-
PDF
- Split View
-
Views
-
Cite
Cite
Sylviane Comparot-Moss, Oliver Koݶtting, Michaela Stettler, Christoph Edner, Alexander Graf, Sean E. Weise, Sebastian Streb, Wei-Ling Lue, Daniel MacLean, Sebastian Mahlow, Gerhard Ritte, Martin Steup, Jychian Chen, Samuel C. Zeeman, Alison M. Smith, A Putative Phosphatase, LSF1, Is Required for Normal Starch Turnover in Arabidopsis Leaves, Plant Physiology, Volume 152, Issue 2, February 2010, Pages 685–697, https://doi.org/10.1104/pp.109.148981
- Share Icon Share
Abstract
A putative phosphatase, LSF1 (for LIKE SEX4; previously PTPKIS2), is closely related in sequence and structure to STARCH-EXCESS4 (SEX4), an enzyme necessary for the removal of phosphate groups from starch polymers during starch degradation in Arabidopsis (Arabidopsis thaliana) leaves at night. We show that LSF1 is also required for starch degradation: lsf1 mutants, like sex4 mutants, have substantially more starch in their leaves than wild-type plants throughout the diurnal cycle. LSF1 is chloroplastic and is located on the surface of starch granules. lsf1 and sex4 mutants show similar, extensive changes relative to wild-type plants in the expression of sugar-sensitive genes. However, although LSF1 and SEX4 are probably both involved in the early stages of starch degradation, we show that LSF1 neither catalyzes the same reaction as SEX4 nor mediates a sequential step in the pathway. Evidence includes the contents and metabolism of phosphorylated glucans in the single mutants. The sex4 mutant accumulates soluble phospho-oligosaccharides undetectable in wild-type plants and is deficient in a starch granule-dephosphorylating activity present in wild-type plants. The lsf1 mutant displays neither of these phenotypes. The phenotype of the lsf1/sex4 double mutant also differs from that of both single mutants in several respects. We discuss the possible role of the LSF1 protein in starch degradation.
Starch accumulates in chloroplasts during the day as a product of photosynthesis and is mobilized at night to provide a continuing supply of sugars to the plant. In nonflowering rosettes of Arabidopsis (Arabidopsis thaliana) grown in controlled conditions, starch degradation is essentially linear, and the rate is such that about 95% of the starch present at the end of the day is mobilized during the night (Smith and Stitt, 2007). The importance of this pattern of starch degradation in maintaining the sugar supply of the plant is illustrated by experiments in which the night is extended beyond the normal period and by the phenotype of mutant plants that are unable to make starch during the day. Extension of the night by as little as 2 h for plants grown in a 12-h-light/12-h-dark regime causes cessation of root elongation and changes in the transcriptome interpretable as a pronounced “starvation response.” These responses also occur during normal 12-h nights in the phosphoglucomutase (pgm) mutant, which cannot synthesize starch: the mutant grows more slowly than wild-type plants in most conditions (Gibon et al., 2004; Smith and Stitt, 2007; Stitt et al., 2007).
The way in which the rate of degradation is controlled remains largely unknown, but there is good evidence for an important role for enzymes that catalyze the phosphorylation of the Glc polymers (glucans) of which the starch granule is composed. Two enzymes that catalyze starch phosphorylation, glucan, water dikinase (GWD) and phosphoglucan, water dikinase (PWD; also called GWD3), are required for normal rates of starch degradation (Yu et al., 2001; Ritte et al., 2002; Baunsgaard et al., 2005; Koݶtting et al., 2005; Ritte et al., 2006). Mutants lacking either enzyme grow slowly, have reduced rates of starch degradation, and display starch-excess phenotypes; that is, they retain high levels of starch in their leaves at the end of the night. The phenotype of mutants lacking GWD (sex1 mutants; Yu et al., 2001) is more pronounced in all of these respects than that of pwd mutants. It is thought that phosphorylation mediates the transition of glucans at the granule surface from a highly ordered to a less ordered and more hydrated state, facilitating their degradation by hydrolytic enzymes (Yu et al., 2001; Ritte et al., 2002; Koݶtting et al., 2005; Edner et al., 2007; Hejazi et al., 2008).
It has recently become clear that dephosphorylation of glucans is also essential for normal starch degradation in Arabidopsis leaves. We discovered that the starch-excess4 (sex4) mutant lacks a phosphatase with a carbohydrate-binding domain (Niittylaݶ et al., 2006). Although it was originally described as a dual-specificity protein phosphatase (PTPKIS1; Fordham-Skelton et al., 2002; Kerk et al., 2006), we have shown that the function of the SEX4 phosphatase is to remove phosphate groups added to starch by GWD (and perhaps PWD), thus facilitating the complete degradation of oligosaccharides by β-amylase (Koݶtting et al., 2009). Recombinant SEX4 catalyzes the dephosphorylation of glucans, including starch polymers, glycogen, soluble oligosaccharides, and leaf starch granules (Gentry et al., 2007). sex4 mutant plants have greatly elevated levels of phosphorylated malto-oligosaccharides (Koݶtting et al., 2009). These are released from the starch granule primarily by the actions of a chloroplastic α-amylase (AMY3) and the debranching enzyme isoamylase 3 (ISA3) but cannot be metabolized further in the absence of an appropriate phosphatase. Thus, glucan-phosphorylating and -dephosphorylating enzymes appear to work together with hydrolytic enzymes during starch degradation in leaves at night.
The Arabidopsis genome contains two further genes encoding proteins with predicted dual-specificity phosphatase domains of high similarity to the SEX4 phosphatase domain (At3g01510 and At3g10940; Fordham-Skelton et al., 2002). We refer to these proteins as LIKE SEX4 (LSF1 and LSF2, respectively). In addition to the phosphatase domain, LSF1 possesses a putative carbohydrate-binding domain, likewise similar to that of SEX4. Since this carbohydrate-binding domain is missing in LSF2, we have focused on the analysis of LSF1 function. Here, we report that LSF1 is a chloroplastic protein essential for normal starch degradation. Genetic and transcriptional analyses indicate that it has a function in this process that is distinct from that of SEX4 and may be regulatory in nature.
RESULTS
LSF1 Is a Chloroplastic Protein
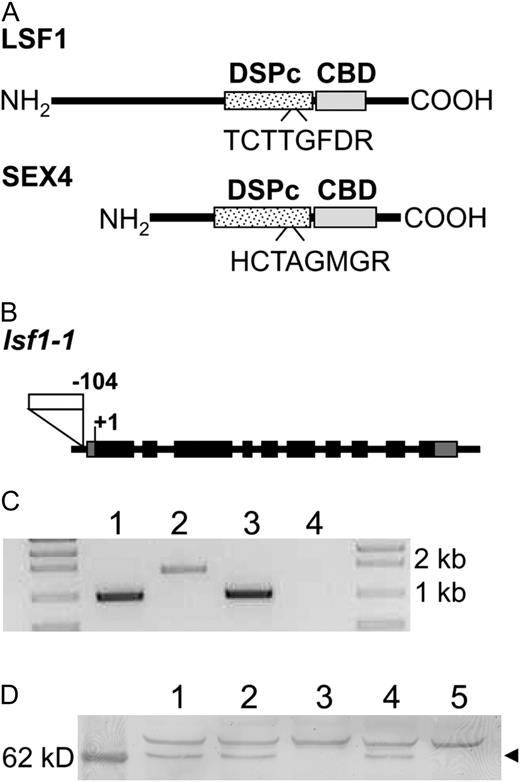
Features of LSF1 and the lsf1 mutant. A, For LSF1 and SEX4 proteins, the predicted positions of the putative carbohydrate-binding domain (CBD) and the DSPc are indicated as boxes, and the conserved motifs of the DSPc domains are indicated. B, Exon and intron structure of the LSF1 gene, showing the location of the T-DNA insertion in the lsf1-1 mutant 104 bp upstream of the ATG start codon. Black boxes indicate exons; gray boxes are the 5′ and 3′ untranslated regions; the white box is the T-DNA insertion. C, LSF1 mRNA levels in wild-type and mutant plants. Total RNA extracted from wild-type (lanes 1 and 2) and homozygous lsf1 mutant (lanes 3 and 4) plants was analyzed by RT-PCR. Reactions contained primers amplifying the following products: a TUBULIN 1-kb fragment (positive control; lanes 1 and 3) and a LSF1 1.7-kb fragment (lanes 2 and 4). D, LSF1 protein levels in wild-type and mutant plants. Immunoblot analysis was on leaf extracts from Col-0 (lane 1), a wild type selected from a population segregating for lsf1-1 mutants (lane 2), lsf1-1 (lane 3), sex4-3 (lane 4), and lsf1-1/sex4-3 (lane 5) using an antiserum raised to a LSF1 peptide. The left lane is a molecular mass marker. The arrowhead indicates a band of approximately 64 kD, the appropriate mass for the mature LSF1 protein.
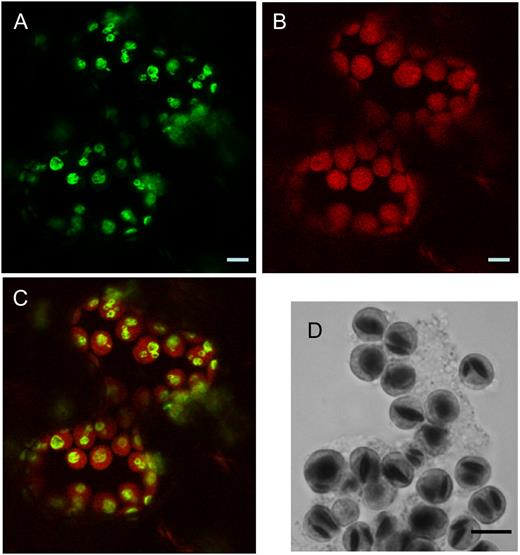
Subcellular localization of LSF1 in N. benthamiana leaves. A, Confocal microscopy of leaf cells transiently expressing 35S∷LSF1-GFP, showing the localization of GFP fluorescence. B, Chlorophyll autofluorescence of the same image. C, Merged fluorescence from A and B. D, Light micrograph of chloroplasts isolated from leaf protoplasts and stained with iodine. The dark objects in the chloroplasts are starch granules. Images were taken in the middle of the photoperiod. Bars = 5 μm.
The nonuniform distribution of fluorescence from the LSF1-GFP fusion protein within the chloroplasts in N. benthamiana leaves (Fig. 2C) suggested that the protein was located on the surface of starch granules. Iodine staining of chloroplasts isolated from N. benthamiana leaf protoplasts showed that the starch granules were similar in size and distribution to areas of GFP fluorescence in the intact leaf (Fig. 2D). SEX4 protein is also reported to be localized to the surface of starch granules in chloroplasts (Sokolov et al., 2006). The nonuniform distribution of fluorescence from the LSF1-GFP fusion protein strongly resembled that from a chloroplast-targeted yellow fluorescent protein fusion of the starch-binding domain (a family 20 carbohydrate-binding module) of PWD. The PWD starch-binding domain also binds to starch granules in vitro (Christiansen et al., 2009).
Isolation of an lsf1 Mutant
One line carrying a T-DNA insertion at the LSF1 locus was found in the SALK collection. The T-DNA is inserted in the promoter region of At3g01510 (™104 bp with respect with the start codon; SALK_053285; Fig. 1B). Plants homozygous for this insertion lacked detectable LSF1 transcript (Fig. 1C). A second line with a T-DNA insertion in the promoter region (™145 bp with respect to the start codon; SALK_100036) is also available, but a full-length transcript was detected in homozygous plants (data not shown).
To check whether the LSF1 protein is absent from the line lacking LSF1 transcript (named lsf1-1), an antiserum was raised against a peptide located toward the C terminus of the protein (amino acids 484–497). This peptide is absent from the SEX4 protein. On immunoblots of extracts of wild-type leaves, the antiserum recognized a band of approximately 64 kD (estimated from molecular mass markers), which was absent from equivalent extracts of the lsf1 mutant (Fig. 1D). Since the predicted molecular mass of the LSF1 protein without the transit peptide is 65.7 kD, we conclude that the LSF1 protein is absent from the lsf1 mutant.
Phenotypic Characterization of the lsf1 Mutant
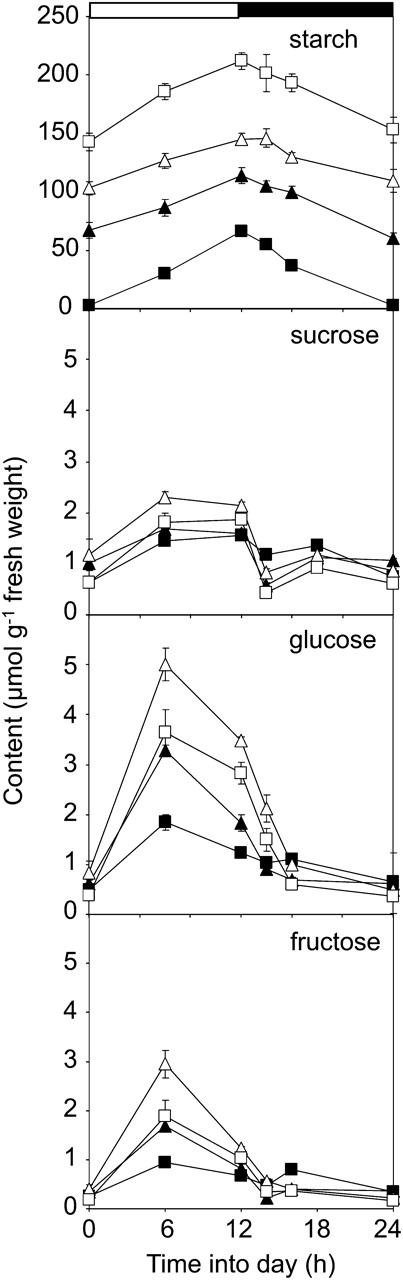
Diurnal changes in starch and sugar contents. Plants were grown in a controlled-environment room with 12 h of light, 12 h of dark at 20°C and approximately 170 μmol quanta m™2 s™1. Starch (as μmol Glc equivalents g™1 fresh weight) and sugar contents were measured on whole rosettes harvested over a 24-h period, starting at dawn. Data are means ± se of measurements on six rosettes per time point. Black squares, wild type (Col-0); black triangles, lsf1-1; white triangles, sex4-3; white squares, lsf1-1/sex4-3.
We took two further steps to check whether the T-DNA insertion into the LSF1 gene was responsible for the starch-excess phenotype of lsf1-1. First, we looked for possible lsf1 mutants in our collections of unidentified starch-excess mutants isolated from forward genetic screens. The mutation responsible for the starch-excess phenotype in line Ke103 (Chen Collection, Academia Sinica, Taipei) had previously been located by genetic mapping to the region of chromosome 3 containing the LSF1 gene (data not shown). Measurement of the starch content of Ke103 over 24 h revealed a pattern very similar to that of the lsf1 mutant (Supplemental Fig. S4A). Reciprocal crosses between Ke103 and the lsf1 mutant confirmed that the F1 plants retained a starch-excess phenotype (data not shown). Sequencing of the LSF1 gene from Ke103 and its wild-type progenitor (ecotype RLD) revealed two single-base-pair deletions in the mutant. The first one is in an intron and therefore silent. The second is at the splice junction between exon 1 and intron 1 and is predicted to create a frame shift leading to an in-frame stop codon, truncating the protein after amino acid 115 (Supplemental Fig. S4B). Immunoblotting with an antiserum raised to the entire mature LSF1 protein failed to detect a protein of the appropriate size in extracts of either lsf1-1 or Ke103 leaves (Supplemental Fig. S2C). Ke103 was renamed lsf1-2.
Second, we attempted to complement the starch-excess phenotype of lsf1-1 by introducing a construct containing the full-length cDNA encoding wild-type LSF1 (35S∷LSF1). Transformed plants expressed LSF1 protein and had levels of starch similar to those of wild-type plants at both the end of the day and the end of the night (Supplemental Fig. S5). Taken together, these approaches provide unambiguous evidence that the LSF1 protein is required for normal starch turnover in Arabidopsis.
Impact of the lsf1 Mutation on Chloroplastic α-Amylase
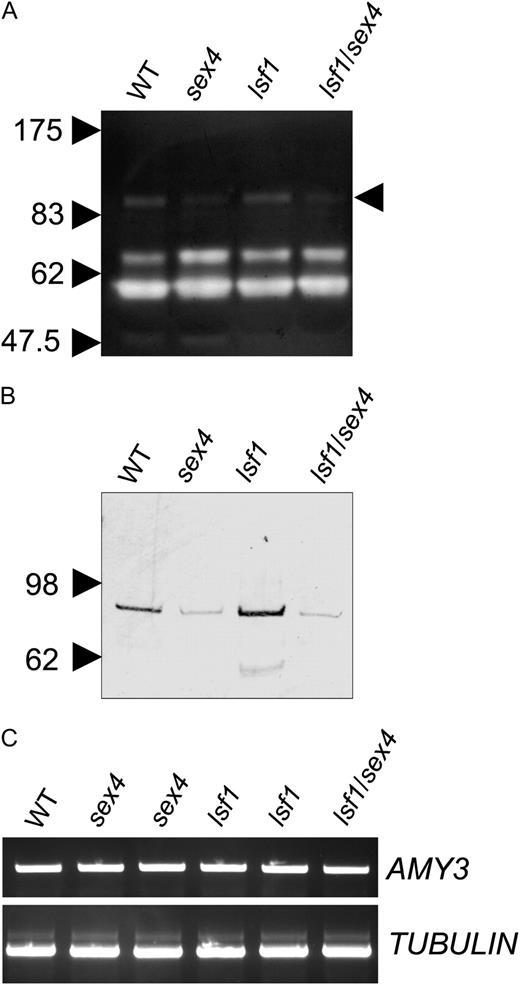
Effects of mutations on expression of the chloroplastic α-amylase AMY3. A, AMY3 activity. A reconstituted amylopectin-containing SDS-polyacrylamide gel, stained with iodine solution after incubation to reveal positions of starch-degrading enzymes, is shown. The positions of molecular mass markers on the same gel are indicated on the left; the position of the AMY3 band is indicated on the right (Yu et al., 2005). WT, Wild type. B, AMY3 protein. Soluble extracts of leaves of wild-type and mutant plants were subjected to SDS-PAGE, blotted onto nitrocellulose membrane, and probed with antiserum to AMY3 as described by Yu et al. (2005). C, AMY3 transcript. Total RNA was extracted from Col-0, sex4-3, and lsf1-1 leaves of plants 9 h into the light period and analyzed by RT-PCR. Reactions contained primers amplifying the following products: an AMY3 1.1-kb fragment (top) and a TUBULIN 1-kb fragment (bottom).
Phenotypic Characterization of the lsf1/sex4 Double Mutant
To shed light on the function of LSF1, we generated a double mutant (lsf1/sex4) using as parents the SALK lines 102567 (sex4-3; Niittylaݶ et al., 2006) and 053285 (lsf1-1) and compared its phenotype with that of the single sex4 and lsf1 mutants. At the same age, nonflowering rosettes of lsf1 and sex4 weighed about 25% and 65% less, respectively, than those of wild-type plants. Double mutants grew more slowly than lsf1 mutant plants and were comparable in weight with sex4 plants (Supplemental Fig. S2B).
Double mutant plants had higher starch contents, at the end of both the light and the dark periods, than wild-type plants and both the lsf1 and sex4 mutants (Fig. 3). However, there was still appreciable starch synthesis during the day and starch degradation at night in all of the mutants. In the experiment shown in Figure 3, the losses of starch over the entire dark period in sex4, lsf1, and lsf1/sex4 plants were 84%, 55%, and 92%, respectively, of that in wild-type plants. In a further, independently grown batch of plants, we obtained values of 99%, 109%, and 99%. This situation contrasts sharply with that in the sex1 mutant, in which the phosphorylation of starch is prevented by the loss of GWD. Under our growth conditions, levels of starch in sex1 rosettes are higher than those in the lsf1/sex4 mutant and change very little over the day/night cycle (Niittylaݶ et al., 2006).
As reported previously for the sex4 mutant (Zeeman and ap Rees, 1999), Glc and Fru contents were substantially higher during the middle part of the light period in all of the mutants than in wild-type plants. This elevation was more pronounced in sex4 than in either lsf1 or lsf1/sex4 (Fig. 3). Thus, although the double mutant had higher levels of starch than either sex4 or lsf1, its sugar content during the day was lower than that of sex4 and similar to that of lsf1. During the first 4 to 6 h of the night, there were larger decreases in levels of sugars in all three mutants than in wild-type plants. However, during the remainder of the night, sugar levels in the mutant plants fell less than in wild-type plants. There was no apparent difference in sugar levels between mutant and wild-type plants at the end of the night.
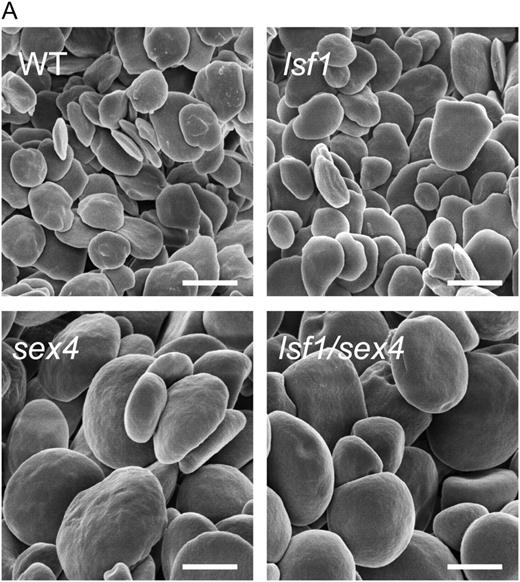
Starch granules from mutant and wild-type (WT) plants. Plants were harvested at the end of a light period. Starch granules were extracted as described by Koݶtting et al. (2005). Scanning electron micrographs were taken with a Zeiss Gemini 1530 FEG apparatus. Bars = 2 μm.
Impact of the lsf1 Mutation on Glucan Phosphorylation
To examine the possibility that LSF1, like SEX4, is a glucan phosphatase, we measured the phosphate content of leaf starch and soluble glucans and amounts of phosphorylated soluble glucans (phospho-oligosaccharides) in leaves of wild-type and mutant plants. We discovered recently that substantial amounts of soluble phospho-oligosaccharides accumulate in leaves of sex4 mutant plants but not wild-type plants. This observation provides strong support for the idea that SEX4 dephosphorylates glucans during starch degradation (Koݶtting et al., 2009). Soluble phospho-oligosaccharides were present in lsf1/sex4 plants (Table I
Phosphate content of leaf starch and phospho-oligosaccharide content in wild-type and mutant plants
Genotypea . | Starchb . | Phospho-oligosaccharidesc . | Total . | Starchd . | Amylopectin . |
---|---|---|---|---|---|
nmol phosphate g™1 fresh weight | nmol phosphate g™1 fresh weight | nmol phosphate g™1 fresh weight | nmol phosphate μmol™1 Glc equivalents | nmol phosphate μmol™1 Glc equivalents | |
Wild type | 89 ± 8 | n.d. | 89 ± 8 | 1.34 ± 0.01 | 1.46 ± 0.11 |
lsf1 | 176 ± 21 | n.d. | 176 ± 21 | 1.55 ± 0.16 | 1.82 ± 0.19 |
sex4 | 135 ± 6 | 452 ± 86 | 587 ± 110 | 0.93 ± 0.02 | 1.43 ± 0.04 |
lsf1/sex4 | 209 ± 8 | 366 ± 33 | 574 ± 56 | 0.98 ± 0.02 | 1.42 ± 0.04 |
Genotypea . | Starchb . | Phospho-oligosaccharidesc . | Total . | Starchd . | Amylopectin . |
---|---|---|---|---|---|
nmol phosphate g™1 fresh weight | nmol phosphate g™1 fresh weight | nmol phosphate g™1 fresh weight | nmol phosphate μmol™1 Glc equivalents | nmol phosphate μmol™1 Glc equivalents | |
Wild type | 89 ± 8 | n.d. | 89 ± 8 | 1.34 ± 0.01 | 1.46 ± 0.11 |
lsf1 | 176 ± 21 | n.d. | 176 ± 21 | 1.55 ± 0.16 | 1.82 ± 0.19 |
sex4 | 135 ± 6 | 452 ± 86 | 587 ± 110 | 0.93 ± 0.02 | 1.43 ± 0.04 |
lsf1/sex4 | 209 ± 8 | 366 ± 33 | 574 ± 56 | 0.98 ± 0.02 | 1.42 ± 0.04 |
Data for the wild type and sex4 are from Koݶtting et al. (2009). All plants were grown and harvested under the same conditions.
Values are means ± se from five biological replicates. Values for phosphate content of starch for all three mutants are statistically significantly different from the wild-type value (P < 0.05, Student's t test).
The phosphate content of phospho-oligosaccharides is underestimated, because it was assumed that each phospho-oligosaccharide is only singly phosphorylated. n.d., Not detected.
Arabidopsis plants were harvested at the end of the light period. Leaf starch was purified and the amylopectin content was determined to be 91.8% ± 0.1% for the wild type, 85.1% ± 0.4% for lsf1, 65.2% ± 1.1% for sex4, and 68.9% ± 0.4% for lsf1/sex4 (means ± se; n = 3). Starch-bound phosphate from the same starch preparations was determined. None of the values for mutants are statistically significantly different from the wild-type values (P > 0.05, Student's t test).
Phosphate content of leaf starch and phospho-oligosaccharide content in wild-type and mutant plants
Genotypea . | Starchb . | Phospho-oligosaccharidesc . | Total . | Starchd . | Amylopectin . |
---|---|---|---|---|---|
nmol phosphate g™1 fresh weight | nmol phosphate g™1 fresh weight | nmol phosphate g™1 fresh weight | nmol phosphate μmol™1 Glc equivalents | nmol phosphate μmol™1 Glc equivalents | |
Wild type | 89 ± 8 | n.d. | 89 ± 8 | 1.34 ± 0.01 | 1.46 ± 0.11 |
lsf1 | 176 ± 21 | n.d. | 176 ± 21 | 1.55 ± 0.16 | 1.82 ± 0.19 |
sex4 | 135 ± 6 | 452 ± 86 | 587 ± 110 | 0.93 ± 0.02 | 1.43 ± 0.04 |
lsf1/sex4 | 209 ± 8 | 366 ± 33 | 574 ± 56 | 0.98 ± 0.02 | 1.42 ± 0.04 |
Genotypea . | Starchb . | Phospho-oligosaccharidesc . | Total . | Starchd . | Amylopectin . |
---|---|---|---|---|---|
nmol phosphate g™1 fresh weight | nmol phosphate g™1 fresh weight | nmol phosphate g™1 fresh weight | nmol phosphate μmol™1 Glc equivalents | nmol phosphate μmol™1 Glc equivalents | |
Wild type | 89 ± 8 | n.d. | 89 ± 8 | 1.34 ± 0.01 | 1.46 ± 0.11 |
lsf1 | 176 ± 21 | n.d. | 176 ± 21 | 1.55 ± 0.16 | 1.82 ± 0.19 |
sex4 | 135 ± 6 | 452 ± 86 | 587 ± 110 | 0.93 ± 0.02 | 1.43 ± 0.04 |
lsf1/sex4 | 209 ± 8 | 366 ± 33 | 574 ± 56 | 0.98 ± 0.02 | 1.42 ± 0.04 |
Data for the wild type and sex4 are from Koݶtting et al. (2009). All plants were grown and harvested under the same conditions.
Values are means ± se from five biological replicates. Values for phosphate content of starch for all three mutants are statistically significantly different from the wild-type value (P < 0.05, Student's t test).
The phosphate content of phospho-oligosaccharides is underestimated, because it was assumed that each phospho-oligosaccharide is only singly phosphorylated. n.d., Not detected.
Arabidopsis plants were harvested at the end of the light period. Leaf starch was purified and the amylopectin content was determined to be 91.8% ± 0.1% for the wild type, 85.1% ± 0.4% for lsf1, 65.2% ± 1.1% for sex4, and 68.9% ± 0.4% for lsf1/sex4 (means ± se; n = 3). Starch-bound phosphate from the same starch preparations was determined. None of the values for mutants are statistically significantly different from the wild-type values (P > 0.05, Student's t test).
The elevated levels of starch-bound phosphate in the mutants reflected the elevated starch contents per gram fresh weight in these plants, rather than differences in the amount of phosphate per weight of starch (Table I). When expressed on a starch basis, the phosphate content of leaf starch was not significantly different in wild-type and lsf1 plants but was lower in both the sex4 mutant (Koݶtting et al., 2009) and the double mutant (Table I). The lower levels are accounted for by differences in amylose content between the starches. The amylose component of starch is not phosphorylated: phosphorylation is essentially confined to the amylopectin component (Blennow et al., 2002). When phosphate content was expressed on an amylopectin basis, there was no statistically significant difference between wild-type plants and any of the mutant plants (Table I).
We also examined the ability of crude extracts of protein from single and double mutant leaves to release phosphate from purified starch granules that had been previously labeled with 33P by incubation with recombinant GWD. Extracts of sex4 and lsf1/sex4 leaves released less phosphate than extracts of wild-type leaves (60% and 70% less, respectively), but phosphate release was unaltered in extracts of lsf1 leaves (Table II
Release of 33P from isolated starch granules by leaf extracts
Purified starch granules from the GWD-deficient Arabidopsis mutants sex1-3 (Yu et al., 2001) and sex1-8 were phosphorylated in vitro with recombinant potato (Solanum tuberosum) GWD (Ritte et al., 2002) as described by Edner et al. (2007). 33P-labeled starch was incubated with desalted extracts of whole rosettes of sex4, lsf1, and lsf1/sex4 plants. Phosphate release over time was linear under these conditions. Data are means ± se of values from five independent extracts
Genotype . | Release of 33P as a Percentage of Release by Wild-Type Extracts . |
---|---|
sex4 | 39.0 ± 3.1 |
lsf1 | 102.2 ± 8.3 |
lsf1/sex4 | 25.8 ± 6.6 |
Genotype . | Release of 33P as a Percentage of Release by Wild-Type Extracts . |
---|---|
sex4 | 39.0 ± 3.1 |
lsf1 | 102.2 ± 8.3 |
lsf1/sex4 | 25.8 ± 6.6 |
Release of 33P from isolated starch granules by leaf extracts
Purified starch granules from the GWD-deficient Arabidopsis mutants sex1-3 (Yu et al., 2001) and sex1-8 were phosphorylated in vitro with recombinant potato (Solanum tuberosum) GWD (Ritte et al., 2002) as described by Edner et al. (2007). 33P-labeled starch was incubated with desalted extracts of whole rosettes of sex4, lsf1, and lsf1/sex4 plants. Phosphate release over time was linear under these conditions. Data are means ± se of values from five independent extracts
Genotype . | Release of 33P as a Percentage of Release by Wild-Type Extracts . |
---|---|
sex4 | 39.0 ± 3.1 |
lsf1 | 102.2 ± 8.3 |
lsf1/sex4 | 25.8 ± 6.6 |
Genotype . | Release of 33P as a Percentage of Release by Wild-Type Extracts . |
---|---|
sex4 | 39.0 ± 3.1 |
lsf1 | 102.2 ± 8.3 |
lsf1/sex4 | 25.8 ± 6.6 |
We considered the possibility that LSF1 specifically removes phosphate from the three-position of glucosyl residues of amylopectin. If this is the case, the analyses above might fail to detect its activity. GWD adds phosphate to the six-position of Glc residues in amylopectin, whereas the second starch-phosphorylating enzyme required for starch degradation, PWD, adds phosphate groups to the three-position (Baunsgaard et al., 2005; Koݶtting et al., 2005; Ritte et al., 2006). In wild-type Arabidopsis starch, the ratio of Glc 6-P to Glc 3-P residues is about 5:1 (Haebel et al., 2008; Table III
Glc 3-P and Glc 6-P contents of leaf starch in wild-type and mutant plants
Leaf starch was purified from plants harvested at the end of the light period. For each genotype, two 5-mg samples were taken from a batch of starch from several pooled plants. Each sample was hydrolyzed, and two separate measurements were made on each hydrolysate. Glc phosphates were separated by HPAEC-PAD using a Dionex ICS-3000 HPLC system with a PA1 column. Values are means ± se from four measurements.
Genotype . | Glc 6-P . | Glc 3-P . | Ratio Glc 3-P/Glc 6-P . |
---|---|---|---|
nmol μmol™1 Glc equivalents | nmol μmol™1 Glc equivalents | ||
Col-0 | 0.91 ± 0.15 | 0.15 ± 0.03 | 0.161 ± 0.011 |
lsf1 | 0.87 ± 0.13 | 0.14 ± 0.02 | 0.158 ± 0.013 |
sex4 | 0.52 ± 0.09 | 0.06 ± 0.01 | 0.124 ± 0.013 |
lsf1/sex4 | 0.63 ± 0.10 | 0.09 ± 0.02 | 0.144 ± 0.010 |
Genotype . | Glc 6-P . | Glc 3-P . | Ratio Glc 3-P/Glc 6-P . |
---|---|---|---|
nmol μmol™1 Glc equivalents | nmol μmol™1 Glc equivalents | ||
Col-0 | 0.91 ± 0.15 | 0.15 ± 0.03 | 0.161 ± 0.011 |
lsf1 | 0.87 ± 0.13 | 0.14 ± 0.02 | 0.158 ± 0.013 |
sex4 | 0.52 ± 0.09 | 0.06 ± 0.01 | 0.124 ± 0.013 |
lsf1/sex4 | 0.63 ± 0.10 | 0.09 ± 0.02 | 0.144 ± 0.010 |
Glc 3-P and Glc 6-P contents of leaf starch in wild-type and mutant plants
Leaf starch was purified from plants harvested at the end of the light period. For each genotype, two 5-mg samples were taken from a batch of starch from several pooled plants. Each sample was hydrolyzed, and two separate measurements were made on each hydrolysate. Glc phosphates were separated by HPAEC-PAD using a Dionex ICS-3000 HPLC system with a PA1 column. Values are means ± se from four measurements.
Genotype . | Glc 6-P . | Glc 3-P . | Ratio Glc 3-P/Glc 6-P . |
---|---|---|---|
nmol μmol™1 Glc equivalents | nmol μmol™1 Glc equivalents | ||
Col-0 | 0.91 ± 0.15 | 0.15 ± 0.03 | 0.161 ± 0.011 |
lsf1 | 0.87 ± 0.13 | 0.14 ± 0.02 | 0.158 ± 0.013 |
sex4 | 0.52 ± 0.09 | 0.06 ± 0.01 | 0.124 ± 0.013 |
lsf1/sex4 | 0.63 ± 0.10 | 0.09 ± 0.02 | 0.144 ± 0.010 |
Genotype . | Glc 6-P . | Glc 3-P . | Ratio Glc 3-P/Glc 6-P . |
---|---|---|---|
nmol μmol™1 Glc equivalents | nmol μmol™1 Glc equivalents | ||
Col-0 | 0.91 ± 0.15 | 0.15 ± 0.03 | 0.161 ± 0.011 |
lsf1 | 0.87 ± 0.13 | 0.14 ± 0.02 | 0.158 ± 0.013 |
sex4 | 0.52 ± 0.09 | 0.06 ± 0.01 | 0.124 ± 0.013 |
lsf1/sex4 | 0.63 ± 0.10 | 0.09 ± 0.02 | 0.144 ± 0.010 |
Hydrolysis of starch followed by anion-exchange chromatography with pulsed amperometric detection revealed that ratios of Glc 6-P to Glc 3-P residues at the end of the day were the same in wild-type and lsf1 plants and differed from the wild-type value by less than 25% in sex4 and lsf1/sex4 plants (Table III).
Transcriptional Analysis of lsf1 and sex4 Mutants
To provide further information about any differences in function between LSF1 and SEX4, we used microarray analysis to compare the transcriptomes of 4-week-old lsf1-1, wild-type (ecotype Columbia [Col-0]), and sex4-3 plants. Plants were grown in 12-h-light/12-h-dark conditions and harvested immediately before the start of the light period. The experiment was repeated three times on separately grown batches of plants. For each of the two mutants, data from the three repetitions were very similar (pairwise comparisons, r2 > 0.98) and were pooled. For the wild type, two of the three data sets were very similar (r2 > 0.98) and were also similar to publicly available, independent data sets for Col-0 plants grown and harvested under the same conditions (data not shown). The third Col-0 data set gave much lower correlation coefficients than the first two and than independent data sets. This replicate was not used in the subsequent analysis.
The differences in transcript levels between the mutants and Col-0 were generally small and were not statistically significant in most cases. Nonetheless, previously recognized, biologically interpretable patterns were observed consistently (see below). For clarity, therefore, we present data based on a cutoff value rather than a statistical analysis (for comparative purposes, a statistical analysis is presented in Supplemental Table S1, and statistically significant differences are highlighted in other Supplemental Tables based on cutoff values). For transcripts with greater abundance in the mutant than in the wild type, a ratio of 1.5 or greater was required. For transcripts with smaller abundance than in the wild type, a ratio of 0.66 or less was required. Among the 22,750 probe sets, 1,013 exceeded cutoff values in the lsf1 mutant (Supplemental Table S2). These probe sets were assigned to functional categories using the MapMan tool (http://mapman.mpimp-golm.mpg.de/; Supplemental Fig. S8A). Very few were assigned to primary carbohydrate metabolism, and none was assigned to starch synthesis and degradation (as defined by Smith et al. [2004]). Most of the transcripts that differed in abundance were assigned to the RNA, protein, and miscellaneous/unassigned bins (11.7%, 12.2%, and 40.3%, respectively). Other bins that individually accounted for more than 2.5% of the transcripts were stress, signaling, cell wall, hormone, development, secondary metabolism, and transport.
We carried out the same analyses for sex4 (Supplemental Tables S1 and S2; Supplemental Fig. S8B). In this case, only 241 transcripts passed the threshold. As for lsf1, very few of these transcripts were assigned to primary carbohydrate metabolism, and most were assigned to RNA, protein, and miscellaneous/unassigned bins (14.1%, 5.4%, and 40.2%, respectively). There were strong similarities in the transcript profiles of lsf1 and sex4. Of the 241 transcripts that were different in abundance between sex4 and Col-0, 136 behaved in the same way in lsf1 relative to Col-0 (Supplemental Table S3). Many of them have no assigned function (37.5%), but 14% encode known or putative transcription factors.
To discover whether differences in transcript abundance between lsf1 mutant and wild-type plants might be related to differences in sugar availability, we used three publicly available microarray data sets in which transcript levels in plants with high or low sugar levels were compared. These comparisons were between the following three sets of plants: wild-type plants and pgm mutant plants, which have very high sugar levels during the day and low levels at night; plants subjected in the light to either atmospheric levels of CO2 or low CO2 levels that bring about reduced levels of sugars; and plants either at the end of a normal night or after an extension of the night to deplete sugar levels (Gibon et al., 2004; Thimm et al., 2004; Blaݶsing et al., 2005). Of those transcripts that differed in abundance in the lsf1 versus wild-type data set, 49%, 67%, and 42% were also altered in abundance in the low CO2, pgm, and extended night data sets, respectively (Supplemental Table S4). In other words, 768 transcripts that differ in abundance between lsf1 and wild-type plants (76% of all such transcripts) are encoded by genes known to respond to sugar contents. For all of these transcripts, the change in the lsf1 mutant relative to Col-0 is in the opposite direction to the change brought about by reduced levels of sugars (shown as a heat map in Supplemental Table S4). In general, the differences between the lsf1 and wild-type transcriptomes are consistent with the idea that lsf1 plants contain more sugar than wild-type plants during the night. Although the changes in transcript abundance in the sex4 mutant were less extensive than in lsf1, a more complex picture emerges with respect to sugar responses. About 40% of the transcripts that differed in abundance between sex4 and wild-type plants are encoded by genes known to respond to sugar levels. Of these transcripts, most (74%) responded in the same way in the lsf1 mutant. Taking into account only those transcripts that differ statistically significantly in abundance between sex4 and Col-0 (Supplemental Table S1), 43% are both encoded by genes known to be responsive to sugar content and change in the same direction as in lsf1.
In an attempt to discover “lsf1-specific” changes in transcript abundance, we removed from the list of transcripts in Supplemental Table S2 all those encoded by genes known to respond to sugar content and all those also changed in abundance in the sex4 mutant. Of the remaining lsf1-specific transcripts, only four (in addition to LSF1 itself) differed statistically significantly in abundance between lsf1 and wild-type plants. These encoded a protein of unknown function (At3g49810), a putative receptor protein (Toll/Interleukin Receptor-Nucleotide Binding Site class; At1g72940), and two (chloroplast-encoded) ATPase subunits (atpA and atpF). About 170 further transcripts from Supplemental Table S2 were lsf1 specific but not statistically significantly different in abundance between lsf1 and wild-type plants. These were assigned to a wide range of MapMan bins, and no obvious trend was discernible (data not shown).
DISCUSSION
Our data provide unambiguous evidence that LSF1 is required for normal starch metabolism in Arabidopsis leaves. Further support for a primary role of LSF1 in starch degradation comes from our previous research on proteins involved in the degradation of leaf starch granules. We found that a soluble protein fraction from Arabidopsis leaves, purified on the basis of its capacity to degrade phosphorylated granules in vitro, contained LSF1 in addition to enzymes known to be directly involved in starch metabolism, including ISA3, BAM1, and the disproportionating enzyme DPE1 (Edner et al., 2007). The fraction was purified by ammonium sulfate precipitation and ion-exchange and amylose-affinity chromatography; it is currently not known whether the enzymes it contains copurify because of common properties or because they form multienzyme complexes.
Given the strong similarities in domain structure, predicted function, and subcellular location between the LSF1 and SEX4 proteins, they might be expected to have the same or similar functions in starch degradation. We discuss first the general similarities between the sex4 and lsf1 mutants that provide support for this idea and then the aspects in which the phenotypes differ. Finally, we consider whether LSF1, like SEX4, may be directly involved in the dephosphorylation of glucans at the surface of the starch granule during its degradation.
Similarities between the lsf1 and sex4 Mutant Phenotypes
lsf1 mutants had higher levels of starch than wild-type plants throughout the day and higher levels of hexoses during the light period. This phenotype is similar to that of the sex4 mutant but less extreme. sex4 plants contained twice as much starch as lsf1 plants at the end of the night and 50% more at the end of the day. Nevertheless, both mutants showed strong diurnal changes in starch content. In two batches of plants, the rates of synthesis and degradation were comparable with or slightly less than those of wild-type plants. The elevated starch content of the sex4 mutant is attributable to a slightly lower rate of starch degradation than of starch synthesis, leading to a gradual buildup of the level of starch as the leaves age (Zeeman et al., 1998; Zeeman and ap Rees, 1999). Our data suggest that this is also the case in the lsf1 mutant (Supplemental Fig. S3). The double mutant lsf1/sex4 has a higher starch content than either single mutant but the same diurnal pattern of change in starch and sugar levels as the single mutants.
The diurnal patterns of change in starch levels in the lsf1, sex4, and lsf1/sex4 mutants are similar to those of mutants lacking either of two chloroplastic β-amylases (BAM3 and BAM4; Fulton et al., 2008) or the debranching enzyme ISA3 (Wattebled et al., 2005; Delatte et al., 2006). In all of these mutants, rates of starch synthesis and degradation remain high even though the starch content of the leaf is elevated relative to that in wild-type leaves. By contrast, under our growth conditions, starch levels show little change during the diurnal cycle in sex1 mutants, deficient in GWD and hence in starch phosphorylation (Niittylaݶ et al., 2006). The actions of SEX4, the β-amylases, and ISA3 are probably closely linked: we have proposed that dephosphorylation of glucans by SEX4 at the granule surface during starch degradation in the chloroplast at night allows the complete hydrolysis of glucans to maltose and maltotriose by the concerted actions of β-amylases and ISA3 (Koݶtting et al., 2009). Evidence for this is provided by in vitro studies of the degradation of isolated, phosphorylated starch granules in which recombinant BAM3, ISA3, GWD, and SEX4 proteins acted synergistically to promote degradation (Edner et al., 2007; Koݶtting et al., 2009). The general phenotypic similarities between the lsf1 and sex4 mutants are consistent with the idea that LSF1, like SEX4, acts early in the process of starch degradation in conjunction with hydrolytic enzymes at the surface of the starch granule.
Microarray analysis revealed general similarities between the transcriptomes of sex4 and lsf1 mutants at the end of the night. Nearly 60% of the transcripts that differed in abundance between sex4 and wild-type plants also differed in abundance between lsf1 and wild-type plants. In both mutants, a substantial proportion of the transcripts that differed in abundance from those in wild-type plants are encoded by genes known to be responsive to sugar levels. The differences are consistent with higher sugar levels in lsf1 than in wild-type plants during the night, despite the fact that lsf1 is compromised in starch degradation.
We offer the following explanation of this phenomenon. Starch reserves in wild-type plants are almost exhausted at dawn. This carbon depletion coincides with increases late in the night in the expression of sugar-repressed genes and decreases in the expression of sugar-induced genes, indicating that sugar levels are correspondingly low (Smith and Stitt, 2007). In contrast, considerable amounts of starch are still present at dawn in both lsf1 and sex4 mutants, and the rate of degradation is apparently linear until the end of the night. Thus, sugar availability is likely to be higher in leaves of these mutants than in wild-type plants at the end of the night, leading to the observed differences in expression of sugar-responsive genes. Our measurements of sugar levels do not show large differences between wild-type, lsf1, and sex4 plants at the end of the night. However, these values are for whole-leaf extracts that include the contents of the phloem, apoplast, vacuole, and nonphotosynthetic cells as well as the cytosol of photosynthetic cells in which we assume that sugar sensing occurs. It is clear that the expression of many sugar-responsive genes is sensitive to small changes in cellular sugar status that are unlikely to be reflected in these measurements (Usadel et al., 2008).
Considerable further experimentation will be required to discover whether this explanation is correct. First, the transcriptome at the end of the night may reflect differences in perception of, and responses to, sugar levels throughout the night rather than simply at the end of the night. For example, in our experiments, sugar levels in the mutant plants fell more abruptly but then recovered more strongly than those in wild-type plants in the first half of the night: this difference may have consequences for the transcriptome later in the night. Second, the lsf1 mutant may sense or respond to sugars differently from wild-type plants, leading to transcriptional differences even when sugar levels are the same. Third, other aspects of the lsf1 phenotype may modulate the sugar responsiveness of the transcriptome. For example, it is possible that plants can sense and respond to starch levels as well as sugar availability. In lsf1, such an effect might counterbalance the low-sugar transcriptional response seen in wild-type plants at the end of the night.
The transcriptomes of the sex4 and lsf1 plants at the end of the night are very different from those of the starchless pgm mutant and the starch-excess sex1 mutant. Whereas transcripts for sugar-induced genes are elevated in lsf1 and sex4, they are reduced in pgm and sex1 mutants, and transcripts for sugar-repressed genes are elevated instead (Thimm et al., 2004; Smith and Stitt, 2007). In both pgm and sex1 mutants, the transcriptional changes probably reflect low carbon availability during the night. In pgm, no starch is available for the synthesis of sugars during the night. In sex1, loss of GWD results in a very low rate of starch degradation. Although GWD, SEX4, and LSF1 are all active at the granule surface at night, the loss of GWD has a much greater effect on the rate of starch degradation than the loss of SEX4 or LSF1, and this probably results in large differences in sugar availability at night between the sex1 mutant and the sex4 and lsf1 mutants. Such differences could explain the large differences between the sex1 transcriptome and those of sex4 and lsf1.
Differences between the lsf1 and sex4 Mutant Phenotypes
Three aspects of the sex4 phenotype are not seen in lsf1 mutants: the accumulation of phospho-oligosaccharides, a reduction in the amount of the chloroplastic α-amylase AMY3, and a radical alteration in the shape of starch granules. The accumulation of phospho-oligosaccharides is discussed below. The origins of the other two aspects of the phenotype, in which sex4 is epistatic to lsf1, are unclear. AMY3 is not essential for starch degradation in leaves of wild-type Arabidopsis (Yu et al., 2005), but it contributes to starch degradation in mutant backgrounds in which other starch-metabolizing enzymes are missing (Delatte et al., 2006; Streb et al., 2008). In the sex4 mutant, AMY3 makes a substantial contribution to starch degradation (Koݶtting et al., 2009). Our data indicate that the reduction in AMY3 protein and activity in the sex4 and lsf1/sex4 mutants is brought about by a posttranscriptional mechanism. This probably reflects the loss of a specific function of SEX4 that is not shared by LSF1, rather than a pleiotropic effect of reduced rates of starch accumulation. The levels and diurnal patterns of change of starch and sugars in the sex4 mutant are broadly similar to those in several other starch-excess mutants (including the lsf1, isa3, isa3/lda, bam3, bam4, and bam3/bam4 mutants), but in all of these other mutants, AMY3 protein levels are unaltered or increased (Fig. 4; Delatte et al., 2006; Fulton et al., 2008).
Starch granules in the sex4 and lsf1/sex4 mutants are larger and much more rounded in shape than the flattened, discoid granules of wild-type and lsf1 mutant plants. This alteration in shape is not a simple consequence of higher starch content: the starch granules of the sex1 mutant, which has a much higher starch content than sex4 or the double mutant, remain flattened rather than rounded (Zeeman et al., 2002). The reasons for these differences in granule morphology are not known. We cannot exclude the possibility that the difference in shape between lsf1 and sex4 granules simply reflects the milder starch-excess phenotype of the lsf1 mutant, rather than a fundamental difference in the roles of the LSF1 and SEX4 proteins in starch degradation.
Potentially, the most informative difference between the lsf1 and sex4 phenotypes is the large accumulation of phospho-oligosaccharides detected in sex4 and lsf1/sex4 but not in lsf1 leaves. This accumulation provides compelling evidence that SEX4 acts as a glucan phosphatase in vivo. Mutant analysis shows that the phospho-oligosaccharides are produced from starch, primarily by the action of AMY3 and ISA3 (Koݶtting et al., 2009). It seems likely that during starch degradation in wild-type plants, phosphorylation of glucan chains at the granule surface alters the packing of the chains and thus allows access to the degradative enzymes β-amylase and ISA3 (Edner et al., 2007; Hejazi et al., 2008). β-Amylase cannot act on phosphorylated parts of the chains, so dephosphorylation by SEX4 is required to allow complete conversion of chains to maltose and maltotriose. In the sex4 mutant, the phosphorylated chains cannot be completely degraded but many can still be released from the granule by AMY3 and ISA3, resulting in a reduced rate of granule degradation and a buildup of soluble phospho-oligosaccharides (Koݶtting et al., 2009). The absence of phospho-oligosaccharides from the lsf1 mutant plus the lack of an effect of the loss of LSF1 on the capacity of leaf extracts to release phosphate from isolated starch granules show that LSF1 does not participate in dephosphorylation of the same substrate as SEX4.
In summary, the locations of the SEX4 and LSF1 proteins, the phenotypes of the lsf1 and sex4 mutants, and the impact of the two mutations on the transcriptome are strikingly similar, suggesting that the two proteins participate in closely related processes at the start of the pathway of starch degradation. Some of the differences between the mutants may be quantitative rather than qualitative. However, we can rule out the possibility that LSF1 and SEX4 catalyze the same reaction and colimit this process during granule degradation or that they represent sequential steps on the pathway of degradation. First, loss of SEX4 dramatically alters the process of starch dephosphorylation in leaves, whereas loss of LSF1 has little effect on this process. Second, clear differences between the phenotype of the lsf1/sex4 mutant and those of the two single mutants show that the two proteins operate in parallel during starch degradation.
Possible Function of LSF1
Based on the observations above, we suggest two possible functions for LSF1. First, it may act as a glucan phosphatase but remove a different set of phosphate groups from those removed by SEX4. Second, it may act as a protein phosphatase, dephosphorylating and thus activating enzymes involved in starch degradation.
Superficially, our experiments would seem to rule out a significant role for LSF1 as a glucan phosphatase. We detected no accumulation of phospho-oligosaccharides in the lsf1 mutant. The capacity of extracts of lsf1 mutant plants to remove phosphate groups from starch phosphorylated by GWD was the same as that of extracts of wild-type plants, whereas this capacity was considerably reduced in extracts of sex4 mutant plants. However, both of these sorts of experiments might fail to reveal a glucan phosphatase activity of LSF1, for the following reasons.
We suggested previously that SEX4 normally acts on phosphorylated chains at the granule surface, which can be released from the granule (as soluble phospho-oligosaccharides) by the actions of AMY3 and ISA3 when SEX4 is absent (Koݶtting et al., 2009). It is possible that LSF1 acts on a different set of phosphorylated chains, which cannot be released from the granule as phospho-oligosaccharides in the lsf1 mutant. The phenotype of the lsf1/sex4 double mutant is arguably consistent with this idea. The double mutant has a higher starch content than either sex4 or lsf1 and a lower accumulation of phospho-oligosaccharides during the night (Supplemental Fig. S5). This phenotype can be explained if LSF1 facilitates the actions of starch-degrading enzymes, including ISA3 and AMY3, in the sex4 mutant by dephosphorylating a subset of phosphorylated chains at the granule surface. In the double mutant, the loss of this activity would inhibit the actions of starch-degrading enzymes on the granule surface, thus reducing the rate of starch degradation and the buildup of phospho-oligosaccharides.
We investigated the possibility that LSF1 specifically removes phosphate groups from the three-position of glucosyl residues. Although only about 20% of phosphate groups are in this position, molecular modeling studies indicate that they are more disruptive of the packing of double helices of amylopectin chains than phosphate groups on the six-position (Blennow et al., 2002; Hansen et al., 2009); hence, they may have a disproportionate effect on the accessibility of the granule surface for starch-degrading enzymes. However, we found no difference in the ratio of Glc 6-P to Glc 3-P residues in amylopectin from wild-type and lsf1 leaves. Thus, while we cannot rule out the possibility that LSF1 can remove phosphate from the three-position of glucosyl residues in vivo, it seems unlikely that this could explain why the protein is required for normal rates of starch degradation.
LSF1 and SEX4 belong to a group of proteins predicted to be dual-specificity protein phosphatases (Pfam DSPc PF00782). Although some of these proteins dephosphorylate proteins in vivo, others do not. SEX4 and the related animal glycogen phosphatase laforin (Gentry et al., 2007) are examples with nonprotein substrates; others include the lipid phosphatase PTEN (phosphatase and tensin homolog) that dephosphorylates phosphatidylinositol-3,4,5-triphosphate and thus acts as a suppressor of the phosphoinositide 3-kinase signaling pathway implicated in several mammalian cancers (Maehama and Dixon, 1998). At present, sequence inspection does not allow the substrate specificity of these enzymes to be predicted. We cannot rule out the possibility that LSF1 dephosphorylates a protein involved in starch degradation, thereby modulating its activity. The fact that LSF1 is absent from starch-synthesizing green algae implies that it is not necessary per se for the efficient degradation of starch but may have a specific function in land plants. Apart from LSF1, most starch-synthesizing green algae contain all of the classes of enzymes shown to be necessary for normal rates of starch synthesis and degradation in Arabidopsis leaves, including GWD1 and SEX4 (Deschamps et al., 2008).
MATERIALS AND METHODS
Plant Materials and Growth Conditions
Arabidopsis (Arabidopsis thaliana) plants were grown in compost in a controlled-environment cabinet at 20°C in a 12-h-light/12-h-dark cycle at 60% to 75% relative humidity and harvested at 3 to 4 weeks old unless otherwise stated. Light intensity was 150 to 200 μmol quanta m™2 s™1 unless otherwise stated.
Genotyping of lsf1-1 and lsf1-2
Identification of homozygous mutant plants (SALK_100036 and SALK_053285) was done by PCR-based screening using a T-DNA left border-specific primer, 5′-TGGTTCACGTAGTGGGCCAT-3′, and the LSF1-specific primers 5′-AGTAAGAGGAGCTCGCCGAC-3′ and 5′-TTCGAGAGCTCCTAAACCGG-3′.
RNA Isolation and Reverse Transcription-PCR
Total RNA was extracted from leaves using the Purescript kit according to the manufacturer's instructions (www1.qiagen.com). For reverse transcription (RT)-PCR, 1 μg of total RNA was treated with DNase I (www.invitrogen.com) and reverse transcription was done on 0.2 μg of total RNA using the Reverse-iT first-strand synthesis kit (www.abgene.com). PCR was performed on 1 μL of cDNA using the following gene-specific primers: LSF1, 5′-CCGGTTTAGGAGCTCTCGAA-3′ and 5′-CGCCAGCCTAAATCTTTCGC-3′; and TUBULIN, 5′-CCTGATAACTTCGTCTTTGG-3′ and 5′-GTGAACTCCATCTCGTCCAT-3′ as an internal control.
Extraction and Assay of Metabolites
Four-week-old rosettes were harvested and immediately frozen in liquid nitrogen. Starch and sugars were extracted and assayed as previously (Hargreaves and ap Rees, 1988; Critchley et al., 2001). Phospho-oligosaccharides were assayed according to Koݶtting et al. (2009) on the extracts used for sugar measurements. Extracts were treated first with Antarctic Phosphatase buffer (New England Biolabs) with or without (mock) Antarctic Phosphatase, then with Dowex 50W and Dowex 1 ion-exchange resins. Samples were separated by high-performance anion-exchange chromatography-pulsed-amperometric detection (HPAEC-PAD) on a CarboPac PA20 column (Dionex). Phospho-oligosaccharide content was calculated as the difference between samples treated with Antarctic Phosphatase and mock-treated samples.
Production and Use of LSF1 Antisera
Two antisera were prepared. For immunoblots shown in Supplemental Figures S2 and S3, a clone encoding full-length LSF1 with a C-terminal 6xHis tag was introduced into expression vector p0GWA (Busso et al., 2005) and expressed in Escherichia coli Rosetta cells. The recombinant protein was insoluble: it was purified from inclusion bodies by repeated centrifugation and resuspension in 50 mm Tris-Cl, pH 7.5, 1 mm EDTA, 100 mm NaCl, 1% (v/v) Triton X-100, and 2 m urea, solubilization in 1.5× SDS sample buffer (100°C, 10 min), preparative SDS-PAGE, and excision of the appropriate band. Antiserum was raised by Eurogentec (www.eurogentec.com). For other immunoblots, a rabbit was immunized with a synthetic peptide predicted from the sequence of the LSF1 gene: 5′-KATHKGGPRFETEV-3′. This work was done by Sigma-Genosys (www.sigmaaldrich.com). Extraction of leaves, SDS-PAGE, and immunoblotting were as described by Chia et al. (2004).
Release of 33P from Prelabeled Starch Granules
Experiments were performed according to Koݶtting et al. (2009). Purified starch granules from sex1 mutant plants were 33P phosphorylated using recombinant GWD and [β-33P]ATP. Soluble extracts of leaf proteins were desalted and incubated with 33P-labeled starch granules for 25 min at 25°C, and release of soluble 33P was measured.
Determination of Starch Phosphate Content
Measurement of total phosphate content was according to Koݶtting et al. (2009). Starch was hydrolyzed in 1 m HCl for 2 h at 95°C, neutralized, and incubated with Antarctic Phosphatase. Orthophosphate was determined with Malachite Green. For measurement of Glc 3-P and Glc 6-P, 5-mg samples of starch were hydrolyzed in 150 μL of 0.7 m HCl at 95°C for 3 h. Samples were neutralized with an equal volume of 0.7 m NaOH and dried. Pellets were resuspended in 100 μL of water, and 25-μL samples were analyzed by HPAEC-PAD using a Dionex ICS-3000 HPLC system with a PA1 column. Elution was with a linear Na-acetate gradient (0–150 mm in 30 min) in 100 mm NaOH and a flow rate of 1 mL min™1. Glc 6-P and Glc 3-P (Ritte et al., 2002) were used as internal standards.
Starch Extraction and Structure Analysis
Extraction of starch for electron microscopy and determination of chain lengths of starch polymers were according to Streb et al. (2008).
AMY3 Protein and Activity Measurements
These methods were as described by Yu et al. (2005).
Infiltration of Nicotiana benthamiana Leaves with Agrobacterium tumefaciens
The coding region of At3g01510 was amplified by RT-PCR using primers 5′-ATGGCGTTTCTTCAACAAATCTCC-3′ and 5′-CGACTTTGGGCATAGTCTTATTGGAC-3′ and cloned into pCR8/GW/TOPO (Invitrogen). The insert was transferred into pK7FWG2,0 (Karimi et al., 2002) in a reaction mediated by Gateway LR Clonase Enzyme Mix (Invitrogen). The pK7FWG2,0 vector encodes an N-terminal enhanced GFP driven by the cauliflower mosaic virus 35S promoter. The plasmid was transformed into Agrobacterium (strain GV301), and transformants were selected with 100 μg mL™1 spectinomycin. N. benthamiana leaves were infiltrated with the transformed Agrobacterium mixed with Agrobacterium containing the gene-silencing suppressor p19 (Voinnet et al., 2003). Three days after transformation, GFP fluorescence was monitored by confocal microscopy.
Microarray Analysis
Plants were grown as above, with a light intensity of 100 μmol quanta m™2 s™1. For each biological replicate, three mature leaves from each of eight 4-week-old plants were pooled to provide a single sample for RNA purification. Harvesting was 0.5 h before the end of the dark period. Three independent biological replicates were performed: for each replication, all three genotypes were grown and harvested at the same time. The array experiments were carried out using the ATH1 Arabidopsis GeneChip microarray at the Nottingham Arabidopsis Stock Centre (NASC) using Affymetrix-recommended protocols. The microarray data are available for public access on the NASC Web site (http://Arabidopsis.info/; accession no. NASCARRAYS-411). Arrays were quantile normalized using the Robust Multichip Analysis method in the R BioConductor version 1.7 package affy (Gentleman et al., 2004). The full normalized data set, and the further data sets used in our analyses, are provided as Supplemental Table S5. Differentially expressed genes were identified using the Rank Products of Breitling et al. (2004) at a false discovery rate of <0.05.
Supplemental Data
The following materials are available in the online version of this article.
Supplemental Figure S1. Phylogenetic analysis of LSF1.
Supplemental Figure S2. Comparison of wild-type and lsf1-1, sex4-3, and lsf1-1/sex4-3 plants.
Supplemental Figure S3. Changes in starch content of wild-type and lsf1 rosettes following destarching.
Supplemental Figure S4. Nature and consequences of the mutation in Ke103 (lsf1-2).
Supplemental Figure S5. Complementation of the lsf1-1 mutant.
Supplemental Figure S6. Differences in the chain length distributions of starch polymers from leaves of wild-type and mutant plants.
Supplemental Figure S7. Diurnal changes in phospho-oligosaccharide content in sex4 and lsf1/sex4 mutant plants.
Supplemental Figure S8. Assigned functions for transcripts that differ in abundance between wild-type and lsf1-1 mutant plants (A) and between wild-type and sex4-3 mutant plants (B).
Supplemental Table S1. Statistical analysis of differences in transcript abundance.
Supplemental Table S2. Differences in transcript abundance greater than 1.5-fold.
Supplemental Table S3. Changes in transcript abundance common to both mutants.
Supplemental Table S4. Changes in transcript abundance of sugar-sensitive genes.
Supplemental Table S5. Normalized microarray data used in this study.
ACKNOWLEDGMENTS
We thank Simona Eicke (Eidgenoݶssische Technische Hochschule Zurich) and Liz Hughes (John Innes Centre) for excellent technical assistance, Peter Waݶgli (Electron Microscopy Center, Eidgenoݶssische Technische Hochschule Zurich) for scanning electron microscopy images, and Andreas Magusin (John Innes Centre) for help with the microarray data analysis.
LITERATURE CITED
Baunsgaard L, Luݶtken H, Mikkelsen R, Glaring MA, Pham TT, Blennow A (
Blaݶsing OE, Gibon Y, Guݶnther M, Hoݶhne M, Morcuende R, Osuna D, Thimm O, Usadel B, Scheible W-R, Stitt M (
Blennow A, Engelsen SB, Nielsen TH, Baunsgaard L, Mikkelsen R (
Breitling R, Armengaud P, Amtmann A, Herzyk P (
Busso D, Delagoutte-Busso B, Moras D (
Chia T, Thorneycroft D, Chapple A, Messerli G, Chen J, Zeeman SC, Smith SM, Smith AM (
Christiansen C, Hachem NA, Glaring MA, Viksø-Nielsen A, Sigurskjold BW, Svensson B, Blennow A (
Critchley JH, Zeeman SC, Takaha T, Smith AM, Smith SM (
Delatte T, Umhang M, Trevisan M, Eicke S, Thorneycroft D, Smith SM, Zeeman SC (
Deschamps P, Moreau H, Worden AZ, Dauvilleݩe D, Ball SG (
Edner C, Li J, Albrecht T, Mahlow S, Hejazi M, Hussain H, Kaplan F, Guy C, Smith S, Steup M, et al (
Fordham-Skelton AP, Chilley P, Lumbreras V, Reignoux S, Fenton TR, Dahm CC, Pages M, Gatehouse JA (
Fulton DC, Stettler M, Mettler T, Vaughan CK, Li J, Francisco P, Gil M, Reinhold H, Eicke S, Messerli G, et al (
Gentleman RC, Carey VJ, Bates DM, Bolstad B, Dettling M, Dudoit S, Ellis B, Gautier L, Ge Y, Gentry J, et al (
Gentry MS, Dowen RH III, Worby CA, Mattoo S, Ecker JR, Dixon JE (
Gibon Y, Blaݶsing OE, Palacios-Rojas N, Pankovic D, Hendriks JH, Fisahn J, Hoݶhne M, Guݶnther M, Stitt M (
Haebel S, Hejazi M, Frohberg C, Heydenreich M, Ritte G (
Hansen PI, Spraul M, Dvortsak P, Larsen FH, Blennow A, Motawia MS, Engelsen SB (
Hargreaves JA, ap Rees T (
Hejazi M, Fettke J, Haebel S, Edner C, Paris O, Frohberg C, Steup M, Ritte G (
Karimi M, Inze D, Depicker A (
Kerk D, Conley TR, Rodriguez FA, Tran HT, Nimick M, Muench DG, Moorhead GBG (
Koݶtting O, Pusch K, Tiessen A, Geigenberger P, Steup M, Ritte G (
Koݶtting O, Santelia D, Edner C, Eicke S, Marthaler T, Gentry MS, Comparot-Moss S, Chen J, Smith AM, Steup M, et al (
Maehama T, Dixon JE (
Niittylaݶ T, Comparot-Moss S, Lue WL, Messerli G, Trevisan M, Seymour MD, Gatehouse JA, Villadsen D, Smith SM, Chen J, et al (
Ritte G, Heydenreich M, Mahlow S, Haebel S, Koݶtting O, Steup M (
Ritte G, Lloyd JR, Eckermann N, Rottmann A, Kossmann J, Steup M (
Smith AM, Stitt M (
Smith SM, Fulton DC, Chia T, Thorneycroft D, Chapple A, Dunstan H, Hylton C, Zeeman SC, Smith AM (
Sokolov L, Dominguez-Solis JR, Allary AL, Buchanan BB, Luan S (
Stitt M, Gibon Y, Lunn JE, Piques M (
Streb S, Delatte T, Umhang M, Eicke S, Schorderet M, Reinhardt D, Zeeman SC (
Thimm O, Blaݶsing OE, Gibon Y, Nagel A, Meyer S, Kruger P, Selbig J, Muݶller LA, Rhee SY, Stitt M (
Usadel B, Blaݶsing OE, Gibon Y, Retzlaff K, Hoݶhne M, Guݶnther M, Stitt M (
Voinnet O, Rivas S, Mestre P, Baulcombe D (
Wattebled F, Dong Y, Dumez S, Devalleݩ D, Planchot V, Berbezy P, Vyas D, Colonna P, Chatterjee M, Ball S, et al (
Yu TS, Kofler H, Haݶusler RE, Hille D, Fluݶgge UI, Zeeman SC, Smith AM, Kossmann J, Lloyd J, Ritte G, et al (
Yu TS, Zeeman SC, Thorneycroft D, Fulton DC, Dunstan H, Lue WL, Hegemanm B, Tung SY, Umemoto T, Chapple A, et al (
Zeeman SC, ap Rees T (
Zeeman SC, Northrop F, Smith AM, ap Rees T (
Zeeman SC, Tiessen A, Pilling E, Kato KL, Donald AM, Smith AM (
Author notes
This work was supported by the Biotechnology and Biological Sciences Research Council (core strategic grant and grant nos. BBS/B/11877 and BB/C513542/1 to the John Innes Centre), by the Swiss National Science Foundation (via the National Centre for Competence in Research-Plant Survival and the Swiss-South African Joint Research Programme grant no. 08 IZ LS Z3122916), by Eidgenoݶssische Technische Hochschule Zurich, and by the Gatsby Charitable Trust (to D.M.).
Present address: University of Exeter School of Biosciences, Geoffrey Pope Building, Stocker Road, Exeter EX4 4QD, UK.
Present address: Metanomics GmbH, Tegeler Weg 33, 10589 Berlin, Germany.
Corresponding author; e-mail [email protected].
The author responsible for distribution of materials integral to the findings presented in this article in accordance with the policy described in the Instructions for Authors (www.plantphysiol.org) is: Alison M. Smith ([email protected]).
The online version of this article contains Web-only data.
Open Access articles can be viewed online without a subscription.