-
PDF
- Split View
-
Views
-
Cite
Cite
Robert Edward Sharwood, Susanne von Caemmerer, Pal Maliga, Spencer Michael Whitney, The Catalytic Properties of Hybrid Rubisco Comprising Tobacco Small and Sunflower Large Subunits Mirror the Kinetically Equivalent Source Rubiscos and Can Support Tobacco Growth, Plant Physiology, Volume 146, Issue 1, January 2008, Pages 83–96, https://doi.org/10.1104/pp.107.109058
- Share Icon Share
Abstract
Plastomic replacement of the tobacco (Nicotiana tabacum) Rubisco large subunit gene (rbcL) with that from sunflower (Helianthus annuus; rbcLS) produced tobaccoRst transformants that produced a hybrid Rubisco consisting of sunflower large and tobacco small subunits (LsSt). The tobaccoRst plants required CO2 (0.5% v/v) supplementation to grow autotrophically from seed despite the substrate saturated carboxylation rate, K m, for CO2 and CO2/O2 selectivity of the LsSt enzyme mirroring the kinetically equivalent tobacco and sunflower Rubiscos. Consequently, at the onset of exponential growth when the source strength and leaf LsSt content were sufficient, tobaccoRst plants grew to maturity without CO2 supplementation. When grown under a high pCO2, the tobaccoRst seedlings grew slower than tobacco and exhibited unique growth phenotypes: Juvenile plants formed clusters of 10 to 20 structurally simple oblanceolate leaves, developed multiple apical meristems, and the mature leaves displayed marginal curling and dimpling. Depending on developmental stage, the LsSt content in tobaccoRst leaves was 4- to 7-fold less than tobacco, and gas exchange coupled with chlorophyll fluorescence showed that at 2 mbar pCO2 and growth illumination CO2 assimilation in mature tobaccoRst leaves remained limited by Rubisco activity and its rate (approximately 11 μmol m−2 s−1) was half that of tobacco controls. 35S-methionine labeling showed the stability of assembled LsSt was similar to tobacco Rubisco and measurements of light transient CO2 assimilation rates showed LsSt was adequately regulated by tobacco Rubisco activase. We conclude limitations to tobaccoRst growth primarily stem from reduced rbcLS mRNA levels and the translation and/or assembly of sunflower large with the tobacco small subunits that restricted LsSt synthesis.
Significant biotechnological effort and expense is being devoted to engineering improvements to CO2 assimilation in C3 plants using various strategies (for review, see Raines, 2006; Parry et al., 2007), with recent success coming by transplanting the glycolate catabolic pathway from Escherichia coli into Arabidopsis (Arabidopsis thaliana) chloroplasts that localized recycling of 2-phosphoglycolate (2-PG) to glycerate in the stroma, thereby increasing the CO2 levels around the photosynthetic CO2-fixing enzyme, Rubisco (Kebeish et al., 2007). The production of 2-PG is a consequence of Rubisco's catalytic bifunctionality that sees it not only catalyze the productive carboxylation of ribulose-P2 into two molecules of 3-phosphoglycerate (3-PGA), but also the oxygenation of ribulose-P2 producing one 3-PGA and one 2-PG molecule. The 2-PG is considered a waste product since its recycling via photorespiration is energetically demanding and also reduces net carbon assimilation due to CO2 release during Gly decarboxylation in the mitochondria (Wingler et al., 2000; Kebeish et al., 2007). The catalytic properties of Rubisco therefore play a critical, frequently rate-limiting, role in photosynthetic carbon assimilation and have a pervasive influence on the efficiency with which plants uses their resources of light, water, and nitrogen. Accordingly, improving the catalytic properties of Rubisco and transplanting such improvements into crop plants has been a longstanding goal toward improving growth efficiencies (Andrews and Whitney, 2003; Raines, 2006; Parry et al., 2007). These efforts are spurred on by the natural variation in Rubisco's kinetic properties, particularly the kinetically better versions from nongreen algae that have the capacity to profoundly improve crop growth (Zhu et al., 2004). The natural variability in the catalytic properties of Rubisco also indicates engineering improvements into higher-plant versions are unlikely to be immutable and theoretically provides a means to improve the efficiency of photosynthesis through genetic manipulation of a single protein rather than by introducing an entirely foreign pathway.
Genetic manipulation of Rubisco in crop plants is hindered by several factors that are particularly complicated by the disparate location of the genes coding for the approximately 54 kD large subunit (L, coded by a single rbcL gene in the plastome) and the approximately 14 kD small subunits (S, coded by multiple RbcS gene copies in the nucleus). Assembly of the cytosolically synthesized S with the stromally synthesized L that contain the catalytic active sites into hexadecameric enzyme (L8S8) within the stroma occurs via a complex, highly organized chaperone-assisted mechanism that further restrains genetic manipulation of Rubisco in higher plants (Whitney and Andrews, 2001a; Zhang et al., 2002). It has been shown the preferred position of rbcL is in the chloroplast genome (plastome; Kanevski and Maliga, 1994), while an appropriate means for the engineering changes to the native (or inserting a foreign) RbcS in higher plants have remained elusive. Directly transplanting in catalytically more efficient Rubiscos from nongreen algae by transplastomic insertion of their genes for L and S into the tobacco (Nicotiana tabacum) plastome was frustrated by folding incompatibilities that precluded productive assembly of the foreign enzyme in tobacco chloroplasts (Whitney et al., 2001). Directed manipulation of RbcS in higher plants is complicated by the multiple copies that essentially precludes them from targeted mutagenic or replacement strategies. The difficulty with engineering changes to S via transplastomic manipulation is further compounded by an apparent preferential assembly of cytosolically synthesized S with the L subunits (Whitney and Andrews, 2001a; Zhang et al., 2002). Only by reducing the level of cytosolic S by >90% by antisensing RbcS and inserting a copy of rbcS in a highly transcribed region of the tobacco chloroplast genome could sufficient quantities of plastid synthesized S be incorporated into assemble tobacco Rubisco hexadeamers (Dhingra et al., 2004).
The generation of fully autotrophic and reproductive transplastomic tobacco-rubrum lines where the native L8S8 tobacco Rubisco has been replaced by the structurally simple L2 form from the bacterium Rhodospirillum rubrum demonstrated the feasibility of replacing Rubisco in higher plants (Whitney and Andrews, 2001b). These lines were produced via homologous replacement of the tobacco rbcL gene with the R. rubrum Rubisco gene, rbcM. Importantly, the CO2 response of photosynthesis in the tobacco-rubrum transformants was consistent with the content and kinetic properties of R. rubrum Rubisco (Whitney and Andrews, 2003). Earlier attempts to replace tobacco rbcL with the comparable gene from a cyanobacterium (Synechococcus PCC6301) or sunflower (Helianthus annuus) produced transformants that were nonautotrophic since they produced either no Rubisco (those containing the PCC6301 rbcL) or assembled a hybrid Rubisco hexadecamer (LsSt) comprising sunflower L and tobacco S (Kanevski et al., 1999). In the leaves of the latter transformant (line Nt-pIK83-1; Kanevski et al., 1999) grown in tissue culture the amount of hybrid enzyme was reduced by approximately 70%. The impeded autotrophic growth of line Nt-pIK83-1 in air necessitated grafting of the tissue onto wild-type tobacco to procure T0 seed (Kanevski et al., 1999).
In this study we show that line Nt-pIK83-1 can be grown photoautotrophycally at elevated CO2 levels and demonstrate that the kinetic properties of the hybrid LsSt mirror those of the source Rubiscos and that the hybrid enzyme is regulated appropriately by tobacco activase in vivo. We show the inability of juvenile Nt-pIK83-1 plants to grow in air and their delayed development at high pCO2 is likely due to inadequate amounts of hybrid LsSt enzyme in the young vegetative tissue to provide sufficient source strength for development.
RESULTS
TobaccoRst Variants Grown in Soil at High CO2 Have Abnormal Phenotypic Features
Successful replacement of the plastome rbcL copy in tobacco with sunflower rbcL (rbcLS) by Kanevski et al. (1999) produced tobacco transformants that produced a hybrid Rubisco comprising eight sunflower large subunits (Ls) and eight tobacco small subunits (St). We identify the transformed line Nt-pIK83-1 (Kanevski et al., 1999) as tobaccoRst (tobacco with a hybrid Rubisco comprising sunflower L and tobacco S) and its hybrid hexadecameric Rubisco as LsSt. Limitations in the catalytic properties and content of LsSt were proposed to perturb autotrophic growth in air of tobaccoRst plants grown in soil. Analogous to other tobacco transgenic lines expressing less Rubisco (Rodermel et al., 1988; Hudson et al., 1992) or catalytically perturbed foreign or mutated Rubiscos (Whitney et al., 1999; Whitney and Andrews, 2001b), the T1 generation of the tobaccoRst line Nt-pIK83-1 (Kanevski et al., 1999) could be grown in soil in air enriched with CO2. The phenotype of the T1 progeny was different to wild-type tobacco (see figure 2 in Andrews and Whitney, 2003) and to ensure the phenotype was not a consequence of unwanted nuclear mutations the tobaccoRst flowers were repeatedly backcrossed with wild-type pollen. No change in the phenotype of multiple plants screened in the T2 or T3 progeny have been found and sequencing of the rbcLS and flanking plastome sequence (approximately 0.2 kb either side) showed no unwanted mutations.
![Growth and phenotype of tobaccoRst plants compared with wild-type tobacco grown in air containing 0.5% (v/v) CO2 (see “Material and Methods” for further details). A, Phenotype of wild-type and tobaccoRst (B and C) plants (tobRst1 and tobRst4, both germinated from the same seed stock of a T2 plant derived from previous generations only backcrossed with wild-type pollen) at the juvenile (top sections) and mature growth stage. The number of days since cotyledon emergence is shown. The white arrows indicate the normal oblanceolate leaves (A and B) and irregular oblanceolate leaves (C) analyzed in Figure 7. D, Change in height of the primary axial shoot for wild-type (•, n = 6) and tobaccoRst plants with one (tobRst1, ○, n = 1), two (□, n = 2), or four (tobRst4, ▵, n = 1) primary axial shoots. The exponential growth rates (black lines) were modeled to the height data according to the equation\batchmode \documentclass[fleqn,10pt,legalpaper]{article} \usepackage{amssymb} \usepackage{amsfonts} \usepackage{amsmath} \pagestyle{empty} \begin{document} \[y{=}\mathrm{A}1^{{\ast}}\mathrm{exp}^{(x/t_{1})}{+}Y_{0}\] \end{document}where A1 is the amplitude, t 1 the time constant (calculated values are shown), and Y 0 is the offset. E, Comparison of a mature wild-type and tobRst leaf (F) with the marginal dimpling phenotype in the tobRst lines not evident when grown in Suc containing tissue culture medium (G; Svab and Maliga, 1993).](https://oup.silverchair-cdn.com/oup/backfile/Content_public/Journal/plphys/146/1/10.1104_pp.107.109058/2/m_plphys_v146_1_83_f1.jpeg?Expires=1750521843&Signature=Q4pVScWbi5-nFPhHpLT8MvrZKxZslR4U4vUI3KwepTZrNF-MhurO3HkmHrN~jDqXgfBm7vzwLeRA1Z~l8YAKKhZlOBmpxbbZjJTz4iUD6hBtTC5K4rBbWh7ag0bOvrPUw7OEkYL58Iumc~Is2MJBy6kbTeQoFTVmXCMXOEOAj0rcDx92TOTP~xEf6Xhtd-nlkXMi4pVRJIYF08retQtUJZOxT4csc-3oHwDJAivHNX0WkVXCuLoWtUt1RiKmKiXJO03Z5uEjnxZ9yvcip2VMsB360pivAg4xQ7wpRL03zr-kDfyV8WHvBWQqYnMk7I~Mk8WViE1iVImII9ocjHBkaA__&Key-Pair-Id=APKAIE5G5CRDK6RD3PGA)
The mature leaves of tobaccoRst were phenotypically different to wild type in that they exhibited curling and dimpling around the leaf margin (Fig. 1, E and F). The severity of this abnormal leaf phenotype was less noticeable in younger tobaccoRst leaves and was absent in the leaves of tissue cultured tobaccoRst plants grown on Suc-containing media (Fig. 1G). The width of the mature leaves produced by the tobaccoRst plants, in particular those with multiple shoots, were smaller than the corresponding leaves from wild-type controls of comparable physiological development (Fig. 1, E and F). For example, the fifth leaf from the apical meristem from the 60 cm high plants used for biochemical analyses (Table I
Leaf Measurement . | Wild-Type Tobacco . | TobaccoRst . |
---|---|---|
Fresh weight (g m−2) | 233.7 ± 16.9 | 234.8 ± 26.8 |
Dry weight (g m−2) | 69.8 ± 5.9 | 47.1 ± 5.7 |
Chlorophyll a and b content (mg m−2) | 2.2 ± 0.1 | 2.85 ± 0.7 |
Chlorophyll a/b ratio | 2.5 ± 0.1 | 2.7 ± 0.2 |
F v/F m | 0.80 ± 0.01 | 0.77 ± 0.02 |
Protein (g m−2) | 7.2 ± 1.0 | 4.5 ± 1.7 |
Rubisco (g m−2) | 1.35 ± 0.3 | 0.31 ± 0.2 |
Ribulose-P2 (mg m−2) | 31.0 ± 8.2 | 64.0 ± 22.1 |
P-glycerate and triose P (mg m−2) | 111.1 ± 28.5 | 81.7 ± 5.8 |
Ribulose-P2 to P-glycerate ratio | 0.4 | 3.6 |
Starch (g m−2) | 23.7 ± 2.7 | 14.9 ± 1.2 |
n = 4 ± sd | n = 3 ± sd |
Leaf Measurement . | Wild-Type Tobacco . | TobaccoRst . |
---|---|---|
Fresh weight (g m−2) | 233.7 ± 16.9 | 234.8 ± 26.8 |
Dry weight (g m−2) | 69.8 ± 5.9 | 47.1 ± 5.7 |
Chlorophyll a and b content (mg m−2) | 2.2 ± 0.1 | 2.85 ± 0.7 |
Chlorophyll a/b ratio | 2.5 ± 0.1 | 2.7 ± 0.2 |
F v/F m | 0.80 ± 0.01 | 0.77 ± 0.02 |
Protein (g m−2) | 7.2 ± 1.0 | 4.5 ± 1.7 |
Rubisco (g m−2) | 1.35 ± 0.3 | 0.31 ± 0.2 |
Ribulose-P2 (mg m−2) | 31.0 ± 8.2 | 64.0 ± 22.1 |
P-glycerate and triose P (mg m−2) | 111.1 ± 28.5 | 81.7 ± 5.8 |
Ribulose-P2 to P-glycerate ratio | 0.4 | 3.6 |
Starch (g m−2) | 23.7 ± 2.7 | 14.9 ± 1.2 |
n = 4 ± sd | n = 3 ± sd |
Leaf Measurement . | Wild-Type Tobacco . | TobaccoRst . |
---|---|---|
Fresh weight (g m−2) | 233.7 ± 16.9 | 234.8 ± 26.8 |
Dry weight (g m−2) | 69.8 ± 5.9 | 47.1 ± 5.7 |
Chlorophyll a and b content (mg m−2) | 2.2 ± 0.1 | 2.85 ± 0.7 |
Chlorophyll a/b ratio | 2.5 ± 0.1 | 2.7 ± 0.2 |
F v/F m | 0.80 ± 0.01 | 0.77 ± 0.02 |
Protein (g m−2) | 7.2 ± 1.0 | 4.5 ± 1.7 |
Rubisco (g m−2) | 1.35 ± 0.3 | 0.31 ± 0.2 |
Ribulose-P2 (mg m−2) | 31.0 ± 8.2 | 64.0 ± 22.1 |
P-glycerate and triose P (mg m−2) | 111.1 ± 28.5 | 81.7 ± 5.8 |
Ribulose-P2 to P-glycerate ratio | 0.4 | 3.6 |
Starch (g m−2) | 23.7 ± 2.7 | 14.9 ± 1.2 |
n = 4 ± sd | n = 3 ± sd |
Leaf Measurement . | Wild-Type Tobacco . | TobaccoRst . |
---|---|---|
Fresh weight (g m−2) | 233.7 ± 16.9 | 234.8 ± 26.8 |
Dry weight (g m−2) | 69.8 ± 5.9 | 47.1 ± 5.7 |
Chlorophyll a and b content (mg m−2) | 2.2 ± 0.1 | 2.85 ± 0.7 |
Chlorophyll a/b ratio | 2.5 ± 0.1 | 2.7 ± 0.2 |
F v/F m | 0.80 ± 0.01 | 0.77 ± 0.02 |
Protein (g m−2) | 7.2 ± 1.0 | 4.5 ± 1.7 |
Rubisco (g m−2) | 1.35 ± 0.3 | 0.31 ± 0.2 |
Ribulose-P2 (mg m−2) | 31.0 ± 8.2 | 64.0 ± 22.1 |
P-glycerate and triose P (mg m−2) | 111.1 ± 28.5 | 81.7 ± 5.8 |
Ribulose-P2 to P-glycerate ratio | 0.4 | 3.6 |
Starch (g m−2) | 23.7 ± 2.7 | 14.9 ± 1.2 |
n = 4 ± sd | n = 3 ± sd |
To unequivocally demonstrate the phenotypic anomalies of the Nt-pIK83-1 tobaccoRst line are a direct consequence of replacing rbcL with rbcLS, additional tobacco transplastomic lines tRstLA7 and tRstLA13 that replace the tobacco L with the sunflower homolog were independently generated (Supplemental Fig. S1, A and B). The phenotype of the T1 progeny of both transplastomic lines mimics that displayed in the T3 tobaccoRst progeny (Supplemental Fig. S1, C and D).
Photosynthesis in TobaccoRst Leaves Corresponds to the Content and Catalytic Properties of the Hybrid Rubisco
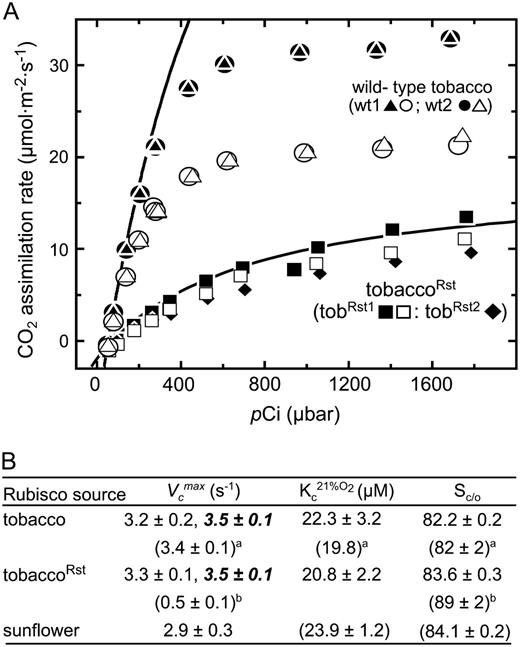
CO2 assimilation rates in tobaccoRst and wild-type tobacco plants and their Rubisco kinetics. A, Gas-exchange measurements of CO2 assimilation rates in response to intercellular pCO2 measured at an irradiance of 350 (white symbols) or 950 (black symbols) μmol quanta m−2 s−1 and leaf temperature of 25°C. Measurements were made on attached leaves from two wild-type plants (wt1, 13.5 cm leaf diameter; wt2, 15 cm leaf diameter containing 33.2 and 30.6 μmol Rubisco sites m−2, respectively) and two tobaccoRst plants (tobRst1 and tobRst2; both 12 cm leaf diameters with 5.8 and 4.9 μmol Rubisco sites m−2, respectively). The Rubisco limited CO2 assimilation rates for wt1 (•) and tobRst2 (▪) were modeled according to Farquhar et al. (1980) using estimates of maximal ribulose-P2 dependent carboxylase activity. B, V c max, K m for CO2 under ambient O2 levels (K c 21%O 2), and CO2/O2 specificity (Sc/o) measured using leaf protein extracts and purified Rubisco preparations. See “Materials and Methods” for further details. V c max measurements in bold italics are those made using leaf tissue from tissue culture grown plants; see Supplemental Figure S3 for details. Superscript a indicates data from Whitney et al. (1999) and superscript b indicates data from Kanevski et al. (1999).
After taking into consideration the reduced Rubisco content, the initial slope of the assimilation curves for the tobaccoRst leaves were inconsistent with the hybrid LsSt having a 5-fold higher K m for CO2 (K c) and 4-fold lower substrate-saturated carboxylase activity (V c max) as reported previously (Kanevski et al., 1999). When limited by carboxylase activity, the slope of the CO2 assimilation rate in response to changing pCO2 is dependant on Rubisco content, V c max, and K c 21%O 2 (K m for CO2 at ambient pO2 and equals K c[1+O/K o] where K o is the K m for O2 and O is the O2 concentration; Farquhar et al., 1980; von Caemmerer et al., 1994). Both K c 21%O 2 and V c max were measured for the tobaccoRst, tobacco, and sunflower Rubiscos in vitro using rapidly sampled soluble protein extracts from mature leaves (Fig. 2B). The measured values for wild-type tobacco Rubisco matched those measured previously and were comparable to that measured for the sunflower and tobaccoRst Rubiscos, indicating all three enzymes show similar kinetics and, most importantly, that the catalytic turnover and substrate CO2 affinity of the hybrid enzyme was not impaired. Comparable rates of catalytic turnover were measured using leaf protein extracts from tobaccoRst and tobacco plants grown in tissue culture (Fig. 2B) even when extracted and assayed in the extraction buffer used by Kanevski et al. (1999; Supplemental Fig. S3).
Purified Rubisco from tobaccoRst, tobacco, and sunflower was used to measure their CO2/O2 selectivity, which again were highly similar (Fig. 2B). As evident in Figure 2A, the modeled assimilation rate of tobaccoRst using the in vitro kinetic (V c max, 3.3 s−1:K c 21%O 2, 20.8 μ m) and Rubisco content measurements (determined by [14C]carboxyarabinitol-P2 binding, see below) closely matched that measured by whole leaf gas exchange supporting the validity of the measured kinetic parameters.
Even in air containing 0.5% (v/v) CO2 the growth of tobaccoRst remains Rubisco activity limited. Ribulose-P2 content in young fully expanded tobaccoRst leaves was approximately 50% higher and the 3-PGA content approximately 3-fold lower compared to wild-type controls, resulting in a 9-fold increase in the ribulose-P2 to 3-PGA ratio (Table I). Both the dry weight and starch content of tobaccoRst leaves were similarly reduced one-third while the fresh weight of the tobaccoRst leaves matched wild type, indicating the leaves of the mutants were highly hydrated.
Rubisco and Rubisco Activase Content in Mature Leaves
![Rubisco activase abundance and Rubisco content and carbamylation status in comparable young, fully expanded leaves from wild-type tobacco (wt, n = 4) and tobaccoRst (tRst, n = 3) plants of similar physiological age (approximately 35 cm in height) grown in air containing 0.5% (v/v) CO2. Soluble protein from 2.7 mm2 of leaves was separated by SDS-PAGE and stained with Coomassie blue (A) or replicate samples (B) blotted and probed with antibodies raised against spinach Rubisco (Rubisco L antibody), tobacco Rubisco activase (tobacco activase antibody), or tobacco Rubisco S subunit (Rubisco S antibody; Whitney and Andrews, 2001a). C, The content of Rubisco active sites and their carbamylation status (D) measured by [14C]carboxyarabinitol-P2 binding/[12C]carboxyarabinitol-P2 exchange under the high-CO2 growth conditions. See “Materials and Methods” for further details.](https://oup.silverchair-cdn.com/oup/backfile/Content_public/Journal/plphys/146/1/10.1104_pp.107.109058/2/m_plphys_v146_1_83_f3.jpeg?Expires=1750521843&Signature=TyWGWrBf4usYla5ucIue7Cbdr8sOz-B6y1fWAacKpgb~86RP0PJvMVtMDiHjOnT5NQIyepceeMK0~p~Az~-c8CaFMu1HHOdOlkRmR9M2bURneMYUuwd5nd~1CyxIfLgqAO7ioh7L~ZuPGOy5V3p-QL4E3KZUpBXOq9U0ObbXHp80KC4JRmQUKT-bMb1w3KI5dQPGSZ~5Xix3cc5nmZ5Ek1T1S31mdz4hzwEn1NQxmcSBF~7ZvtfJpZCFOCPp6JmRGPCYhsfuKULSjF19XH9tWn75i6-9gJEjY9R42VDF0r5QH4xVnHDE3B-89d4JAXQtIxyjHzlZNSpehaRPnJ7new__&Key-Pair-Id=APKAIE5G5CRDK6RD3PGA)
Rubisco activase abundance and Rubisco content and carbamylation status in comparable young, fully expanded leaves from wild-type tobacco (wt, n = 4) and tobaccoRst (tRst, n = 3) plants of similar physiological age (approximately 35 cm in height) grown in air containing 0.5% (v/v) CO2. Soluble protein from 2.7 mm2 of leaves was separated by SDS-PAGE and stained with Coomassie blue (A) or replicate samples (B) blotted and probed with antibodies raised against spinach Rubisco (Rubisco L antibody), tobacco Rubisco activase (tobacco activase antibody), or tobacco Rubisco S subunit (Rubisco S antibody; Whitney and Andrews, 2001a). C, The content of Rubisco active sites and their carbamylation status (D) measured by [14C]carboxyarabinitol-P2 binding/[12C]carboxyarabinitol-P2 exchange under the high-CO2 growth conditions. See “Materials and Methods” for further details.
Regulation of LsSt by Tobacco Rubisco Activase
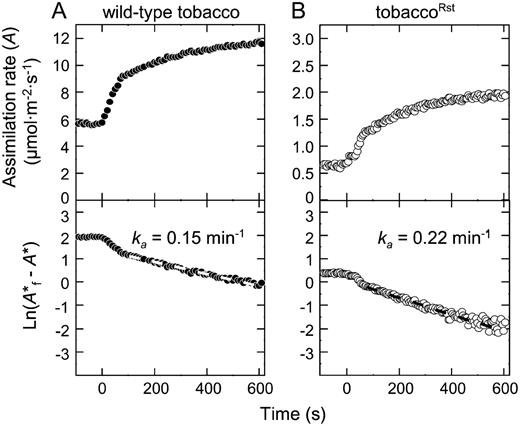
Measurement of the activation constant for tobacco and tobaccoRst Rubisco. Representative whole leaf gas-exchange measurements of the CO2 assimilation response for physiologically analogous leaves (12 cm leaf width, 35 cm plant height) from a wild-type tobacco (A) and a tobaccoRst (B) before and after leaf irradiance was suddenly increased from 110 to 1,200 μmol quanta m−2 s−1 (time zero). The chamber CO2 concentration and leaf temperature were set at 250 μbar and 25°C, respectively. Bottom panels show the data replotted as the natural logarithm of the final assimilation rate (A*f) minus the adjusted assimilation rate (A*) as described (Woodrow and Mott, 1989). See “Materials and Methods” for further details. The rate constant for Rubisco activation (k a) was calculated from the gradients of the linear second phase fitted to the wild-type (white dashed line) and tobaccoRst (black dashed line) measurements.
Stability of Assembled LsSt
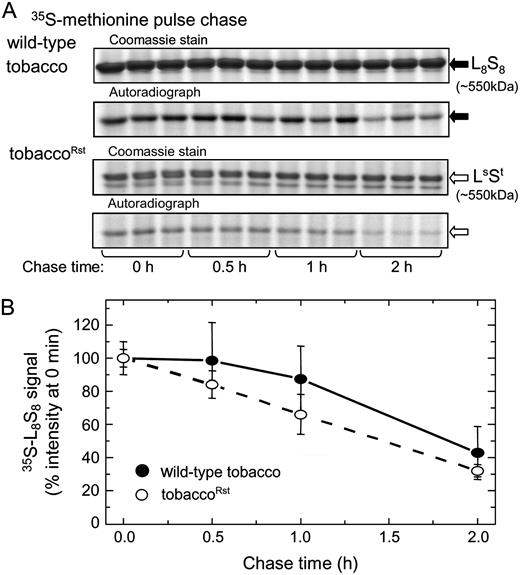
Rubisco turnover in tobacco and tobaccoRst leaves. Multiple leaf discs from the same leaf were infiltrated with 35S-Met and chased with 10 mm unlabeled Met for the times shown and the soluble protein from 18 mm2 of leaf separated by nondenaturing PAGE. A, Hexadecameric Rubisco from wild-type tobacco (L8S8, black arrow) and tobaccoRst (LsSt, white arrow) leaves identified in the gels by Coomassie staining and autoradiography. B, Densitometry measurements of the 35S-labeled Rubiscos during the chase periods (±sd of three separate leaf samples for each time point).
Relative Translational Efficiency of the Sunflower rbcLS Transcripts
![Plastome organization, transcript content, and translational efficiency of rbcL in comparable light adapted leaves of physiologically analogous tobacco (wt) and tobaccoRst (tRst) plants (approximately 60 cm in height). A, The tRst transformants have the tobacco rbcL gene replaced with the corresponding gene from sunflower (rbcLS) and contain a p-aadA-t gene cassette (p, 16S rDNA rrn promoter and 5′ UTR; t, rps16 3′ UTR; Svab and Maliga, 1993) inserted 174-bp downstream of the rbcL stop codon within the rbcL 3′ UTR (T). The annealing positions of the 5′ΔrbcL probe and the primers LsE and LsH are shown. The dotted lines and numbering (Shinozaki et al., 1986) refer to positioning of the homologously inserted foreign sequence into the tobacco plastome (GenBank accession no. Z00044). P, rbcL promoter and 5′ UTR. B, Blot of total leaf RNA (the amount and relative leaf area used are shown) probed with 5′ΔrbcL, showing the rbcL produced in wt and the mono- (rbcLS) and bi- (rbcLS-aadA) cistronic mRNAs produced in the tRst plants. Equivalent loading was confirmed by comparing the amount of 16SrRNA transcript (data not shown). C, The relative abundance of the rbcL and rbcLS mRNAs measured from B and the corresponding Rubisco content measured by [14C]CABP binding are expressed as a percentage to the average rbcL transcript and Rubisco content in the wt samples. The RTE was obtained by dividing Rubisco content by transcript abundance. For tRst, the RTE in parentheses is calculated from the sum of the abundances of the rbcLS and rbcLS-aadA transcripts.](https://oup.silverchair-cdn.com/oup/backfile/Content_public/Journal/plphys/146/1/10.1104_pp.107.109058/2/m_plphys_v146_1_83_f6.jpeg?Expires=1750521843&Signature=FF1wcwkNHsuiGKQE0blnQvZ62Zr6L69telw1K7csKXDcfdQ9APp6GwkUlQ6k9OcN5SWtWPClelvinEncrnRVJhTm2NskFLnrKP12St3hdIk9yDQiOus5Bc6f55Gh1fRwtHNkkp7vZ5umm8uKw0yPGaWa2no9mWK-XEb2~K-Mg8rFJYniJzWMqd5zRgnvQhunlfPe8LKVos22I5EAyl3~jScTIB-DC76213rH74KtkLbGoU6ptaVCI9YF3GSO7tYgyxD2mVlf-2866D2qzvpsR2ELmjnDK~rlaCHGjOeGgHiTDz3xaOb0133-30wEeQVFHRpGj5~xrFHL1cY9DjRAow__&Key-Pair-Id=APKAIE5G5CRDK6RD3PGA)
Plastome organization, transcript content, and translational efficiency of rbcL in comparable light adapted leaves of physiologically analogous tobacco (wt) and tobaccoRst (tRst) plants (approximately 60 cm in height). A, The tRst transformants have the tobacco rbcL gene replaced with the corresponding gene from sunflower (rbcLS) and contain a p-aadA-t gene cassette (p, 16S rDNA rrn promoter and 5′ UTR; t, rps16 3′ UTR; Svab and Maliga, 1993) inserted 174-bp downstream of the rbcL stop codon within the rbcL 3′ UTR (T). The annealing positions of the 5′ΔrbcL probe and the primers LsE and LsH are shown. The dotted lines and numbering (Shinozaki et al., 1986) refer to positioning of the homologously inserted foreign sequence into the tobacco plastome (GenBank accession no. Z00044). P, rbcL promoter and 5′ UTR. B, Blot of total leaf RNA (the amount and relative leaf area used are shown) probed with 5′ΔrbcL, showing the rbcL produced in wt and the mono- (rbcLS) and bi- (rbcLS-aadA) cistronic mRNAs produced in the tRst plants. Equivalent loading was confirmed by comparing the amount of 16SrRNA transcript (data not shown). C, The relative abundance of the rbcL and rbcLS mRNAs measured from B and the corresponding Rubisco content measured by [14C]CABP binding are expressed as a percentage to the average rbcL transcript and Rubisco content in the wt samples. The RTE was obtained by dividing Rubisco content by transcript abundance. For tRst, the RTE in parentheses is calculated from the sum of the abundances of the rbcLS and rbcLS-aadA transcripts.
TobaccoRst Grows in Air
At ambient pCO2 (C i of approximately 350 μbar) the CO2 assimilation rates in the young fully expanded tobaccoRst leaves were approximately 3 μmol m−2 s−1, indicating photoautotrophic growth should be supported in air. When grown from seed in air the leaf tissue that developed following normal cotyledon development was necrotic, consistent with Kanevski et al. (1999). However, when grown at elevated pCO2 and then transferred to growth in air once a defined apical meristem had developed and shoot elongation was imminent, growth to fertile mature plants was possible, albeit 4-fold slower than a tobaccoRst plant maintained in air with 0.5% (v/v) CO2 (Supplemental Fig. S4). The leaves that developed in air were phenotypically distinct, being paler green, thinner, and consequently, extremely fragile.
Rubisco Content in Juvenile Leaves
![Comparison of Rubisco activase content and Rubisco production in juvenile wild-type tobacco and tobaccoRst (tRst) leaves grown in air containing 0.5% (v/v) CO2. Total and soluble protein from 0.3 cm2 of normal ovate wild-type leaves (n = 6) and the strappy oblanceolate tRst leaves (n = 4; refer to white arrows in Fig. 1, A–C) were separated by SDS-PAGE and stained with Coomassie Blue (A) or replicate samples (B) blotted and probed with antibodies raised against spinach Rubisco (Rubisco L antibody) and tobacco Rubisco activase (activase antibody). For comparison, the content of Rubisco active sites measured in the soluble leaf protein by [14C]CABP binding are shown. C, The abundance of rbcLS (black) and rbcLS-aadA (white) transcripts in the tRst samples relative to the average amount of rbcL message in wild type measured from RNA blots probed with 5′ΔrbcL (see Fig. 6B for example). The RTE was obtained by dividing Rubisco content by transcript abundance. For tobaccoRst, the RTE in parentheses is calculated from the sum of the abundances of the rbcLS and rbcLS-aadA transcripts.](https://oup.silverchair-cdn.com/oup/backfile/Content_public/Journal/plphys/146/1/10.1104_pp.107.109058/2/m_plphys_v146_1_83_f7.jpeg?Expires=1750521843&Signature=ilEEnh2WM2hHN89AcnNnRpCQQrPwXeiLfTIVrLw-~2MICbvyFnhXgcvJdQZBU5M0xx-7HhYwY1RJlEaFYex~SBsDWSTsKtv5kpWEX6EFiBA3ykOxwkPzBWzzpM-y373vxYDLp5CE3UdHuKNRBRTxU9C9j44bP~TuWz7gn77UHIwxkCAFccrg0sCZ4y~B5jIE~Bi1oNqUGyrb6LpSdzXvIDuj4JMnk7MWkl6DOqDO~36g8FM3tGffcwgTLLXtQv6J3C8YYIOs1wb8Ek3mRrL5j9lBL1p62Wpx2lP044BOjW-KfIWlNRSkkmQiKo5q0GcsXiMyU4Ovv9JBf0dGQbvJng__&Key-Pair-Id=APKAIE5G5CRDK6RD3PGA)
Comparison of Rubisco activase content and Rubisco production in juvenile wild-type tobacco and tobaccoRst (tRst) leaves grown in air containing 0.5% (v/v) CO2. Total and soluble protein from 0.3 cm2 of normal ovate wild-type leaves (n = 6) and the strappy oblanceolate tRst leaves (n = 4; refer to white arrows in Fig. 1, A–C) were separated by SDS-PAGE and stained with Coomassie Blue (A) or replicate samples (B) blotted and probed with antibodies raised against spinach Rubisco (Rubisco L antibody) and tobacco Rubisco activase (activase antibody). For comparison, the content of Rubisco active sites measured in the soluble leaf protein by [14C]CABP binding are shown. C, The abundance of rbcLS (black) and rbcLS-aadA (white) transcripts in the tRst samples relative to the average amount of rbcL message in wild type measured from RNA blots probed with 5′ΔrbcL (see Fig. 6B for example). The RTE was obtained by dividing Rubisco content by transcript abundance. For tobaccoRst, the RTE in parentheses is calculated from the sum of the abundances of the rbcLS and rbcLS-aadA transcripts.
DISCUSSION
TobaccoRst Can Grow Autotrophically
We have shown using tobaccoRst and independently generated tRstLA transplastomic lines that tobacco only expressing a hybrid LsSt enzyme comprising sunflower L and tobacco S subunits can be grown autotrophically past cotyledon emergence to fertile maturity in air when supplemented with CO2 (Fig. 1; Supplemental Fig. S1). Both photosynthetic gas-exchange measurements on mature leaves and kinetic measurements made on extracted LsSt showed that the catalytic properties of the hybrid enzyme are comparable to the native sunflower and tobacco Rubiscos (Fig. 2B). However, even when supplied with 0.5% CO2 (v/v) the tobaccoRst line and the tRstLA lines display atypical developmental phenotypes and slower growth.
The Sustained Kinetics of the Hybrid LsSt Enzyme Is Unique
Previous mutagenic studies using cyanobacterial and Chlamydomonas Rubiscos have clearly shown the S subunits are required for catalytic competency, have a pervasive influence on the kinetic properties of Rubisco, and that the L subunits are catalytically impaired when assembled with heterologous or structurally altered S subunits (Spreitzer, 2003; Karkehabadi et al., 2005). This is not the case for LsSt, invalidating the canonical belief that the assembly of heterologous Rubisco L and S subunits will unfavorably influence catalytic efficiency. Indeed, although only sharing 76% sequence identity we can only assume from the sustained kinetics of LsSt that there is sufficient similarity in the quaternary structure of the assembled tobacco S subunits so as to retain the normal active site geometry of the assembled sunflower L subunits. This raises the interesting possibility that there may be greater structural flexibility between heterologous Rubisco S and L peptides from higher plants that enables their assembly with little or no kinetic perturbation to the hybrid enzyme. Addressing this possibility will necessitate comparable tranplastomic studies focused on replacing the tobacco rbcL with other plant rbcL variants.
Our in vitro measurements of V c max were made using rapidly isolated protein extracts from leaves from both tissue-culture and autotrophically grown LsSt plants using different extraction buffers containing different protease inhibitor additives without appreciable decline in activity after 30 min incubation at 25°C (Supplemental Fig. S3). This use of rapidly isolated leaf protein extract is now commonplace within this laboratory and gives accurate, and reproducible, estimates of V c max (Whitney et al., 2001; Ghannoum et al., 2005) and also K c whose values match those obtained previously using freshly purified Rubisco (Fig. 2B). Possibly the purified LsSt used by Kanevski et al. (1999) to measure its kinetics had been compromised by partial proteolysis of terminal residues during the extended purification process. Proteolysis of N- and/or C-terminal residues may explain their lower V c max (Fig. 2B) and high K c (60 μ m) measurements for LsSt as these kinetic parameters (and to a much lesser extent Sc/o) have also been strongly compromised in R. rubrum, cyanobacteria, and spinach (Spinacia oleracea) Rubiscos upon removal of residues from their L subunit termini by partial proteolysis or mutagenesis (Gutteridge et al., 1986a; Kettleborough et al., 1987; Ranty et al., 1990).
Rubisco Activity Limits TobaccoRst Growth
Our results indicate the efficiency of the photosystems was uncompromised in tobaccoRst at their growth illumination as their chlorophyll content, a/b ratio, and F v/F m ratio mirrored that observed for wild type. It is more likely the primary basis for the developmental differences resulted from limitations in LsSt production. Development was particularly perturbed during juvenile growth when LsSt levels were reduced more than 7-fold, resulting in the production of unique oblanceolate leaf clusters not seen previously in anti-RbcS tobacco lines where Rubisco production was reduced 5-fold (Tsai et al., 1997), suggesting greater limitations to source strength in tobaccoRst. With maturation, the leaf LsSt content improved to levels approximately 20% that of Rubisco in wild-type tobacco, however, the photosynthetic capacity of tobaccoRst remained Rubisco activity limited despite CO2 supplementation (Fig. 3). This limitation was substantiated by an approximately 2-fold lower content of nonstructural carbohydrate, an approximately 9-fold higher ribulose-P2 to P-glycerate ratio, and high nonphotochemical quenching up to a intracellular pCO2 (Cc) of 2 mbar (Supplemental Fig. S2), and the ineffectual response of CO2 assimilation rate to illumination above the growth intensity of 350 μmol quanta m−2 s−1 for tobaccoRst (Fig. 2A). Consistent with the differences in their exponential growth rates (Fig. 1D) the predicted Rubisco limited CO2 assimilation rate at the growth pCO2 (approximately 5 mbar) in fully expanded tobaccoRst leaves (containing 5 μmol Rubisco active sites m−2) modeled according to Farquhar et al. (1980) is 14 μmol CO2 m−2 s−1, which is approximately two-thirds that of the light limited assimilation rate in wild-type tobacco (23 μmol CO2 m−2 s−1; Fig. 2A). Also consistent with leaf gas-exchange measurements was the capacity for tobaccoRst to grow without CO2 enrichment, albeit only once source strength and leaf LsSt content were adequate. This necessitated the development of normal ovate leaves before growth through to reproductive maturity could be supported in air, albeit approximately 4-fold slower than if maintained at high pCO2 (Supplemental Fig. S4).
What Is Limiting the Content of LsSt in TobaccoRst Leaves?
While the comparable stability of the tobaccoRst and wild-type tobacco Rubiscos indicated little or no perturbations to turnover of the assembled LsSt hexadecamer (Fig. 4), reductions in the relative RNA content per leaf area and in the steady-state pool of the sunflower rbcLS and rbcLS-aadA mRNAs contributed to the paucity of LsSt produced. Compared with the rbcL pool in tobacco, the abundance of both the rbcLS and dicistronic rbcLS-aadA transcripts in juvenile and mature tobaccoRst leaves were approximately 5- and 4-fold lower, respectively. The stability of chloroplast transcripts are strongly influenced by appropriate endo- and exonucleolytic maturation of the 5′- and 3′-untranslated regions (UTRs; Bollenbach et al., 2004). The sequence of these regions, and that of the juxtaposed coding sequence, strongly influence appropriate mRNA folding and interaction with nucleus encoded chloroplast RNA-binding complexes that maintain the integrity of the transcript by correctly processing the 5′ and 3′ ends to prevent degradation by nucleases (Monde et al., 2000; Bollenbach et al., 2004; Zicker et al., 2007). As rbcLS expression in tobaccoRst is controlled by the same promoter and 5′ UTR as tobacco rbcL it is likely changes to the 3′ UTR and rbcLS coding sequences perturbed proper maturation and stabilization of the transcripts making them more susceptible to degradation by plastid ribonucleases. The importance of the tobacco rbcL 3′ UTR for maintaining steady-state levels of rbcL mRNA has been shown previously (Whitney and Andrews, 2003). In tobaccoRst the aadA cassette is inserted at the equivalent position 178-bp downstream of the rbcL stop codon after nucleotide 59251 in the tobacco plastome (GenBank accession no. Z00044). Intriguingly in subsequent RNA blots using fully denaturing formaldehyde gels we find the rbcLS mRNA is smaller than the native rbcL mRNA, indicating the mature 3′ UTR of rbcL extends further downstream (data not shown). In support of this, insertion of a promoterless aadA gene a further 91-bp 3′ to that in tobaccoRst does not perturb rbcL abundance or Rubisco production and still enables transcription of an additional, less abundant (approximately 20% of the rbcL mRNA) rbcL-aadA transcript (Whitney et al., 1999; Whitney and Andrews, 2003). Clearly, the 3′ UTR does have a pervasive influence on the steady-state levels of chloroplast mRNAs and to what extent lengthening the 3′ UTR would improve rbcLS transcript abundance, and reduce transcriptional read through of the rbcLS-aadA message, remains to be examined.
Problems with translation and/or assembly also contributed to the paucity of LsSt produced in the tobaccoRst leaves. Frequently in plastome transformation studies the equipping of foreign gene sequences with nonnative UTR sequences can perturb mRNA folding and slow translational processing of transgenes (Maliga, 2002). This necessitates the trialing of different UTR combinations to optimize expression (Maliga, 2003). The RTE of both rbcLS mRNAs in both the juvenile and mature tobaccoRst leaves was reduced 30% to 50%. One possible reason for this difference is the levels of rbcL and Rubisco holoenzyme during leaf ontogeny may differ between tobaccoRst and wild type. That is, despite sampling expanding leaves from a comparable position in the canopy from wild type and tobaccoRst of similar heights, their apparent developmental differences (Fig. 1) might extend to variations in the patterns of rbcL and Rubisco contents during leaf development that are otherwise generally correlated in tobacco (Miller et al., 2000) but not in all plants (see Suzuki et al., 2001 and refs. therein). Clearly this needs to be examined more comprehensively in the future by profiling the variation in plastome copy number, rbcL, RbcS, and Rubisco contents, and the rates of subunit translation (using rapid 35S-Met pulse methods; Rodermel et al., 1996) in both tobacco and tobaccoRst leaves during their development.
The reduced RTE of rbcLS mRNAs in tobaccoRst might otherwise arise from problems related to translational processing of the transcripts due to the introduced NheI cloning site in codons 9 and 10. This introduces changes to nucleotides 27 and 30 in rbcLS that otherwise shows absolute homology to tobacco rbcL for the first 56 nucleotides (GenBank accession no. AF097517). Unfortunately these nucleotide changes may potentially hamper translational processing of rbcLS since the translational control region of rbcL includes both the 5′ UTR and N-terminal coding sequence (approximately 42 nucleotides; Kuroda and Maliga, 2001). By examining the sedimentation behavior of the polysomes associated with the rbcLS mRNA it should be possible to identify whether translation initiation or translation elongation is impeded (Kim and Mullet, 1994). Alternatively, truncation of the mRNA 3′ UTR may affect interactions between the 5′ UTR and 3′ UTR that are required for efficient recycling of mRNAs during translation (Kawaguchi and Bailey-Serres, 2002).
The lower RTE of the rbcLS transcripts in mature tobaccoRst leaves may also stem from limitations on the functional assembly of the LsSt hexadecamer. This limitation may arise from incompatibilities between tobacco chloroplast chaperone complexes and the folding and assembly requirements of sunflower L, or be indicative of reduced chaperone availability in response to elevated metabolic stress. Indeed chaperone incompatibility problems preclude assembly of functional form I Rubiscos from nongreen algae in tobacco chloroplasts (Whitney et al., 2001). However, unlike nongreen algal Rubisco subunits, no misfolded, unassembled sunflower L were detected, possibly due to their rapid removal along with the unassembled tobacco S by chloroplast proteases. Improvements in foreign Rubisco assembly might therefore be attained by cotransplanting in appropriate chaperones with their cognate Rubisco L subunits. In favor of this, the production of correctly folded cuboid crystals of the insecticidal Bacillus thuringiensis Cry protein in tobacco plastids required cotransplanting of a complementary chaperone (De Cosa et al., 2001). Despite the complexity of Rubisco assembly in chloroplasts, candidate chaperones that specifically interact with Rubisco L subunits have been identified and may provide useful starting points. These chaperones include the BSDII chaperone protein that shares homology with DnaJ chaperones (Brutnell et al., 1999) and RbcX-like homologs that play an important role in promoting assembly of cyanobacterial L8 cores in E. coli by forming dynamic intermediate complexes with L subunits after having undergone folding by the chaperonins (Saschenbrecker et al., 2007).
The Hybrid Rubisco Is Adequately Regulated by Tobacco Rubisco Activase
The compatibility problems that can potentially limit the regulatory capacity of a Rubisco activase to Rubiscos from related species (Wang et al., 1992) did not affect the capacity of tobacco activase to adequately regulate LsSt. This was initially evident from the approximately 2-fold higher carbamylation status of the hybrid Rubisco. As seen in transplastomic tobacco lines producing 50% less Rubisco (Whitney and Andrews, 2003) or a kinetically inferior mutant (Whitney et al., 1999), the high carbamylation status presumably compensates for the lower carboxylation potential. Light transient gas-exchange analyses also showed the activation constant for LsSt was 25% faster than wild-type tobacco Rubisco, indicating the hybrid enzyme was adequately regulated by tobacco Rubisco activase (Fig. 4). Possibly this faster activation occurred as a consequence of the 4- to 7-fold (depending on leaf age) higher stoichiometry of activase to Rubisco in the tobaccoRst leaves that resulted from a concomitant reduction in LsSt levels but no discernable change in activase production. This higher stoichiometry might also conceal regulatory incompatibilities between LsSt and tobacco Rubisco activase that will need to be resolved using appropriate in vitro enzyme assays (Wang et al., 1992), particularly if efforts are undertaken to increase production of LsSt in tobacco chloroplasts.
Reasons for the Abnormal TobaccoRst Leaf Phenotype Are Unclear
As the developmental phenotype of tobaccoRst leaves has been maintained over three generations after backcrossing with wild-type pollen and is repeated in the independently generated tobRstLA7 and tobRstLA13 transplastomic lines (Supplemental Fig. S1), the phenotype is not caused by pleiotropic effects and results from the replacement of tobacco L with the sunflower L. More comprehensive developmental studies on tobaccoRst and the tobRstLA lines are needed to uncover the underlying cause(s) of its aberrant phenotypic features. While sharing developmental similarities to anti-RbcS and other Calvin cycle antisense mutants with reduced carbon assimilation capacity (Tsai et al., 1997; Raines and Paul, 2006), the unique production of leaf clusters and multiple shoots early in tobaccoRst development and the marginal dimpling of mature leaves indicate their development process is more severely perturbed. Interestingly, juvenile tobacco-rubrum plants do not show this phenotype when grown in air containing 1% or 2.5% CO2 (v/v), suggesting their rates of carbon assimilation are sufficiently nonlimiting at these high pCO2. However, at 1% CO2 some tobacco-rubrum leaves display slight marginal dimpling early in development that disappears as the leaves age and accumulate more Rubisco (data not shown). Whether the developmental anomalies of tobaccoRst can be circumvented by growth under non-Rubisco limiting conditions (i.e. higher pCO2, lower light) have yet to be examined. Nevertheless, it is important to note that when grown in tissue culture on media containing Suc, tobaccoRst only produces normal leaves consistent with limitations in carbon assimilation being the primary cause of the developmental differences during autotrophic growth.
Possibly the developmental differences of tobaccoRst arise from greater limitations in carbohydrate production than in other anti-Calvin cycle mutants, particularly in the juvenile tissue where survival past cotyledon emergence necessitates CO2 supplementation. Very low Rubisco levels would impose significant limitations on the acquisition of sufficient source strength, explaining the lengthy duration of the juvenile phase in tobaccoRst (30–40 d) and phenotypic abnormalities that possibly arise from alterations in the developmental program of the shoot apical meristems in response to developmental delay. As shoot apical meristems regulate the development of lateral organs such as leaves and branches (Piazza et al., 2005), it is possible some (or all) of the multiple apical shoots produced by tobaccoRst may really be lateral branches. Clearly a more thorough growth comparison of tobaccoRst with anti-RbcS tobacco (‘Petit Havana’) with comparable Rubisco reductions (particularly in juvenile leaves) is needed to identify differences in their development, carbon-nitrogen status, and metabolite profiles (Fritz et al., 2006) and improve our understanding of the regulatory signals derived from changes in leaf metabolism that influence a plant's developmental program (Raines and Paul, 2006).
Future Considerations for Rubisco Engineering in Tobacco Chloroplasts
While many unresolved problems associated with transplanting foreign Rubiscos into higher plant plastids remain to be addressed, our discovery that LsSt is not catalytically impaired should motivate further plastomic replacement studies with foreign Rubisco L subunits, particularly those from other higher plants. As highlighted in this study, success will necessitate the introduced rbcL to be stably transcribed, efficiently translated, and the folding and assembly requirements of the L be sufficiently compatible with the tobacco chaperone complexes and the tobacco S and suitably regulated with the endogenous Rubisco activase. Only needing to engineer rbcL would be advantageous as it would negate the need for cotransplanting in the complementary RbcS and evade further obstacles with needing to silence the endogenous S or genetically manipulate it as this is complicated by the multiple RbcS copies in the nuclear genome (Andrews and Whitney, 2003). Candidate rbcL genes for testing would include those of the kinetically superior Rubiscos from C3 plants growing in hot arid conditions (Galmes et al., 2005), such as Limonium gibertii (Parry et al., 2007) whose L shows 92% identity to tobacco L. A wider examination might also include rbcL genes from C4 Rubiscos that characteristically have higher k c cat values relative to C3 species, albeit at the expense of higher K c values (Yeoh et al., 1981; Seemann et al., 1984).
MATERIALS AND METHODS
Materials
Substrate ribulose-P2 (both 1 3H labeled and unlabeled) was synthesized according to Kane et al. (1998). Unlabelled ([12C]) and carboxyl-14C-labeled ([14C]) carboxypentitol-P2 (an isomeric mixture of carboxyarabinitol-P2 and carboxyribitol-P2) were synthesized as described in Pierce et al. (1980). The carboxyarabinitol-P2, that binds stoichiometrically to CO2-Mg2+ activated Rubisco active sites almost irreversibly (Schloss, 1988) was purified from these preparations by ion exchange (Zhu and Jensen, 1991).
Plant Growth
Tobacco (Nicotiana tabacum L. ‘Petit Havana’ [N,N]) and transplastomic tobaccoRst (line Nt-pIK 83-1; Kanevski et al., 1999; T0 seed obtained from Pal Maliga) were germinated and grown in soil in a controlled environment cabinet with a 14 h light (25°C) and 10 h dark (20°C) photoperiod in air supplemented with 0.5% (v/v) CO2 during the light period where the artificial illumination was 350 μmol quanta m−2 s−1 and the humidity set at 65%. Each generation of tobaccoRst was artificially pollinated with wild-type pollen and the T2 seed used for all analyses. The plants were grown in 5-L pots of soil and provided with Hoagland nutrient mix (Hoagland and Snyder, 1933) every 2 d. The height of the plants was measured every 2 to 3 d from the soil surface to the apical meristem of the primary axial shoot until the emergence of the first floral apertures.
Leaf Gas Exchange
Leaf Sampling, Metabolite, and Biochemical Measurements
Biochemical measurements were made on leaf samples taken 8 h into the 14 h photoperiod from either developing juvenile leaf tissue or from young expanding mature leaves at comparable positions in the canopy (typically the fifth leaf below the apical meristem) from plants during the fast growth phase that were either approximately 35 or 60 cm tall. For the mature leaves, samples were taken from the same leaf and frozen immediately in liquid nitrogen and stored at −80°C or dried at 80°C for elemental nitrogen and carbon content analysis as described in Whitney et al. (2001b). Stored leaf samples were used to measure Rubisco content and carbamylation status using the [14C]carboxyarabinitol-P2 binding/[12C]carboxyarabinitol-P2 exchange procedure (Ruuska et al., 1998; Whitney and Andrews, 2001b) and the content of nonstructural starch, chlorophyll, soluble protein, ribulose-P2, and P-glycerate measured according to Whitney and Andrews (2003).
The substrate saturated turnover rate (V c max) and K m for CO2 at ambient pO2 (K c 21%O 2) for Rubisco were measured using the soluble protein rapidly extracted on ice from 1 cm2 leaf discs using glass homogenizors (Wheaton) into 0.8 mL CO2-free extraction buffer (50 mm Hepps-NaOH, pH 8.0, 1 mm EDTA, 2 mm dithiothreitol, 1% [v/v] plant protease inhibitor cocktail [Sigma-Aldrich], and 1% [w/v] polyvinylpolypyrrolidone). A sample of lysate was taken for SDS-PAGE analysis as described (Whitney et al., 2001) and the remainder centrifuged (14,000g, 1 min, 4°C) and samples of the soluble protein taken for SDS-PAGE or incubated (activated) with NaHCO3 and MgCl2 (15 mm each) for 10 min and used to: measure Rubisco content in duplicate aliquots incubated with 10 or 30 μ m of [14C]CABP and the amount of Rubisco-bound [14C]CABP recovered by gel filtration (Ruuska et al., 1998; Whitney and Andrews, 2001b), or used to measure K c 21%O 2 at 25°C, pH 8.0 using 14CO2 fixation assays as described in Whitney and Sharwood (2007). The K c assays were initiated by adding the activated soluble protein extract into septum capped scintillation vials containing CO2-free air equilibrated assay buffer (100 mm Hepps-NaOH, 15 mm MgCl2, 0.6 mm ribulose-P2, 0.1 mg mL−1 carbonic anhydrase) containing 0 to 44 μ m 14CO2. The assays were stopped after 1 min with 0.5 volumes of 25% (v/v) formic acid and processed for scintillation counting (Whitney and Sharwood, 2007). Measurements of V c max were calculated using comparable 14CO2 fixation assays containing 15 mm NaH14CO3 and dividing the rate by the concentration of Rubisco active sites measured by [14C]carboxyarabinitol-P2 binding (see above).
Rubisco Purification and CO2/O2 Specificity
Rubisco was rapidly purified from approximately 50 g of freshly harvested leaves of tobacco, tobaccoRst, and sunflower (Helanthus annuus) plants grown at high CO2. The leaves were homogenized in 40 mL ice cold extraction buffer, filtered through five layers of miracloth, centrifuged (30,000g, 10 min, 2°C), and the soluble protein chromatographed on a 5 mL Q-sepharose column equilibrated with column buffer (50 mm Hepps-NaOH, pH 8.0, 1 mm EDTA). Proteins were eluted over a 0 to 400 mm NaCl gradient over 150 mL (5 mL min−1 flow rate) and the two fractions (5 mL) containing peak Rubisco activities (measured by ribulose-P2-dependent 14CO2 fixation) were pooled and dialyzed for 16 h at 4°C against 1 L of storage buffer (25 mm Hepps-NaOH, pH 8.0, 1 mm EDTA, 20% [v/v] glycerol). This concentrated the samples approximately 3-fold before they were frozen in liquid nitrogen and stored at −70°C. The purified Rubisco preparations were used to measure their CO2/O2 specificity (Sc/o) using the method of Kane et al. (1994).
SDS-PAGE and Immunoblots
Leaf protein samples were separated by SDS-PAGE (Bis-Tris-buffered 4%–12% NuPAGE gels, Invitrogen), blotted onto nitrocellulose, probed with appropriate antibodies, and the immunoreactive bands visualized using AttoPhos (Promega) as described previously (Whitney and Andrews, 2001a).
35S-Pulse Chase Labeling
Pulse chase labeling of leaf proteins was performed at 25°C using a modified protocol described by Whitney and Andrews (2001a). Thirty leaf discs (1 cm2) were collected in the growth chamber from young fully expanded leaves and transferred to the laboratory in covered dishes onto prewetted Whatman paper. Within 5 min the discs were layered abaxial side down onto pulse solution (Murashige and Skoog nutrient solution [pH 7.2]; Murashige and Skoog, 1962) containing 10 mm NaHCO3, 0.25% (v/v) silwet, and 2% (v/v) [35S]Met (10.5 mCi/mL; ICN Biomedicals) and vacuum infiltrated at 200 mbar for 4 min before slowly releasing the vacuum. After 20 min illumination (600 μmol quanta m−2 s−1) the discs were quickly rinsed with three 100 mL washes of chase solution (pulse solution containing 10 mm Met) with intermittent blotting on Whatman paper to remove excess solution. Three leaf discs were snap frozen in liquid nitrogen (0 h chase samples) and the remainder layered onto 50 mL chase solution, vacuum infiltrated, and illuminated a further 0.5, 1, or 2 h before freezing in liquid nitrogen. The frozen discs were extracted in 0.5 mL extraction buffer on ice in Wheaton glass homogenizors then centrifuged (35,000g, 10 min, 4°C) and the supernatant diluted 9:1 with native load buffer (60% [v/v] glycerol, 1% [w/v] bromphenol blue) and the proteins loaded onto Tris-Glycine buffered 4% to 20% NuPAGE gels and separated at 60 V for 18 h at 4°C in nondenaturing running buffer (19.2 mm Gly, 2.4 mm Tris, pH 8.3). The separated proteins were fixed in the gels and stained with Gel Code Blue Coomassie stain (Pierce) according to manufacturer instructions before vacuum drying the gels and exposing them to a storage phosphor screen for 21 d. The radioactive signals were detected using a Molecular Dynamics 400-B 2D PhosphorImager and the banding quantified by densitometry analysis using ImageQuant software.
Leaf DNA, RNA, and PCR Analyses
Total leaf DNA and RNA was extracted, separated by agarose gel electrophoresis, and blotted onto membranes as described previously (Whitney et al., 1999, 2001). The membranes were prehybridized at 55°C for at least 1 h in hybridization buffer supplied in the AlkPhos Direct Labeling kit (Promega) before hybridizing with DNA probes labeled with α-[32P]dATP using the MegaPrime Labeling kit (GE Biosciences). For RNA blots the 575-bp tobacco 5′ΔrbcL probe (Fig. 6A), which spans the tobacco rbcL promoter, 5′ UTR, and the first 42 nucleotides of the tobacco rbcL gene, was amplified from tobacco DNA using the primers LsH (5′-CTATGGAATTCGAACCTGAACTT-3′) and NdeHis-15 (5′-CCATATGTTTGAATCCAACACTTGCTTTA-3′). The DNA blots were hybridized with the 1,856-bp DNA rbcL probe as described (Whitney and Andrews, 2001b; Supplemental Fig. S1). The autoradiograph signals were detected on storage phosphor screens as described above.
Total leaf DNA from tobaccoRst transplastomic plants was used as template for PCR amplification using primers LsE (Whitney and Andrews, 2001b) and LsH (Fig. 6A). The 2.6 kb amplified product was cloned into pGEM-T Easy (Promega) and fully sequenced using BigDye terminator sequencing (Applied Biosystems) on an ABI 3730 sequencer (Biomolecular Resource Facility, John Curtin School of Medical Research, Australian National University).
Supplemental Data
The following materials are available in the online version of this article.
Supplemental Figure S1. The genotype and phenotype of new tRstLA (tobaccoRst-Lox-aadA) transplastomic lines.
Supplemental Figure S2. Measurement of electron transport rate, nonphotochemical quenching (NPQ), and CO2 assimilation rate in response to change in intercellular pCO2 using simultaneous leaf chlorophyll fluorescence and CO2 assimilation measurements.
Supplemental Figure S3. Change in maximal ribulose-P2-dependent carboxylase activity (V c max) in leaf protein extracts from tissue-cultured plants in response to CO2-Mg2+ activation during incubation at 25°C.
Supplemental Figure S4. Growth of tobaccoRst in air.
ACKNOWLEDGMENTS
We thank Archie Portis for his helpful comments on the manuscript and John Andrews for his contribution to the research.
LITERATURE CITED
Andrews TJ, Whitney SM (
Bollenbach TJ, Schuster G, Stern DB (
Brooks A, Farquhar GD (
Brutnell TP, Sawers RJH, Mant A, Langdale JA (
De Cosa B, Moar W, Lee SB, Miller M, Daniell H (
Dhingra A, Portis AR, Daniell H (
Farquhar GD, von Caemmerer S, Berry JA (
Fritz C, Mueller C, Matt P, Feil R, Stitt M (
Galmes J, Flexas J, Keys AJ, Cifre J, Mitchell RAC, Madgwick PJ, Haslam RP, Medrano H, Parry MAJ (
Ghannoum O, Evans JR, Chow WS, Andrews TJ, Conroy JP, von Caemmerer S (
Gutteridge S, Millard BN, Parry MAJ (
Gutteridge S, Parry MAJ, Burton S, Keys AJ, Mudd A, Feeney J, Servaites JC, Pierce J (
Hammond ET, Andrews TJ, Mott KA, Woodrow IE (
Hoagland DR, Snyder WC (
Hudson GS, Evans JR, von Caemmerer S, Arvidsson YBC, Andrews TJ (
Kane HJ, Viil J, Entsch B, Paul K, Morell MK, Andrews TJ (
Kane HJ, Wilkin JM, Portis AR, Andrews TJ (
Kanevski I, Maliga P (
Kanevski I, Maliga P, Rhoades DF, Gutteridge S (
Karkehabadi S, Peddi SR, Anwaruzzaman M, Taylor TC, Cederlund A, Genkov T, Andersson I, Spreitzer RJ (
Kawaguchi R, Bailey-Serres J (
Kebeish R, Niessen M, Thiruveedhi K, Bari R, Hirsch H, Rosenkranz R, Stabler N, Schonfeld B, Kreuzaler F, Peterhansel C (
Kettleborough CA, Parry MAJ, Burton S, Gutteridge S, Keys AJ, Phillips AL (
Kim J, Mullet JE (
Kuroda H, Maliga P (
Maliga P (
Maliga P (
Miller A, Schlagnhaufer C, Spalding M, Rodermel S (
Monde RA, Greene JC, Stern DB (
Murashige T, Skoog F (
Parry MAJ, Madgwick PJ, Carvalho JFC, Andralojc PJ (
Piazza P, Jasinski S, Tsiantis M (
Pierce J, Tolbert NE, Barker R (
Raines C, Paul M (
Raines CA (
Ranty B, Lundqvist T, Schneider G, Madden M, Howard R, Lorimer G (
Rodermel S (
Rodermel S, Haley J, Jiang CZ, Tsai CH, Bogorad L (
Rodermel SR, Abbott MS, Bogorad L (
Ruuska S, Andrews TJ, Badger MR, Hudson GS, Laisk A, Price GD, von Caemmerer S (
Saschenbrecker S, Bracher A, Rao KV, Rao BV, Hartl FU, Hayer-Hartl M (
Schloss JV (
Seemann JR, Badger MR, Berry JA (
Shinozaki K, Ohme M, Tanaka M, Wakasugi T, Hayashida N, Matsubayashi T, Zaita N, Chunwongse J, Obokata J, Yamaguchi-Shinozaki K, et al (
Spreitzer RJ (
Suzuki Y, Makino A, Mae T (
Svab Z, Maliga P (
Tsai CH, Miller A, Spalding M, Rodermel S (
von Caemmerer S, Evans JR, Hudson GS, Andrews TJ (
Wang Z-Y, Snyder GW, Esau BD, Portis AR Jr, Ogren WL (
Whitney SM, Andrews TJ (
Whitney SM, Andrews TJ (
Whitney SM, Andrews TJ (
Whitney SM, Baldet P, Hudson GS, Andrews TJ (
Whitney SM, Sharwood RE (
Whitney SM, von Caemmerer S, Hudson GS, Andrews TJ (
Wingler A, Lea PJ, Quick WP, Leegood RC (
Woodrow IE, Mott KA (
Yeoh H-H, Badger MR, Watson L (
Zhang XH, Ewy RG, Widholm JM, Portis AR Jr (
Zhu G, Jensen RG (
Zhu XG, Portis AR, Long SP (
Zicker A, Kadakia C, Herrin D (
Author notes
This work was supported by a Discovery grant (no. DP0450564) awarded to S.M.W. by the Australian Research Council. Research in P.M.'s laboratory was supported by the National Science Foundation (grant no. MCB 93–05037).
Corresponding author; e-mail [email protected].
The author responsible for distribution of materials integral to the findings presented in this article in accordance with the policy described in the Instructions for Authors (www.plantphysiol.org) is: Spencer Michael Whitney ([email protected]).
The online version of this article contains Web-only data.
Open Access articles can be viewed online without a subscription.