-
PDF
- Split View
-
Views
-
Cite
Cite
Chong Wei Jin, Shao Ting Du, Wei Wei Chen, Gui Xin Li, Yong Song Zhang, Shao Jian Zheng, Elevated Carbon Dioxide Improves Plant Iron Nutrition through Enhancing the Iron-Deficiency-Induced Responses under Iron-Limited Conditions in Tomato, Plant Physiology, Volume 150, Issue 1, May 2009, Pages 272–280, https://doi.org/10.1104/pp.109.136721
- Share Icon Share
Abstract
The increases in atmospheric carbon dioxide (CO2) concentrations can enhance plant growth and change their nutrient demands. We report that when tomato (Lycopersicon esculentum ‘Zheza 809’) plants were grown in iron (Fe)-limited medium (with hydrous ferric iron oxide) and elevated CO2 (800 μL L−1), their biomass and root-to-shoot ratio were greater than plants grown in ambient CO2 (350 μL L−1). Furthermore, the associated increase in Fe concentrations in the shoots and roots alleviated Fe-deficiency-induced chlorosis. Despite the improved nutrient status of plants grown in Fe-limited medium under elevated CO2, the Fe-deficiency-induced responses in roots, including ferric chelate reductase activity, proton secretion, subapical root hair development, and the expression of FER, FRO1, and IRT genes, were all greater than plants grown in the ambient CO2. The biomass of plants grown in Fe-sufficient medium was also increased by the elevated CO2 treatment, but changes in tissue Fe concentrations and Fe deficiency responses were not observed. These results suggest that the improved Fe nutrition and induction of Fe-deficient-induced responses in plants grown in Fe-limited medium under elevated CO2 are caused by interactions between elevated CO2 and Fe deprivation. Elevated CO2 also increased the nitric oxide (NO) levels in roots, but treatment with the NO scavenger cPTIO inhibited ferric chelate reductase activity and prevented the accumulation of LeFRO1, LeIRT1, and FER transcripts in roots of the Fe-limited plants. These results implicate some involvement of NO in enhancing Fe-deficiency-induced responses when Fe limitation and elevated CO2 occur together. We propose that the combination of elevated CO2 and Fe limitation induces morphological, physiological, and molecular responses that enhance the capacity for plants to access and utilize Fe from sparingly soluble sources, such as Fe(III)-oxide.
Carbon dioxide (CO2) is one of the most important greenhouse gases contributing to global warming (Intergovernmental Panel on Climate Change, 2001). Human activity has increased the concentration of atmospheric CO2 from about 280 μL L−1 at the beginning of the nineteenth century to 367 μL L−1 at the end of the twentieth century (Bolin and Kheshgi, 2001), and the concentration is estimated to reach 490 to 1,260 μL L−1 by 2100 (Intergovernmental Panel on Climate Change, 2001). Increases in CO2 concentration will likely have a profound impact on plant growth. Previous studies have shown that elevated CO2 increases net photosynthesis rate in C3 plants because higher CO2 suppresses ribulose-1,5-bisphosphate oxygenase activity, decreases photorespiration, and increases carbon assimilates for plant growth and development (Lawlor and Mitchell, 2000). As a consequence, elevated CO2 treatments generally increase the biomass of C3 plants (Dijkstra et al., 2002). For example, Kimball and Mauney (1993) demonstrated that cotton plants (Gossypium hirsutum) grown under 550 μL L−1 CO2 had a 35% higher biomass, 40% higher fruit weight, and 60% higher lint yield than plants grown under 350 μL L−1 CO2.
The enhancement of plant growth by elevated CO2 will also increase their demand for nutrients. For example, the growth response of Japanese red pine (Pinus densiflora) seedlings to phosphate (Pi) was saturated at 0.1 mm Pi in ambient CO2 (350 μL L−1), whereas in the elevated CO2 (700 μL L−1), the growth response to Pi supply did not saturate even at 0.2 mm Pi supply (Kogawara et al., 2006). Iron (Fe) is an essential micronutrient for plant growth and development. Although the total Fe content in soil regularly exceeds plant requirements, its bioavailability to plants is often limited (Guerinot and Yi, 1994), particularly in calcareous soils, which represent 30% of the earth's surface (Imsande, 1998). Therefore, Fe nutrition in plants is likely to be affected by the continued elevation of atmospheric CO2, which, in turn, will affect crop production. Sasaki et al. (1998) demonstrated that both ferric reductase activity and Fe uptake capacity of the marine alga Chlorococcum littorale cultured in Fe-limited medium were significantly enhanced by extremely high concentrations of CO2. However, little is known about how elevated CO2 influences Fe nutrition in higher plants, which undergo specific morphological and physiological changes in response to Fe deficiency (Marschner, 1995). Römheld and Marschner (1986) classified these changes as strategy I and strategy II mechanisms. Strategy I occurs in nongraminaceous monocots and dicots, and strategy II occurs in graminaceous monocots. For strategy I plants, Fe deficiency enhances the activity of the plasmalemma NADPH-ferric chelate reductase (FCR; Robinson et al., 1999; Yi and Guerinot, 1996), enhances the expression of plasmalemma Fe(II) transporters (Eide et al., 1996; Vert et al., 2002), increases acidification of the extracellular medium, and increases the subapical development of root hairs.
We investigated how elevated CO2 affects the Fe status of the strategy I species tomato (Lycopersicon esculentum) grown with a soluble Fe source or the sparingly soluble hydrous Fe(III)-oxide. We demonstrate that elevated CO2 can improve plant Fe nutrition under Fe-limited conditions by inducing morphological, physiological, and molecular changes that enhance Fe uptake.
RESULTS
Effect of Elevated CO2 on Chlorophyll Synthesis, Plant Growth, and Uptake of Fe
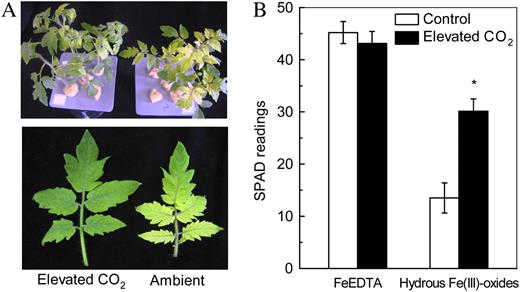
Effects of elevated CO2 treatment on chlorophyll synthesis. A, Tomatoes grown in hydrous Fe(III)-oxide medium. B, SPAD readings of tomatoes grown in FeEDTA or hydrous Fe(III)-oxide medium. Tomatoes were grown under ambient (350 μL L−1) or elevated (800 μL L−1) CO2. The image was taken on the seventh day of treatment, and the SPAD readings were recorded. Data are means ± sd (n = 8). *, Significant differences (P < 0.05) between ambient and elevated CO2 treatments.
Plant growth was increased by elevated CO2 in both Fe-sufficient and Fe-limited media. Shoot fresh weight was increased by 22% and 44%, respectively, and root fresh weight by 43% and 97%, respectively, compared with plants grown in ambient CO2 (Table I
Effects of elevated CO2 treatment on biomass, root-to-shoot ratio, and Fe concentration of tomatoes grown in FeEDTA or hydrous Fe(III)-oxide medium
Tomatoes were grown under ambient (350 μL L−1) or elevated (800 μL L−1) CO2. On the seventh day of treatment, biomass and Fe concentration were analyzed. Data are means ± sd (n = 5). *, Significant differences (P < 0.05) between ambient and elevated CO2 treatments. DW, Dry weight.
Fe Source . | Treatment . | Fresh Biomass . | . | Root-to-Shoot Ratio . | Fe Concentration . | . | ||
---|---|---|---|---|---|---|---|---|
. | . | Shoot . | Root . | . | Shoot . | Root . | ||
g plant−1 | μg g−1 DW | |||||||
FeEDTA | Ambient | 3.354 ± 0.242 | 0.442 ± 0.073 | 0.132 ± 0.014 | 106.07 ± 9.40 | 338.28 ± 35.95 | ||
Elevated CO2 | 4.078 ± 0.309 * | 0.631 ± 0.079 * | 0.155 ± 0.021 | 107.46 ± 4.24 | 327.42 ± 31.05 | |||
Hydrous Fe(III)-oxide | Ambient | 2.461 ± 0.431 | 0.351 ± 0.059 | 0.143 ± 0.024 | 58.74 ± 2.07 | 101.81 ± 12.23 | ||
Elevated CO2 | 3.539 ± 0.458 * | 0.690 ± 0.101 * | 0.194 ± 0.013 * | 77.36 ± 5.25 * | 156.42 ± 15.27 * |
Fe Source . | Treatment . | Fresh Biomass . | . | Root-to-Shoot Ratio . | Fe Concentration . | . | ||
---|---|---|---|---|---|---|---|---|
. | . | Shoot . | Root . | . | Shoot . | Root . | ||
g plant−1 | μg g−1 DW | |||||||
FeEDTA | Ambient | 3.354 ± 0.242 | 0.442 ± 0.073 | 0.132 ± 0.014 | 106.07 ± 9.40 | 338.28 ± 35.95 | ||
Elevated CO2 | 4.078 ± 0.309 * | 0.631 ± 0.079 * | 0.155 ± 0.021 | 107.46 ± 4.24 | 327.42 ± 31.05 | |||
Hydrous Fe(III)-oxide | Ambient | 2.461 ± 0.431 | 0.351 ± 0.059 | 0.143 ± 0.024 | 58.74 ± 2.07 | 101.81 ± 12.23 | ||
Elevated CO2 | 3.539 ± 0.458 * | 0.690 ± 0.101 * | 0.194 ± 0.013 * | 77.36 ± 5.25 * | 156.42 ± 15.27 * |
Effects of elevated CO2 treatment on biomass, root-to-shoot ratio, and Fe concentration of tomatoes grown in FeEDTA or hydrous Fe(III)-oxide medium
Tomatoes were grown under ambient (350 μL L−1) or elevated (800 μL L−1) CO2. On the seventh day of treatment, biomass and Fe concentration were analyzed. Data are means ± sd (n = 5). *, Significant differences (P < 0.05) between ambient and elevated CO2 treatments. DW, Dry weight.
Fe Source . | Treatment . | Fresh Biomass . | . | Root-to-Shoot Ratio . | Fe Concentration . | . | ||
---|---|---|---|---|---|---|---|---|
. | . | Shoot . | Root . | . | Shoot . | Root . | ||
g plant−1 | μg g−1 DW | |||||||
FeEDTA | Ambient | 3.354 ± 0.242 | 0.442 ± 0.073 | 0.132 ± 0.014 | 106.07 ± 9.40 | 338.28 ± 35.95 | ||
Elevated CO2 | 4.078 ± 0.309 * | 0.631 ± 0.079 * | 0.155 ± 0.021 | 107.46 ± 4.24 | 327.42 ± 31.05 | |||
Hydrous Fe(III)-oxide | Ambient | 2.461 ± 0.431 | 0.351 ± 0.059 | 0.143 ± 0.024 | 58.74 ± 2.07 | 101.81 ± 12.23 | ||
Elevated CO2 | 3.539 ± 0.458 * | 0.690 ± 0.101 * | 0.194 ± 0.013 * | 77.36 ± 5.25 * | 156.42 ± 15.27 * |
Fe Source . | Treatment . | Fresh Biomass . | . | Root-to-Shoot Ratio . | Fe Concentration . | . | ||
---|---|---|---|---|---|---|---|---|
. | . | Shoot . | Root . | . | Shoot . | Root . | ||
g plant−1 | μg g−1 DW | |||||||
FeEDTA | Ambient | 3.354 ± 0.242 | 0.442 ± 0.073 | 0.132 ± 0.014 | 106.07 ± 9.40 | 338.28 ± 35.95 | ||
Elevated CO2 | 4.078 ± 0.309 * | 0.631 ± 0.079 * | 0.155 ± 0.021 | 107.46 ± 4.24 | 327.42 ± 31.05 | |||
Hydrous Fe(III)-oxide | Ambient | 2.461 ± 0.431 | 0.351 ± 0.059 | 0.143 ± 0.024 | 58.74 ± 2.07 | 101.81 ± 12.23 | ||
Elevated CO2 | 3.539 ± 0.458 * | 0.690 ± 0.101 * | 0.194 ± 0.013 * | 77.36 ± 5.25 * | 156.42 ± 15.27 * |
Effect of Elevated CO2 on Fe-Deficiency-Induced Physiological and Morphological Responses
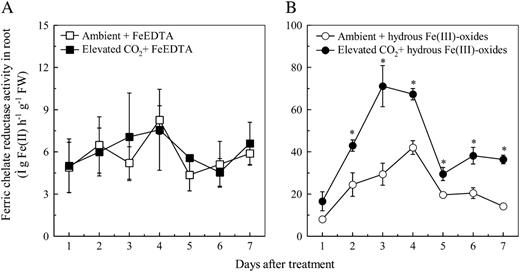
Effects of elevated CO2 treatment on FCR activity of roots from the plants grown in FeEDTA (A) or hydrous Fe(III)-oxide (B) medium. Treatments are the same as in Figure 1. Data are means ± sd (n = 4). *, Significant differences (P < 0.05) between ambient and elevated CO2 treatments at each time point. FW, Fresh weight.
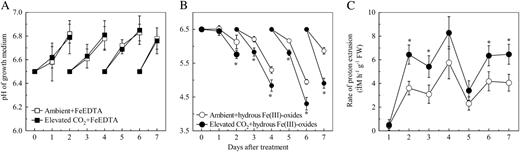
Effects of elevated CO2 treatment on proton extrusion of roots. A, pH of the FeEDTA growth medium. B, pH of hydrous Fe(III)-oxide growth medium. C, Root proton extrusion rate of the plants grown in hydrous Fe(III)-oxide medium. Treatments are the same as in Figure 1 and measurement of the proton extrusion rate was conducted as described in “Materials and Methods.” Data are means ± sd (n = 4 for pH data; n = 8 for proton extrusion rate). *, Significant differences (P < 0.05) between ambient and elevated CO2 treatments at each time point. FW, Fresh weight.
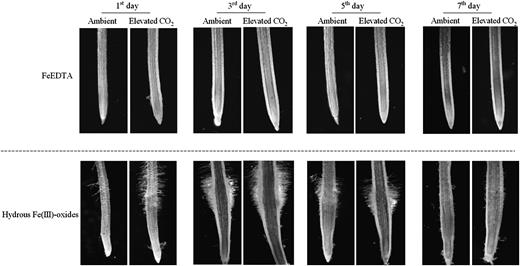
Effects of elevated CO2 treatment on subapical root hair development of the plant grown in FeEDTA (A) or hydrous Fe(III)-oxide (B) medium. Treatments are the same as in Figure 1.
Effect of Elevated CO2 on the Expression of Genes Involved in Fe Uptake
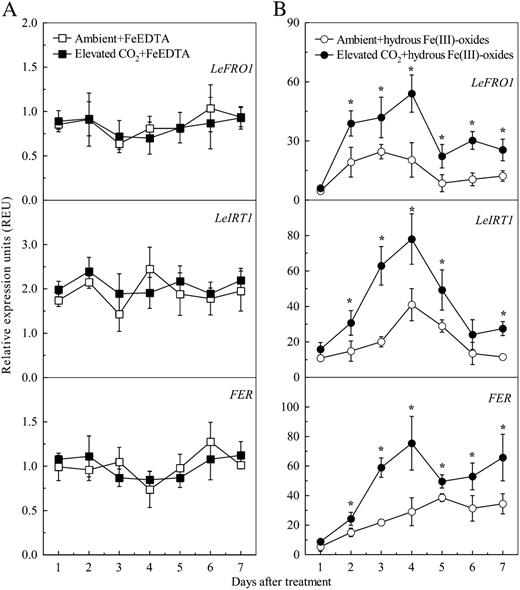
Effects of elevated CO2 treatment on expression levels of FER, LeFRO1, and LeIRT1 in tomato roots cultured with FeEDTA (A) or hydrous Fe(III)-oxide (B) medium. Relative expression levels were calculated and normalized with respect to α-tubulin mRNA. Treatments are the same as in Figure 1. Data are means ± sd (n = 5). *, Significant differences (P < 0.05) between ambient and elevated CO2 treatments at each time point.
The basic helix-loop-helix (bHLH) protein FER regulates the expression of LeFRO1 and LeIRT1 genes, as well as other responses to Fe deficiency in tomato roots (Ling et al., 2002; Bereczky et al., 2003; Li et al., 2004). The expression of the FER gene in roots of plants grown in Fe-limited medium was significantly induced as well, and this change was even greater after 2 d in elevated CO2 (Fig. 5B). However, the elevated CO2 did not affect the FER expression in roots of Fe-sufficient plants (Fig. 5A).
Possible Role of Nitric Oxide in the Regulation of the Enhanced Plant Responses to Fe Deficiency under Elevated CO2
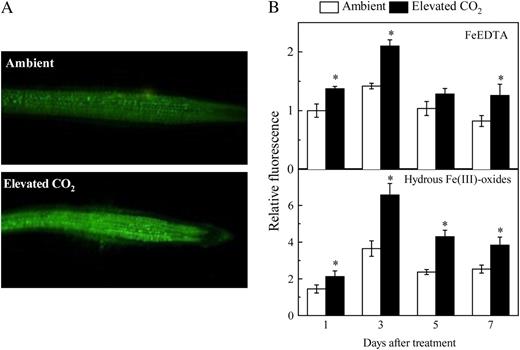
Effect of elevated CO2 treatment on NO production in roots. A, Photographs of NO production shown as green fluorescence in representative roots after 3 d of growth in hydrous Fe(III)-oxide medium. B, Time course of NO production expressed as relative fluorescence. Treatments are the same as in Figure 1. NO production was visualized with DAF-FM DA dye. Photographs were analyzed with Photoshop software (Adobe Systems) and fluorescence intensity was estimated by measuring the average pixel intensity. Data are means ± sd (n = 20). *, Significant differences (P < 0.05) between ambient and elevated CO2 treatments at each time point.
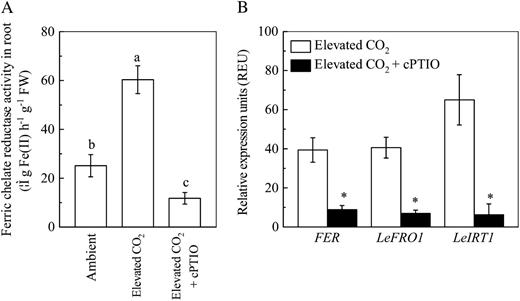
Role of NO in regulating the stimulative effect of elevated CO2 treatment on plant Fe uptake. A, Effects of cPTIO treatment on root FCR activity. FW, Fresh weight. B, Effects of cPTIO treatment on expression levels of LeFRO1, LeIRT1, and FER in tomato roots. After 2 d of growth under elevated CO2, plants were subjected to 200 μm cPTIO treatment for 24 h, and then the FCR activity and expression of genes were analyzed. Relative expression levels were calculated and normalized with respect to α-tubulin mRNA. Data are means ± sd (n = 5). Different letters or asterisks indicate significant differences (P < 0.05) among the treatments.
DISCUSSION
Kläring et al. (2007) proposed that plant growth is stimulated by CO2 concentrations up to 800 to 1,000 μL L−1, suggesting that the current ambient CO2 concentration of approximately 350 μL L−1 is suboptimal. Thus, plant growth is likely to increase as the CO2 concentration reaches the 490 to 1,260 μL L−1 levels predicted to occur by 2100 (Intergovernmental Panel on Climate Change, 2001). Previous studies have examined how the CO2-dependent stimulation in growth is likely to enhance the requirements for macronutrients like nitrogen (N) and phosphorus (Baxter et al., 1994; Kogawara et al., 2006), but none have considered the changing demand for micronutrients. Although this extra demand for nutrients may be partially compensated by increasing nutrient-use efficiency (Conroy et al., 1992; Newbery et al., 1995), the stimulation in growth associated with elevated CO2 is unlikely to be sustained without a concomitant increase in nutrient supply. In other words, either more fertilizer will need to be applied to avoid nutrient deficiencies or plants will have to become more efficient at acquiring those nutrients from the soil. Furthermore, because Fe deficiency is a major nutritional disorder in humans, affecting an estimated 2 billion people (Baynes and Bothwell, 1990), the Fe status of food crops will be increasingly important in the future. In this study, we found that elevated CO2 not only increased the biomass accumulation of plants cultured in the Fe-limited medium, but also significantly improved their Fe status.
Elevated CO2 Favors Plant Growth under Fe-Limited Condition
The increased biomass accumulation of plants grown in elevated CO2 is largely attributed to the increase of net photosynthesis, but nutrient limitation has generally been found to suppress this response (Conroy 1992; McKee and Woodward 1994; Lloyd and Farquhar 1996; Stitt and Krapp, 1999). For examples, when birch (Betula pendula; Pettersson et al., 1993; Silvola and Ahlholm, 1995), loblolly pine (Pinus taeda; Gebauer et al., 1996), rice (Oryza sativa; Ziska et al., 1996), cotton (Rogers et al., 1993), wheat (Triticum aestivum; G.S. Rogers et al., 1996), and tobacco (Nicotiana tabacum; Geiger et al., 1999) were grown at various N supplies, elevated CO2 led to large increases of biomass at the highest N supply, small increases at a moderately limiting N supply, and no increase, or even a slight decrease, at the lowest N supply. Therefore, nutrient supply and, consequently, the nutrient status of plants should be a critical factor determining growth responses to the elevated CO2. We have shown that the elevated CO2 treatments significantly increased the Fe concentrations in tomato leaves (Table I) and alleviated the Fe-deficiency-induced chlorosis (Fig. 1) when grown in Fe-limited medium. Furthermore, the relative increases in biomass at elevated CO2 in Fe-limited plants were greater than the increases measured in Fe-sufficient plants (Table I). Therefore, the increase in plant biomass under elevated CO2 and restricted Fe supply (Fig. 2) cannot be attributed to increased photosynthesis alone, but also to the improved Fe nutrition of the plants.
Elevated CO2 Enhances Fe Acquisition from the Fe-Limited Medium
How, then, does the elevated CO2 increase Fe status of plants grown in Fe-limited medium alleviate the Fe-deficiency-induced chlorosis? Increased activities of FCR and the Fe(II) transporter IRT1 in roots are indispensable for plant adaptation to Fe deficiency (Robinson et al., 1999; Vert et al., 2002; Curie and Briat, 2003) and we found that both FCR activity and LeFRO1 and LeIRT1 expression in Fe-limited plants were significantly enhanced by the elevated CO2 (Figs. 2B and 5B). Graziano and Lamattina (2007) claimed that, while these two responses are essential responses to Fe deficiency, they are not sufficient to confer a noticeable increase of Fe concentration in plants under Fe-limited conditions. Their argument was based on two observations: (1) NO-stimulated FCR activity and expression of LeIRT1 did not significantly increase the Fe concentration of the plants grown in low Fe medium (0.1 μm FeEDTA; Graziano and Lamattina, 2007); (2) Arabidopsis (Arabidopsis thaliana) plants overexpressing AtIRT1 or AtFRO2 and grown in Fe-deficient medium do not accumulate more Fe than the wild-type plants (Connolly et al., 2002, 2003). However, the similar Fe concentrations in the transgenic and wild-type plants may have been caused by the fact that Fe was not available for reduction and uptake in the Fe-deficient treatment used in those experiments (Connolly et al., 2002, 2003). This explanation may also be true for the finding of Graziano and Lamattina (2007) because 0.1 μm FeEDTA is very low for plant growth and can be quickly depleted from nutrient medium. Recently, Yuan et al. (2008) reported that the overexpression of FIT (the Arabidopsis ortholog of FER; Bauer et al., 2007) with either AtbHLH38 or AtbHLH39 in Arabidopsis, which results in constitutive accumulation of AtIRT1 protein and high FCR activity in roots, significantly increases Fe accumulation in shoots. Therefore, we propose that the increase of FCR activity and expression of the LeIRT1 gene found in this study contributes, at least in part, to the increased Fe concentration of plants grown under elevated CO2 and limited Fe.
Dicotyledons also acidify the rhizosphere as part of the strategy I responses to Fe deficiency (Römheld and Marschner, 1986). In well-aerated soils, the solubility of the inorganic Fe depends on the reversible dissolution and precipitation of the ferric oxides in the soil and a decrease of one pH unit will theoretically increase the Fe solubility by 1,000-fold (Lindsay and Schwab, 1982). Therefore, the solubility of Fe strongly depends on the proton activity in the medium. The increase in soluble Fe concentration in these experiments can be estimated by GEOCHEM-PC (Parker et al., 1995). In the growth medium containing hydrous Fe(III)-oxide, the greater acidification in the elevated CO2 treatment (Fig. 3) would lead to a 12-fold greater concentration of soluble Fe compared to the ambient CO2 treatment. This response may contribute to the increased Fe accumulation in plants under the elevated CO2 environment and Fe-limited medium.
Development of dense subapical root hairs is another typical morphological response of strategy I plants to Fe deficiency (Römheld and Marschner, 1986; Schmidt, 1999). Longer root hairs or a greater root hair density greatly enlarges the root surface area and allows for a larger soil volume to be explored. The formation of root hairs in response to Fe deficiency is associated with cell-specific accumulation of transcripts that are involved in Fe acquisition (Santi and Schmidt, 2008). Therefore, subapical root hair development enhanced by elevated CO2 (Fig. 4) may also contribute partly to the increased Fe accumulation in plants grown in the Fe-limited medium. In this study, however, we found that the subapical root region of Fe-limited plants did not develop root hairs in both the ambient and elevated CO2 treatments on day 7 of the treatment (Fig. 4), even though FCR activity of roots was higher in the elevated CO2 treatment (Fig. 2B). This indicates that the subapical root hair development may only play a minor role in increasing Fe uptake of plants under the combined conditions of Fe limitation and elevated CO2. Importantly, the role of root hairs is likely to have been underestimated in these hydroponic experiments because the dissolved nutrients can easily diffuse to the roots. The role of root hairs will be more important in soil experiments.
Elevated CO2 stimulated root growth and resulted in a significantly greater root-to-shoot ratio (Table I). Similar changes in root-to-shoot ratio have previously been interpreted as a mechanism by which plants can take up more nutrients when growth is stimulated by the elevated CO2 concentration (H.H. Rogers et al., 1996).
Taken together, the elevated CO2 under Fe-limited conditions enhances root growth, root hair development, proton release, root FCR activity, and expressions of LeFRO1 and LeIRT1 genes, all of which enable plants to access and accumulate more Fe. Importantly, elevated CO2 did not induce these responses in plants that were well supplied with Fe (Figs. 2A, 3A, 4A, and 5A) and the Fe concentrations in those plants remained unchanged (Table I). A combination of limited Fe availability and elevated CO2 was required to induce the symptoms typical of Fe deficiency.
A Possible Role for NO
Then, how does the elevated CO2 enhance the Fe-deficiency-induced responses of plants under Fe-limited conditions? It is clear that this enhancement should not be related to the extent of Fe deficiency because the plant Fe nutrient status was better in the elevated than ambient CO2 (Fig. 1; Table I). Recently, NO was shown to be a general signal molecule involved in inducing the adaptive responses of roots to Fe-deficient conditions, including enhancing the expressions of genes involved in Fe uptake (Graziano and Lamattina, 2007). We found in this study that NO levels in roots in response to Fe deficiency were consistently greater in elevated CO2 (Fig. 6) and that the NO scavenger cPTIO inhibited the usual changes associated with Fe deficiency that affect FCR activity and LeFRO1 and LeIRT1 expression (Fig. 7). These results suggest that the adaptive responses induced by elevated CO2 and Fe deficiency may be controlled by NO. Interestingly, although the elevated CO2 treatment significantly increased NO levels in roots of Fe-sufficient plants, previous studies have shown that the Fe deficiency responses were not induced in those plants (Graziano and Lamattina, 2007). This may also be the reason why the elevated CO2 only specifically enhanced the Fe-deficiency-induced responses of the Fe-limited tomatoes.
The bHLH protein FER has been demonstrated to regulate the responses to Fe deficiency in tomato roots (Ling et al., 2002; Bereczky et al., 2003; Li et al., 2004). Moreover, the overexpression of FIT confers the increased FCR activity in Fe-deficient plants (Jakoby et al., 2004). We also found that the expression of FER was increased by a combination of Fe deficiency and elevated CO2 (Fig. 5B) and that cPTIO treatment prevented the accumulation of FER transcripts (Fig. 7B). Furthermore, the FER protein is necessary to mediate the regulatory function of NO in Fe-deficiency-induced responses (Graziano and Lamattina, 2007).
The question of how elevated CO2 increases NO levels in roots remains open. CO2 could enhance the activity of nitrate reductase in plants (Buchanan et al., 2000), which is a major enzyme in NO synthesis through the nitrite reduction (Yamasaki et al., 1999; Meyer et al., 2005). Alternatively, elevated CO2 could increase auxin levels (Li et al., 2002; Teng et al., 2006), which then induces NO accumulation (Du et al., 2008). These issues require further investigation, but we propose that elevated CO2 first increases NO levels in roots by enhancing the nitrate reductase activity and then enhances FER expression. These changes strengthen the molecular and physiological responses to Fe deprivation and induce the morphological changes in roots.
We have demonstrated that a combination of elevated CO2 and Fe limitation can induce a set of morphological, physiological, and molecular responses in plants that improve their Fe status by enabling them to better access Fe from sparingly soluble sources. NO may be a signaling molecule that controls these processes.
MATERIALS AND METHODS
Plant Culture
The tomato (Lycopersicon esculentum ‘Zheza 809’) seeds were germinated in 0.5 mm CaSO4 solution. Seven days after sowing, seedlings of similar size were transferred to 1-L pots (four holes per seedling holder, and one seedling per hole) filled with aerated, full-strength complete nutrient solution. The nutrient solution had the following composition (in μm): KH2PO4 250, MgSO4 500, KNO3 1,000, Ca(NO3)2 500, H3BO3 10, MnSO4 0.5, ZnSO4 0.5, CuSO4 0.1, (NH4)6Mo7O24 0.1, FeEDTA 20. The solution pH was adjusted to 6.5 using 1 m NaOH. The nutrient solutions were renewed every 3 d. All plants were grown in the controlled-environment growth chambers at a humidity of 70%, with a daily cycle of a 28°C, 14-h day and a 22°C, 10-h night. The daytime light intensity was 180 μmol photons m−2 s−1.
After 13 d of growth in the complete nutrient solution, one-half of the plants were transferred to an otherwise identical nutrient solution with the FeEDTA replaced with 0.3 g/L hydrous Fe(III)-oxide, and another one-half of the plants were continuously cultured in the 20 μm Fe EDTA contained nutrient solution. Meantime, CO2 treatments were also initiated by growing the above plants in the chambers with a CO2 concentration of either 350 (ambient) or 800 (elevated CO2) μL L−1. The hydrous Fe(III)-oxide, which consisted of hematite together with a trace amount of by-produced goethite, was prepared according to Schwertmann and Cornell (1991). To avoid the hydrous Fe(III)-oxide adhering to the root surface during cultivation, the hydrous Fe(III)-oxide was placed in a 36-mm-diameter dialysis bag (Puyi) with 12,000 to 18,000 Mr cutoff, which could ensure that smaller molecules like Fe ions and their chelates pass freely in or out of the bag. The initial soluble Fe concentration in the hydrous Fe(III)-oxide nutrient solution was 0.06 μm, which could be taken as Fe-limited medium. The nutrient solution was renewed every 3 d and was supplemented to 1 L with deionized water daily.
cPTIO Treatment
As we found that the NO levels of roots in hydrous Fe(III)-oxide medium were increased by elevated CO2, a NO scavenger, cPTIO [2-(4-carboxyphenyl)-4,4,5,5-tetramethyl-imidazoline-1-oxyl-3-oxide], was used to investigate the possible role of NO in regulating the enhancement of Fe-deficient responses induced by elevated CO2. After 2 d of growth in the hydrous Fe(III)-oxide-containing nutrient solution under elevated CO2, part of plants were transferred to an otherwise identical growth solution containing 200 μm cPTIO. After 24 h, FCR activity and mRNA levels of FER, LeFRO1, and LeIRT1 in roots were analyzed following the methods described below.
Chlorophyll Synthesis and Biomass Analysis
After 7 d of growth under elevated CO2, the chlorophyll content of the newly formed leaves was analyzed with a chlorophyll meter (SPAD-502; Minolta) and recorded as a SPAD reading. After chlorophyll content recording, the plants were separated into shoots and roots with scissors. Roots were washed with deionized water and blotted dry with a paper towel. The shoots and roots were weighed and dried in a 75°C oven to a constant weight for elements content analysis.
Analysis of Proton Extrusion Rate
After the plants were subjected to ambient and elevated CO2 treatments, the pH in nutrient solution was measured every day with a pH electrode (METROHM). The proton extrusion rate was analyzed following the method of Römheld et al. (1984). Briefly, the individual plants precultured with hydrous Fe(III)-oxide nutrient solution under ambient or elevated CO2 treatment were transferred to 300-mL pots filled with Fe-omitted nutrient solution and were continuously grown under ambient or elevated CO2 conditions. After 12 h, each solution was filtered and the volume was adjusted to the initial value. The net release of protons was determined by titrating the solution with 10−3m NaOH. Prior to the determination of the fresh weight of roots, the excess water was removed by blotting with filter paper. The aim of omitting hydrous Fe(III)-oxide from nutrient solution in this experiment was to avoid the reaction of protons with hydrous Fe(III)-oxide so as to more accurately estimate the proton extrusion rate.
Determination of FCR Activity
FCR activity was determined following our previously research (Jin et al., 2007). Briefly, 1 g of the excised roots (<10 cm from the root tips) was placed in an Erlenmeyer flask filled with 50 mL of an assay solution consisting of 0.5 mm CaSO4, 0.1 mm MES, 0.1 mm 4,7-diphenyl-1,10-phenanhroline-disufonic acid, and 100 μm Fe EDTA at pH 5.5 adjusted by 1 m NaOH. The flasks were placed in a dark room at 25°C for 1 h, with periodic hand swirling at 15-min intervals. The absorbance of the assay solutions was recorded by a spectrophotometer at 535 nm, and the concentration of Fe(II)[BPDS]3 was quantified using an extinction coefficient of 22.14 mm−1 cm−1.
Root Morphology Observation
Observation of the root subapical root hair patterns was performed using light microscopy with differential interference contrast optics. Photomicrographs were recorded on a CCD camera (Nikon Eclipse E600).
In Situ Measurement of NO in the Root
NO was imaged using DAF-FM DA and epifluorescence microscopy. Roots were loaded with 5 μm DAF-FM DA in 20 mm HEPES-NaOH buffer (pH 7.4) for 30 min, washed three times in fresh buffer, and observed under a microscope (Nikon Eclipse E600; Nikon; excitation 488 nm, emission 495–575 nm). Twenty roots of each treatment were measured each time. The signal intensities of green fluorescence in the images of the young root hair zone (3–10 mm from root tip) were quantified according to the method of Guo and Crawford (2005) by using Photoshop software (Adobe Systems). Data are presented as the mean of fluorescence intensity relative to the roots of day 1 ambient treatment.
Real-Time Reverse Transcription-PCR Analyses
Fe Content Analysis
The dried root and shoot samples were wet digested in the concentrated HNO3 at 120°C until there was no brown NO gas emitting, then further digested with HNO3/HClO4 at 180°C until the solution became transparent. Digestates were diluted by ultrapure water and the concentration of Fe in the digestates was analyzed by inductively coupled plasma-mass spectrometry (Agilent 7500a).
Statistics
All statistical analyses were conducted with SAS software (SAS Institute). Means were compared by t test or Fisher's LSD test at P < 0.05 in all cases.
ACKNOWLEDGMENTS
We thank the anonymous reviewers for their constructive suggestions, as well as Dr. Peter Ryan from CSIRO for critical reading and revision of the manuscript.
LITERATURE CITED
Bauer P, Ling HQ, Guerinot ML (
Baxter R, Gantley M, Ashenden TW, Farrar IF (
Bereczky Z, Wang HY, Schubert V, Ganal M, Bauer P (
Bolin B, Kheshgi HS (
Buchanan BB, Gruissem W, Jones RL (
Connolly EL, Campbell NH, Grotz N, Prichard CL, Guerino ML (
Connolly EL, Fett JP, Guerinot ML (
Conroy JP (
Conroy JP, Milham PJ, Barlow EWR (
Curie C, Briat JF (
Dijkstra P, Hymus G, Colavito D, Vieglais DA, Cundari CM, Johnson DP, Hungate BA, Hinkle CR, Drake BG (
Du ST, Zhang YS, Lin XY, Wang Y, Tang CX (
Eide D, Broderius M, Fett J, Guerinot ML (
Gebauer RLE, Reynolds JF, Strain BR (
Geiger M, Haake V, Ludewig F, Sonnewald U, Stitt M (
Graziano M, Lamattina L (
Guerinot ML, Yi Y (
Guo FQ, Crawford NM (
Imsande J (
Intergovernmental Panel on Climate Change (
Jakoby M, Wang HY, Reidt W, Weisshaar B, Bauer P (
Jin CW, You GY, Tang CX, Wu P, Zheng SJ (
Kimball BA, Mauney JR (
Kläring HP, Hauschild C, Heißner A, Bar-Yosef B (
Kogawara S, Norisada M, Tange T, Yagi H, Kojima K (
Lawlor DW, Mitchell RAC (
Li CR, Gan LJ, Xia K, Zhou X, Hew CS (
Li L, Cheng X, Ling HQ (
Lindsay WL, Schwab AP (
Ling HQ, Bauer P, Bereczky Z, Keller B, Ganal M (
Lloyd J, Farquhar GD (
McKee IF, Woodward FI (
Meleshkevitch EA, Assis-Nascimento P, Popova LB, Miller MM, Kohn AB, Phung EN, Mandal A, Harvey WR, Boudko DY (
Meyer C, Lea US, Provan F, Kaiser WM, Lillo C (
Newbery RM, Wolfenden J, Mansfield TA, Harrison AF (
Parker DR, Norvell WA, Chaney RL (
Pettersson R, MacDonald JS, Stadenburg I (
Robinson NJ, Procter CM, Connolly EL, Guerinot ML (
Rogers GS, Milham PJ, Gillings M, Conroy JP (
Rogers GS, Payne L, Milham P, Conroy J (
Rogers HH, Prior SA, Runios GB, Mitchell RJ (
Römheld V, Marschner H (
Römheld V, Muller C, Marschner H (
Santi S, Schmidt W (
Sasaki T, Kurano N, Miyachi S (
Schmidt W (
Schwertmann U, Cornell RM (
Silvola J, Ahlholm U (
Stitt M, Krapp A (
Teng NJ, Wang J, Chen T, Xu W, Wang Y, Li J (
Vert G, Grotz N, Dedaldechamp F, Gaymard F, Guerinot ML, Briat JF, Curie C (
Yamasaki H, Sakihama Y, Takahashi S (
Yi Y, Guerinot ML (
Yuan YX, Wu HL, Wang N, Li J, Zhao WN, Du J, Wang DW, Ling HQ (
Author notes
This work was supported by the Natural Science Foundation of China (grant no. 30625026) and the China Postdoctoral Science Foundation (grant no. 20080440204).
Corresponding author; e-mail [email protected].
The author responsible for the distribution of materials integral to the findings presented in this article in accordance with the policy described in the Instructions for Authors (www.plantphysiol.org) is: Shao Jian Zheng ([email protected]).
Open access articles can be viewed online without a subscription.