interlaced
- Col-0
Columbia-0
- DAPI
4,6-diamidino-2-phenylindole
- TEM
transmission electron microscopy
- RT
reverse transcription
- ChIP
chromatin immunoprecipitation
- PMSF
phenylmethylsulfonyl fluoride
- cDNA
complementary DNA
- T-DNA
transfer DNA
LITERATURE CITED
Author notes
This work was supported by the Major Research Plan from the Ministry of Science and Technology of China (grant no. 2013CB945100), the National Science Foundation of China (grant no. 30925007), and the Leading Academic Discipline Project of the Shanghai Municipal Education Commission (grant no. J50401).
Corresponding author; e-mail [email protected].
The author responsible for distribution of materials integral to the findings presented in this article in accordance with the policy described in the Instructions for Authors (www.plantphysiol.org) is: Zhong-Nan Yang ([email protected]).
Some figures in this article are displayed in color online but in black and white in the print edition.
The online version of this article contains Web-only data.
Open Access articles can be viewed online without a subscription.