-
PDF
- Split View
-
Views
-
Cite
Cite
Ruiqi Li, Zhifeng Wei, Yan Li, Xudong Shang, Ying Cao, Liusheng Duan, Ligeng Ma, SKI-INTERACTING PROTEIN interacts with SHOOT MERISTEMLESS to regulate shoot apical meristem formation, Plant Physiology, Volume 189, Issue 4, August 2022, Pages 2193–2209, https://doi.org/10.1093/plphys/kiac241
- Share Icon Share
Abstract
The shoot apical meristem (SAM), which is formed during embryogenesis, generates leaves, stems, and floral organs during the plant life cycle. SAM development is controlled by SHOOT MERISTEMLESS (STM), a conserved Class I KNOX transcription factor that interacts with another subclass homeodomain protein, BELL, to form a heterodimer, which regulates gene expression at the transcriptional level in Arabidopsis (Arabidopsis thaliana). Meanwhile, SKI-INTERACTING PROTEIN (SKIP), a conserved protein in eukaryotes, works as both a splicing factor and as a transcriptional regulator in plants to control gene expression at the transcriptional and posttranscriptional levels by interacting with distinct partners. Here, we show that, similar to plants with a loss of function of STM, a loss of function of SKIP or the specific knockout of SKIP in the SAM region resulted in failed SAM development and the inability of the mutants to complete their life cycle. In comparison, Arabidopsis mutants that expressed SKIP specifically in the SAM region formed a normal SAM and were able to generate a shoot system, including leaves and floral organs. Further analysis confirmed that SKIP interacts with STM in planta and that SKIP and STM regulate the expression of a similar set of genes by binding to their promoters. In addition, STM also interacts with EARLY FLOWERING 7 (ELF7), a component of Polymerase-Associated Factor 1 complex, and mutation in ELF7 exhibits similar SAM defects to that of STM and SKIP. This work identifies a component of the STM transcriptional complex and reveals the mechanism underlying SKIP-mediated SAM formation in Arabidopsis.
Introduction
The aerial tissues and organs of angiosperms, including leaves, stems, and flowers, are initiated through the activity of the shoot apical meristem (SAM), which generates organs in a predictable and regular pattern (Scheres, 2007; Dinneny and Benfey, 2008). The SAM is a highly organized group of cells that can be divided into distinct zones with different functions (Hake et al., 2004; Scheres, 2007). These functional zones include the central zone (CZ), which contains a pool of slowly dividing pluripotent stem cells called the stem cell niche; the peripheral zone (PZ), which surrounds the CZ and in which cells divide rapidly to generate leaf primordia; and the rib zone, which lies below the CZ and contains cells that divide to form stems (Scheres, 2007; Dinneny and Benfey, 2008). Apart from this functional classification, the SAM is organized into three clonally distinct layers of cells. The cells in the outermost L1 layer and the subepidermal L2 layer divide in an anticlinal manner, while the underlying corpus forms a multilayered structure in which cells divide in random orientations (Weigel and Jurgens, 2002). Therefore, SAM formation in angiosperms requires a precise balance in cell division patterns among cells that belong to distinct zones and clonal layers (Williams, 2021).
The organization of these distinct zones and cell layers is maintained by the mobile homeodomain protein SHOOT MERISTEMLESS (STM) in Arabidopsis (Arabidopsis thaliana) (Long et al., 1996; Tsuda and Hake, 2015). STM is a Class I KNOX transcription factor, part of a small family of three amino acid loop extension (TALE) homeobox proteins. There are four Class I members in Arabidopsis: STM, Knotted-1-like 1 (KNAT1), also called BREVIPEDICELLUS, KNAT2, and KNAT6 (Hake et al., 2004; Hay and Tsiantis, 2010). They share similar expression patterns and are broadly expressed in the SAM with enrichment in certain regions, including the organ boundary. Nevertheless, STM plays a dominant role in maintaining SAM activity (Long et al., 1996). Repression of STM expression outside of the SAM is important for lateral organ formation, and is redundantly controlled by ASYMMETRIC LEAVES 1 (AS1), AS2, POLYCOMB REPRESSIVE COMPLEX 1 (PRC1)/PRC2, YABBY, and the phytohormone auxin (Byrne et al., 2000; Kumaran et al., 2002; Guo et al., 2008; Xu and Shen, 2008; Chung et al., 2019). A defect in STM expression within the SAM region or ectopic expression of STM outside of the SAM region causes defects in SAM formation and in the determination of cell fate and organ shape (Hake et al., 2004). STM is also required for axillary meristem initiation and branching in plants (Long and Barton, 2000; Shi et al., 2016; Zhang et al., 2018; Cao et al., 2020).
STM interacts with several members of another subclass of TALE homeodomain proteins called BELL family proteins to form heterodimers that activate or repress the expression of target genes at the transcriptional level (Cole et al., 2006; Hay and Tsiantis, 2010). STM activity can be controlled by regulating the translocation of STM from the nucleus to the cytosol (i.e. altering its accumulation in the nucleus) or by regulating the intercellular trafficking of STM, which alters its availability for protein–protein interactions (Balkunde et al., 2017). Several STM-interacting proteins that are required for the translocation of STM from the nucleus to the cytosol and for the intercellular trafficking of STM have been identified. They include BELL, which mediates STM nuclear import through the formation of a STM–BELL heterodimer (Cole et al., 2006); OVATE FAMILY PROTEIN and CHROMOSOMAL REGION MAINTENANCE 1, which are required for the transportation of STM from the nucleus to the cytosol (Hackbusch et al., 2005; Rutjens et al., 2009); FT INTERACTING PROTEIN (FTIP)3 and FTIP4, which are responsible for translocating STM from the plasma membrane to the nucleus by protecting STM from binding to the plasma membrane (Liu et al., 2018); and the microtubule-associated protein MOVEMENT PROTEIN 30 BINDING PROTEIN 2C and poly (ADP-ribose) polymerase domain-containing protein TWISTED-LEAF 1, which mediate the intercellular trafficking of STM via plasmodesmata (Winter et al., 2007; Xu et al., 2011; Cui et al., 2019). Thus, STM is a mobile transcription factor that regulates the expression of genes required for SAM formation and whose activity is regulated by its interacting partner proteins.
SKI-INTERACTING PROTEIN (SKIP) is a conserved protein from yeast to plants and metazoa (Folk et al., 2004; Wang et al., 2012). It is a component of the spliceosome and is required for the alternative splicing of pre-mRNAs in yeast and human cells (Wahl et al., 2009; Wan et al., 2020). In plants, SKIP works as a bifunctional factor (i.e. a splicing factor and a transcriptional regulator) to regulate gene expression at both the transcriptional and posttranscriptional levels (Cao and Ma, 2019). It integrates into the spliceosome by interacting with components of the spliceosome, including MOS4-Associated Complex 3 (MAC3) (Palma et al., 2007; Li et al., 2019), Spliceosomal Timekeeper Locus 1 (STIPL1) (Jones et al., 2012; Li et al., 2019), and Pleiotropic Regulatory Locus 1 (PRL1) (Németh et al., 1998; Li et al., 2019). SKIP plays an essential regulatory role in the circadian clock and salt stress responses in Arabidopsis by controlling alternative pre-mRNA splicing (Wang et al., 2012; Feng et al., 2015; Li et al., 2019). Further, SKIP interacts with a transcriptional activation complex, Polymerase-Associated Factor 1 complex (Paf1c), which mediates flowering through the activation of FLOWERING LOCUS C (FLC) transcription (Cao et al., 2015; Li et al., 2019). Interestingly, other spliceosome components, including MAC3, STIPL1, and PRL1, are not required for the regulation of FLC expression and flowering, and Paf1c is not required for the regulation of salt responses (Li et al., 2019). Therefore, SKIP integrates into two distinct complexes to mediate the floral transition and stress responses at the transcriptional and posttranscriptional levels of gene expression, respectively, in Arabidopsis (Li et al., 2019). However, Paf1c is a mediator of histone monoubiquitination and methylation during transcription in eukaryotes from humans to plants (Zhu et al., 2005; Cao et al., 2015), and SKIP is a transcriptional coregulator of SKIP-mediated transcription. Thus, SKIP requires a partner to achieve target selection during SKIP-mediated gene expression. However, no specific transcription factor or other class of DNA-binding protein that interacts with SKIP to regulate the expression of target genes in plants has been identified.
In this study, we uncovered a direct interaction between SKIP and STM in planta, and we verified that both SKIP and STM bind to the promoters of genes required for SAM formation and that this binding is required for the transcription of those genes and SAM formation. Our findings not only indicate an additional function for SKIP, they also reveal both a transcription factor that works with SKIP and a STM-interacting protein that regulates SAM development in Arabidopsis.
Results
The mutation of SKIP produces a defect in SAM formation
Previously, we isolated a mutant, skip-1, which carries a 22-nucleotide deletion in SKIP that results in a frameshift, which is predicted to generate a truncated protein (Wang et al., 2012). The skip-1 mutant exhibits early flowering with a long-period clock phenotype (Wang et al., 2012; Cao et al., 2015). However, when we characterized a SKIP T-DNA insertion mutant, skip-2, it exhibited severe dwarfism, infertility, and strong developmental defects that prevented it from completing its life cycle (Figure 1, A–C and Supplemental Figure S1, A–C). The transformation of SKIP genomic DNA (with a GFP tag) into skip-2 plants fully complemented all of the developmental defects observed in skip-2, from the seedling stage to the adult stage (Figure 1, A–E), suggesting that the defects observed in skip-2 were due to the mutation of SKIP. Further analysis revealed that skip-1 plants expressed a truncated version of SKIP while no SKIP protein was detected in skip-2 (Figure 1F); thus, skip-1 represents a weak allele, while skip-2 is a strong allele.
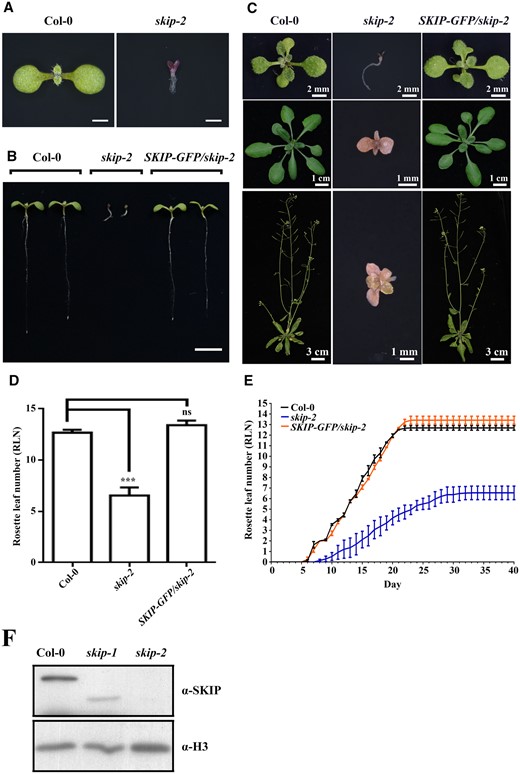
SKIP is an essential part of the Arabidopsis life cycle. A, Seven-day-old wild-type and skip-2 plants. Scale bar = 1 mm. B, Seven-day-old wild-type, skip-2, and skip-2 complemented (pSKIP::SKIP-GFP/skip-2) plants. At least 10 independent transgenic lines with similar phenotypes were observed; one representative line is shown. Scale bar: 1 cm. C, Wild-type, skip-2, and skip-2 complemented (pSKIP::SKIP-GFP/skip-2) plants at 14 days old (top), 21 days old (middle), and 35 days old (bottom). D, The rosette leaf number in wild-type, skip-2, and skip-2 complemented (pSKIP::SKIP-GFP/skip-2) plants. The data represent the mean ± sd from three biological replicates. ***P < 0.001 (Student’s t test). ns: no significant difference. E, Leaf emergence in wild-type, skip-2, and skip-2 complemented (pSKIP::SKIP-GFP/skip-2) plants. The data represent the mean ± sd from three biological replicates. F, Western blot analysis of SKIP protein expression from wild-type and skip alleles using anti-SKIP antibodies.
Given that skip-2 plants exhibited severe developmental defects (Figure 1, A–E) and that postembryonic development in plants is directed by the SAM, we studied SAM development in wild-type and skip-2 plants. Wild-type plants produced a normal SAM and generated leaves, shoots, and floral organs during their life cycle, but no clear SAM was observed in skip-2, and no normal leaves or shoots were generated (Figure 2A). At the cellular level, the SAM in wild-type plants exhibited a clear cellular organization, with L1, L2, and L3 cells, while the enlarged cells in the SAM of skip-2 plants were not organized and no clear L1, L2, or L3 cells were observed (Figure 2, B and C). Notably, the transformation of SKIP genomic DNA into skip-2 plants fully complemented all of the SAM defects observed in the original mutant (Figure 2, A–C). Further analysis suggested that SAM size is decreased in both skip-1 and skip-2 compared with that of wild-type plants in terms of width, height and area, skip-2 exhibited stronger phenotype than skip-1, and this decrease in SAM size was rescued in the complementation line (Figure 2, D–F). Those result indicated that skip-1 plants exhibited a mild defect in the SAM compared with wild-type and skip-2 plants (Figure 2, A–F), consistent with their semi-dwarf phenotype (Supplemental Figure S2). Together, these results suggest that SKIP is required for SAM formation and maintenance, and that the mutation of SKIP causes a defect in SAM formation.

The mutation of SKIP confers SAM defects in Arabidopsis. In contrast to wild-type and skip-2 complemented (pSKIP::SKIP-GFP/skip-2) plants, skip-1 and skip-2 exhibited defects in SAM development. A, Dissecting microscope images of the shoot apices of 7-day-old plants. Scale bar: 400 μm. B, Microscopic images of the SAM in 7-day-old plants stained with toluidine blue. Scale bar: 20 μm. C, Laser scanning confocal microscope observations of the SAM following mPS-PI staining. Scale bar: 40 μm. D, Differential interference contrast microscopy images of the SAM. Scale bar: 20 μm. White dashed lines in (B)–(D) mark the cell layers; arrows indicate the SAM. Numbers indicate the number of plants that exhibited a similar pattern out of the total number of plants observed. E, SAM width and height of the SAM measured shown in (C). The data represent the mean ± sd from 30 SAM. **P < 0.01. ***P < 0.001 (Student’s t test). F, SAM area measured shown in (D). The data represent the mean ± sd from 20 SAM. *P < 0.05. ***P < 0.001 (Student’s t test).
The truncated SKIP protein in skip-1 is partly functional
The skip-1 mutants expressed a truncated SKIP protein and exhibited a weaker SAM phenotype than did skip-2 plants (Figures 1F and 2, A–F); thus, the truncated SKIP protein was partly functional in terms of SAM formation. SKIP contains three domains: N-terminal, SNW, and C-terminal domains (Li et al., 2016). To determine the domain requirement for the function of SKIP in SAM formation in Arabidopsis, we expressed different domains of SKIP in skip-2 plants driven by the native SKIP promoter. Transformation of the SNW (SKIPS), C-terminal (SKIPC), N-terminal + SNW (SKIPNS), or SNW + C-terminal (SKIPSC), but not the N-terminal (SKIPN), domain rescued the phenotypes of the skip-2 mutant at least partly in terms of SAM formation and development (Figure 3, A and B), root length (Figure 3C), fresh weight (Figure 3D), rosette leaf number (Figure 3E), and leaf emergence rate (Figure 3F) compared with the transformation of full-length SKIP, which completely rescued the defects observed in skip-2. Among these truncated proteins, which contained various SKIP domains, SKIPNS and SKIPSC were more effective at rescuing the skip-2 mutant phenotypes compared with SKIPS, SKIPS was more effective than SKIPC, and SKIPN was not functional in Arabidopsis (Figure 3, A–F). These results demonstrate that the truncated SKIP proteins were at least partially functional in skip-2 in terms of SAM formation and maintenance, suggesting that SKIP functions in a domain-friendly and flexible manner in Arabidopsis.
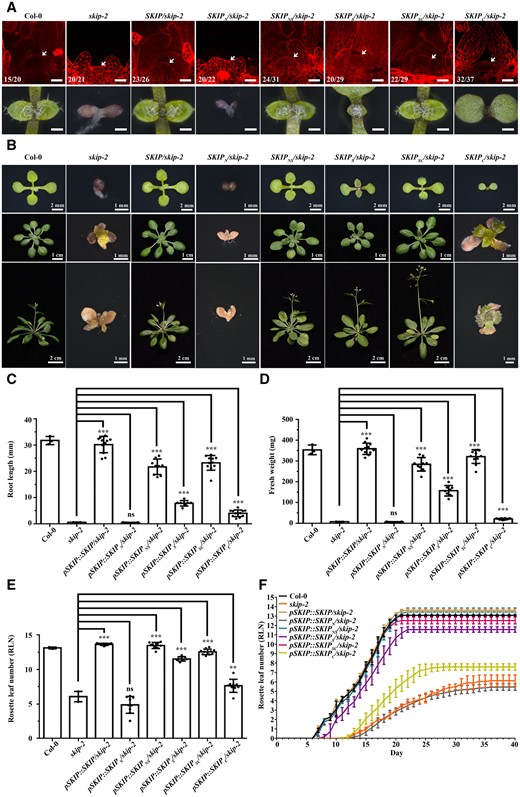
Truncated SKIP proteins were partially functional in Arabidopsis. A, Laser scanning confocal microscope observations of the SAM following mPS-PI staining (top). Scale bar: 40 μm. Arrows indicate the SAM. Numbers indicate the number of plants that exhibited a similar pattern out of the total number of plants observed. Dissecting microscope images of the shoot apices of 7-day-old plants (bottom). Scale bar: 400 μm. B, The development of wild-type, skip-2, and skip-2 plants transformed with full-length or different truncated versions of SKIP at 10 days old (top), 21 days old (middle), and 28 days old (bottom). At least 10 independent transgenic lines exhibited a similar phenotype; one representative independent line for each is shown. C, The root length in wild-type, skip-2, and skip-2 plants transformed with full-length or different truncated versions of SKIP at 7 days old. D, The fresh weight of wild-type, skip-2, and skip-2 plants transformed with full-length or different truncated versions of SKIP at 14 days old. E, The rosette leaf number in wild-type, skip-2, and skip-2 plants transformed with full-length or different truncated versions of SKIP at 28 days old. F, The emergence of leaves in wild-type, skip-2, and skip-2 plants transformed with full-length or different truncated versions of SKIP at 40 days old. For the wild-type and skip-2 plants in (C)–(F), the data represent the mean ± sd from three biological replicates. For each transgenic SKIP line in (C)–(F), the data represent the mean ± sd from 10 independent lines, and for each independent transgenic line, the data represent the mean from three biological replicates. **P < 0.01. ***P < 0.001 (Student’s t test).
SKIP interacts with STM in Arabidopsis
SKIP works both as a splicing factor and a transcriptional coregulator in plants (Wang et al., 2012; Cao et al., 2015). To understand how SKIP regulates SAM formation, we performed a yeast two-hybrid screen with an Arabidopsis yeast two-hybrid library to identify SKIP-interacting proteins. Among the more than 10 million clones examined, STM, a homeodomain transcription factor that is required for SAM formation in Arabidopsis (Long et al., 1996), was identified as a SKIP-interacting protein. This observation was confirmed in a separate yeast two-hybrid assay using SKIP and STM (Figure 4A). Each of the three domains of SKIP (N-terminal, SNW, and C-terminal domains) was capable of interacting with full-length STM, although the strength of the interaction was weaker than that using full-length SKIP or the N-terminal + SNW (SKIPNS) or SNW + C-terminal (SKIPSC) domains of SKIP, and the interaction between the SKIP C-terminal domain (SKIPC) and STM was the weakest one among them (Supplemental Figure S3, A and B). The interaction between full-length or truncated SKIP (except SKIPN) and STM is consistent with the function of full-length or truncated SKIP in rescuing the defects observed in skip-2 plants (Figure 3, A–F). As for SKIPN, although it interacted with STM in yeast (Supplemental Figure S3, A and B), it did not localize to the nucleus and was observed instead in the cytosol (Li et al., 2016). Since SKIP functions in the nucleus, it is not surprising that SKIPN was not functional in Arabidopsis.
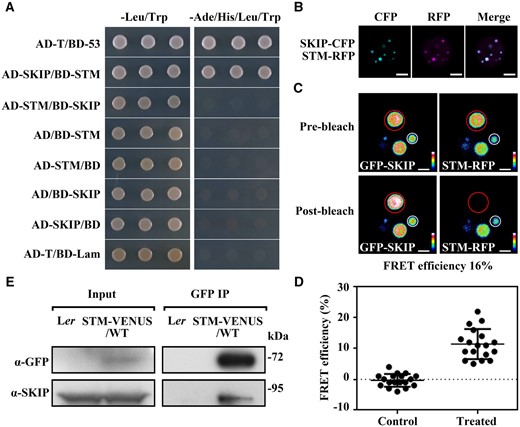
SKIP interacts physically with STM in planta. A, Yeast two-hybrid assay. AD-T/BD-53 is the positive control; AD-T/BD-Lam is the negative control. The interaction between SKIP and STM was assessed by growth on medium lacking leucine and tryptophan (–Leu/Trp, as a growth control) or lacking adenine, histidine, leucine, and tryptophan (–Ade/His/Leu/Trp, as a growth test). B, Colocalization assay in N. benthamiana cells. Laser scanning confocal microscope images from the CFP, RFP, and both (Merge) channels are shown. Scale bar: 5 μm. C, FRET assay in N. benthamiana cells. Laser scanning confocal microscope images from the GFP and RFP channels before and after SKIP-RFP photobleaching are shown. Different colors indicate the difference in fluorescence intensity in the barcode. Efficiency of FRET = (post ID-pre ID)/post ID, where ID is the intensity of the donor (GFP). Scale bar: 2 μm. D, The FRET efficiency from individual cells of three independent biological replicates. The control data were calculated from the nuclear speckle marked with a white circle in (C). The treated data were calculated from the nuclear speckle marked with a red circle in (C). The data represent the mean ± sd from 18 cells. E, Co-IP assay of the interaction between SKIP and STM in Arabidopsis. Total protein from inflorescences containing only unopened flowers in a wild-type (Ler) or pSTM::STM-VENUS (Ler background) background was immunoprecipitated with GFP-trap magnetic beads. The immunoprecipitate was analyzed by Western blotting using anti-GFP or anti-SKIP antibodies as indicated on the left.
Further analysis indicated that SKIP and STM were colocalized in nuclear speckles (Figure 4B), and a strong fluorescence resonance energy transfer (FRET) emission signal was detected in the nuclear speckles of cells coexpressing GFP-SKIP and STM-RFP; this observation was further confirmed in a photobleaching assay using Nicotiana benthamiana cells (Figure 4, C and D). Although it was not as clear as in N. benthamiana cells, STM-VENUS localized in and co-localized with SKIP-CERULEAN in the nuclear speckles of SAM cells in Arabidopsis (Supplemental Figure S4). Finally, the interaction between SKIP and STM in Arabidopsis was confirmed in a co-immunoprecipitation (Co-IP) assay (Figure 4E). These results suggest that SKIP and STM function in the same complex to control SAM formation in Arabidopsis.
Consistent with the interaction between SKIP and STM, the mutation of SKIP and STM produced similar phenotypes in Arabidopsis. Both skip-2 and stm-1 plants exhibited severe defects in cell organization in the SAM, a lack of SAM formation at the shoot tip, failed production of normal leaves and shoots, and an inability to complete their life cycle (Figure 5, A and B). Thus, both SKIP and STM are required for SAM formation in Arabidopsis.
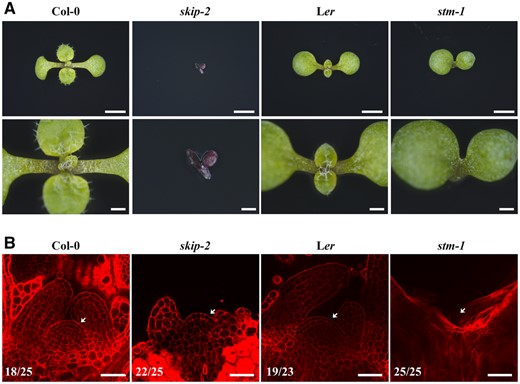
Both skip-2 and stm-1 exhibited defects in SAM development. A, Dissecting microscope images of the shoot apices of 9-day-old wild-type (Col-0), skip-2 (Col-0), wild-type (Ler), and stm-1 (Ler) plants from the top view. Scale bars: 2 mm (first) and 500 μm (second). B, Laser scanning confocal microscope observations of the SAM following mPS-PI staining. Scale bar: 40 μm. Arrows indicate the SAM. Numbers indicate the number of plants that exhibited a similar pattern out of the total number of plants observed.
SKIP expression in the SAM is required for SAM formation and maintenance
SKIP is expressed constitutively during the plant life cycle (Wang et al., 2012). To assess the function of SKIP in the SAM, we specifically expressed SKIP in the SAM region by expressing GFP-SKIP driven by the native STM promoter (pSTM::GFP-SKIP) in skip-2 plants. We first tested the specific expression of the native STM promoter in Arabidopsis (Wang et al., 2012; Landrein et al., 2015). Both pSTM::STM-VENUS and pSTM::GFP-SKIP were specifically expressed in the SAM region in embryos, while pSKIP::SKIP-GFP was expressed in whole embryos (Supplemental Figure S5), indicating that both promoters functioned as expected. We subsequently found that SKIP expression completely rescued the defects in cell organization and SAM formation observed in the skip-2 mutant (Figure 6, A–D). In addition, SKIP expression in the SAM region in skip-2 completely complemented SAM-mediated postembryonic development, including leaf, shoot, and floral organ production (Supplemental Figure S6, A–C). These results suggest that the expression of SKIP in the SAM region is required for SAM formation and development in Arabidopsis.
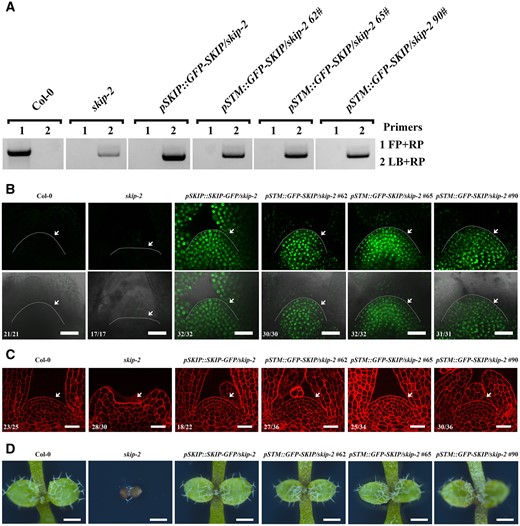
SKIP expression in the SAM region complemented the defect in SAM formation in skip-2. A, Genotyping of complemented skip-2 transgenic plants by the transformation of pSTM::GFP-SKIP into skip-2. FP is forward primer; RP is reverse primer; LB is primer in T-DNA. B, Laser scanning confocal microscope images of the SAM in 9-day-old plants using the GFP channel (top) and “Merge” (bright-field + GFP) channel (bottom). Scale bar: 30 μm. Arrowheads indicate the SAM. C, Laser scanning confocal microscope observations of the SAM in 7-day-old plants following mPS-PI staining. Scale bar: 30 μm. Arrowheads indicate the SAM. The numbers in (B) and (C) indicate the number of plants that exhibited a similar pattern out of the total number of plants observed. D, Dissecting microscope images of the shoot apices of 7-day-old plants. Scale bar: 500 μm.
To confirm this observation, we specifically knocked out SKIP in the SAM region by expressing Cas9-SKIP-sgRNA driven by the native STM promoter (Landrein et al., 2015) (pSTM::Cas9-SKIP-sgRNA) in a skip-2 complementation line (pSKIP::SKIP-GFP/skip-2) (Figure 7A). We subsequently found that Cas9-SKIP-sgRNA expression in the SAM region did not confer SAM formation in our transgenic plants (Figure 7B). DNA sequence analysis indicated that SKIP had been edited to produce two new forms as the result of a frameshift that was predicted to generate very short truncated proteins (Figure 7C and Supplemental Figure S7, A–C). Among 349 individual, independent T1 transgenic lines, 155 exhibited SAM defects as indicated in Figure 7B. Among 65 independent T1 transgenic lines with a normal SAM, none of them possessed an edited form of SKIP, while among 124 independent T1 transgenic lines that exhibited a defect in the SAM, 102 exhibited SKIP editing as indicated (Figure 7C andSupplemental Figure S7, A–C). Further analysis revealed that SKIP-GFP was expressed in the SAM region with the same regular cellular organization observed in our pSKIP::SKIP-GFP/skip-2 line; in contrast, no SKIP-GFP signal was observed in the SAM region of the pSTM::Cas9-SKIP-sgRNA/pSKIP::SKIP-GFP/skip-2 transgenic lines, and the cellular organization in the SAM of our transgenic pSTM::Cas9-SKIP-sgRNA lines was irregular, similar to skip-2 and stm-1 (Figures 5, A and B and 7D). In addition, similar to stm-1, transgenic pSTM::Cas9-SKIP-sgRNA lines produced a few adventitious leaves from the apex at later developmental stages (Supplemental Figure S8, A and B). These findings suggest that the expression of SKIP in the SAM region is necessary for SAM formation in Arabidopsis.

The knockout of SKIP in the SAM using pSTM::Cas9-SKIP-sgRNA caused a defect in SAM formation in Arabidopsis. A, Schematic diagram of the CRISPR/Cas9 binary vectors. The Cas9 cassette was driven by the EC1.1 (top) or STM (bottom) promoter, while target-gRNA was driven by the U6-26 promoter. B, Phenotype of T1 transgenic pSTM::Cas9-SKIP-sgRNA pSKIP::SKIP-GFP/skip-2 plants. Seven-day-old wild-type, pSKIP::SKIP-GFP/skip-2, pSTM::Cas9-SKIP-sgRNA pSKIP::SKIP-GFP/skip-2, and skip-2 plants are shown. Scale bar: 2 mm. C, Genotype analysis of SKIP genomic DNA from T1 plants using the primer pair SKIPg1/2-F/R. The arrowhead indicates the edited SKIP genomic DNA, which lacked sequences between Target 1 and Target 2. D, In contrast to wild-type and pSKIP::SKIP-GFP/skip-2 plants, the pSTM::Cas9-SKIP-sgRNA pSKIP::SKIP-GFP/skip-2 plants exhibited a defect in SAM (arrowheads) formation. Laser scanning confocal microscope images of the SAM in 9-day-old plants using the GFP channel (top). Scale bar: 60 μm. Laser scanning confocal microscope observations of the SAM following mPS-PI staining (middle). Scale bar: 40 μm. Dissecting microscope images of the shoot apices of 7-day-old plants (bottom). Scale bar: 500 μm. Numbers indicate the number of plants that exhibited a similar pattern out of the total number of plants observed.
The SKIP-STM complex works as a transcriptional activator to regulate the expression of target genes
STM localizes to both the nucleus and cytosol (Hay and Tsiantis, 2010). The translocation of STM from the nucleus to the cytosol, which is mediated by STM-interacting proteins, is one point of regulation in the function of STM (Hay and Tsiantis, 2010). In comparison, SKIP contains two nuclear localization sequences (NLSs) in its SNW and C-terminal domains, respectively, and it is a nuclear protein in Arabidopsis (Wang et al., 2012; Li et al., 2016). Therefore, SKIP may function as an STM-interacting protein to recruit STM to the nucleus, and the defects in SAM formation observed in skip-2 may be due to the exclusion of STM from the nucleus caused by a loss of SKIP function. If this were the case, nuclear expression of STM would recue the SAM defects observed in skip-2. To test this, we fused 2 NLSs to the C-terminus of STM and GFP, in frame, after the NLSs to generate a nonmobile nuclear-localized version of STM (STM-2NLS-GFP) (Balkunde et al., 2017), which was expressed in skip-2 plants under the control of the native STM promoter. Consistent with previous reports, overexpression of STM in wild-type plants produced a lobed leaf phenotype (Supplemental Figure S9A; Cole et al, 2006; Balkunde et al., 2017), indicating that STM-2NLS-GFP was functional in Arabidopsis under our conditions (Supplemental Figure S9A). However, although STM-2NLS-GFP was expressed and localized to the nucleus in skip-2 plants based on the observation of overlapping STM-2NLS-GFP and DAPI signals, the nucleus-specific expression of STM in skip-2 had no effect on SAM formation (Supplemental Figure S9, A and B). These results suggest that the defects in SAM formation in skip-2 were not caused by a defect in the nuclear localization of STM due to the lack of its interacting partner, SKIP. Our results also suggest that both STM and SKIP are required for SAM formation in Arabidopsis.
STM works as a transcription factor to activate or repress the expression of specific target genes in plants (Sakamoto et al., 2001; Tsuda et al., 2014), while SKIP functions as a transcriptional coregulator of gene expression in Arabidopsis (Cao et al., 2015; Li et al., 2019). Therefore, the STM-SKIP complex may function as a transcriptional complex to regulate the expression of target genes in the SAM. STM (Tsuda et al., 2011), KNAT1 (Tsuda et al., 2011), CLV3 (Su et al., 2020), and GA 2-oxidase 1 (GA2OX1) (Bolduc and Hake, 2009) are typical STM target genes. Therefore, the STM-SKIP complex may bind to chromatin where these genes reside and regulate their expression. Chromatin immunoprecipitation (ChIP) revealed that both STM and SKIP could bind directly to the promoter regions of STM, KNAT1, CLAVATA 3 (CLV3), and GA2OX1 (Figure 8A), and reverse transcription-quantitative real time polymerase chain reaction (RT-qPCR) analysis confirmed that the mutation of SKIP or STM decreased the expression of STM, KNAT1, CLV3, and GA2OX1 in Arabidopsis (Figure 8B andSupplemental Figure S10). Thus, STM and SKIP regulate a similar set of target genes that are required for SAM formation. This supports the notion that a SKIP-STM transcriptional complex is required to regulate the expression of genes that support SAM formation in Arabidopsis.
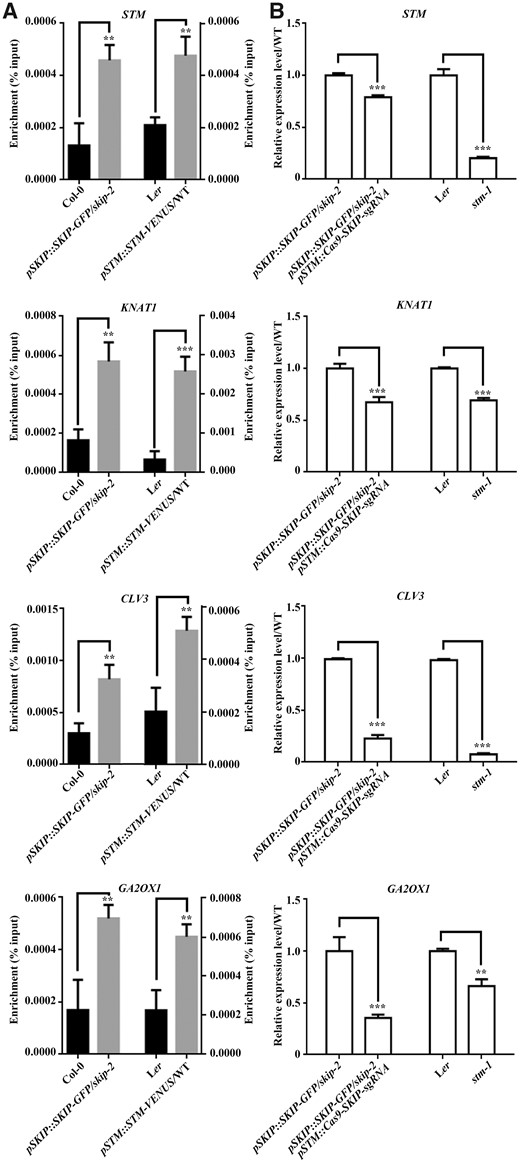
SKIP and STM bind to the promoter of target genes to regulate their expression in Arabidopsis. A, ChIP-qPCR of STM (first intron region), KNAT1 (promoter region), CLV3 (promoter region), and GA2OX1 (promoter region). ChIP products were obtained by immunoprecipitation using samples from inflorescences containing only unopened flowers of wild-type (Col-0) and pSKIP::SKIP-GFP/skip-2 (for SKIP binding) or wild-type (Ler) and pSTM::STM-VENUS (for STM binding) plants with anti-GFP antibodies. B, Relative expression levels of STM, KNAT1, CLV3, and GA2OX1 as determined by RT-qPCR. The samples are from pSKIP::SKIP-GFP/skip-2 and pSTM::Cas9-SKIP-sgRNA pSKIP::SKIP-GFP/skip-2 (for SKIP-regulated expression) or Ler and stm-1 (for STM-regulated expression) plants. The primers used are shown in Supplemental Table S1. The data represent the mean ± sd from three biological replicates. **P < 0.01, ***P < 0.001 (Student’s t test).
Paf1c is required for SAM formation
Our previous results indicated that SKIP interacted with Paf1c, and SKIP-Paf1c-mediated FLC transcription is required for the regulation of floral transition in Arabidopsis (Cao et al., 2015; Li et al., 2019). In this work, it was observed that SKIP interacted with STM to regulate SAM development. Therefore, it is interesting to know whether Paf1c is required for SAM development. It was observed that EARLY FLOWERING 7 (elf7) exhibits similar SAM defects to skip-1 and stm-bum1 (Figure 9, A and B). FRET assay verified that STM interacts with ELF7 in N. benthamiana cells (Figure 9, C and D), and mutation in ELF7 or ELF8 down-regulated the expression of KNAT1 and GA2OX1 (Figure 9E). Those results are consistent with the observation of the interaction between SKIP and Paf1c, and indicated that Paf1c is required for SAM formation.
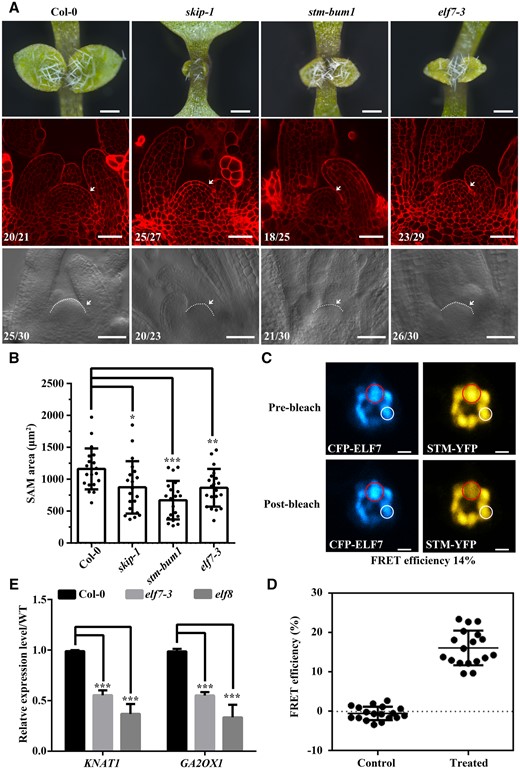
The mutation in ELF7 confers SAM defects in Arabidopsis. elf7-3 exhibited similar SAM defects in Arabidopsis. A, Dissecting microscope images of the shoot apices of 7-day-old plants (top, scale bar: 400 μm). Laser scanning confocal microscope observations of the SAM following mPS-PI staining (middle, scale bar: 40 μm). Differential interference contrast microscopy images of the SAM (bottom, scale bar: 40 μm). Numbers indicate the number of plants that exhibited a similar pattern out of the total number of plants observed. White dashed lines and arrows indicate the SAM. B, SAM area was measured from bottom of (A). The data represent the mean ± sd from 20 SAM. *P < 0.05, **P < 0.01, ***P < 0.001 (Student’s t test). C, FRET assay in N. benthamiana cells. Laser scanning confocal microscope images from the CFP and YFP channels before and after STM-YFP photobleaching are shown. Efficiency of FRET = (post ID-pre ID)/post ID, where ID is the intensity of the donor (CFP). Scale bar: 2 μm. D, The FRET efficiency from individual cells of three independent biological replicates. The control data were calculated from the nuclear speckle marked with a white circle in (C). The treated data were calculated from the nuclear speckle marked with a red circle in (C). The data represent the mean ± sd from 18 cells. E, Relative expression levels of KNAT1 and GA2OX1 as determined by RT-qPCR. The samples are from Col-0, elf7, and elf8 plants. The primers used are shown in Supplemental Table S1. The data represent the mean ± sd from three biological replicates. ***P < 0.001 (Student’s t test).
Discussion
STM is a transcription factor that works with SKIP to regulate the expression of genes required for SAM formation and maintenance
SKIP is required for the expression of several genes, including STM, KNAT1, CLV3, GA2OX1 (Figure 8, A and B), and FLC (Cao et al., 2015; Li et al., 2019). It also interacts with such transcriptional machinery as Paf1c (Cao et al., 2015; Li et al., 2019). However, neither SKIP nor Paf1c has a DNA-binding domain, so they are not capable of binding directly to target genes. Therefore, SKIP requires partner proteins for target selection. Transcription factors target specific genes by binding directly to those genes using their DNA-binding domain. Thus, transcription factors are good candidate partners for SKIP. We found that both SKIP and STM are required for SAM formation and maintenance (Figures 1–3 and 5–8), that SKIP interacts physically with STM in planta (Figure 4 andSupplemental Figure S3), and that SKIP binds to the promoter of STM target genes (Figure 8A). Thus, the transcription factor STM mediates target gene selection in SKIP-mediated SAM formation and maintenance by interacting with SKIP to regulate target gene expression. Therefore, SKIP may regulate distinct developmental processes by interacting with different transcription factors. As SKIP is also a splicing factor required for alternative gene splicing, it would be interesting to know whether SKIP couples the transcription and alternative splicing of its target genes and their transcripts.
SKIP is a cofactor of STM that regulates the expression of genes required for SAM formation and maintenance
STM is a transcription factor that regulates the expression of genes required for SAM formation and maintenance. To achieve this function, STM must work with cofactors to form a transcriptional complex (Hay and Tsiantis, 2010). BELL family proteins are cofactors of STM; STM and BELL proteins form heterodimers to regulate target genes (Cole et al., 2006), and different combinations of KNOX/BELL transcription factors may regulate different downstream genes. Within a STM–BELL heterodimer, STM is responsible for transcriptional activation while BELL is responsible for DNA binding (Cao et al., 2020). Another STM cofactor is mini-KNOX, a KNOX protein that lacks a homeodomain. Mini-KNOX interacts selectively with BELL proteins and affects their availability to form active STM-BELL complexes; therefore, mini-KNOX antagonizes the regulatory activity of STM-BELL complexes (Kimura et al., 2008; Magnani and Hake, 2008). These results indicate that KNOX proteins regulate target genes differently depending on their cofactor.
In this study, we found that SKIP interacts with STM in a domain-friendly and flexible manner (Figure 4 andSupplemental Figure S3), and that both of them bind to the promoter of their target genes and are required for the expression of these genes (Figure 8, A and B). This suggests that SKIP is a cofactor of STM that helps promote its gene regulatory function. We previously confirmed that SKIP interacts with Paf1c to regulate the transcription of FLC (Cao et al, 2015; Li et al., 2019). Paf1c is a transcriptional mediator that is required for the initiation and elongation phases of transcription (Zhu et al., 2005; Cao et al., 2015). Interestingly, VERNALIZATION INDEPENDENCE 3 (VIP3), which encodes a component of Paf1c, interacts genetically with STM (Takagi and Ueguchi, 2012), and the mutation of VIP3 confers a defect in PZ development, reducing the size of the SAM to half that in wild-type, and it produces a semi-dwarf phenotype in Arabidopsis (Fal et al., 2017). In this work, we also observed that mutation in ELF7, another component of Paf1c, confers similar SAM defects as that of STM and SKIP (Figure 9, A and B), and confirmed the physical interaction between STM and ELF7 (Figure 9, C and D). Taken together, these results suggest that SKIP functions as a cofactor of STM to recruit transcriptional machinery (e.g. Paf1c) to STM target genes and promote their transcription (i.e. initiation and elongation; Figure 10). However, as SKIP also works as a splicing factor and is required for the alternative splicing of pre-mRNAs in Arabidopsis (Wang et al., 2012; Feng et al., 2015; Li et al., 2019), mutation in SKIP has a slight defect in the splicing of STM, KNAT1, CLV1, and CLV3 (but not obvious compared with that of a tolerance gene PROTEIN S-ACYL TRANSFERASE 10 [PAT10]) (Supplemental Figure S11). Therefore, we cannot rule out the possibility that SKIP-mediated alternative splicing also contributes to the regulation of SAM development.
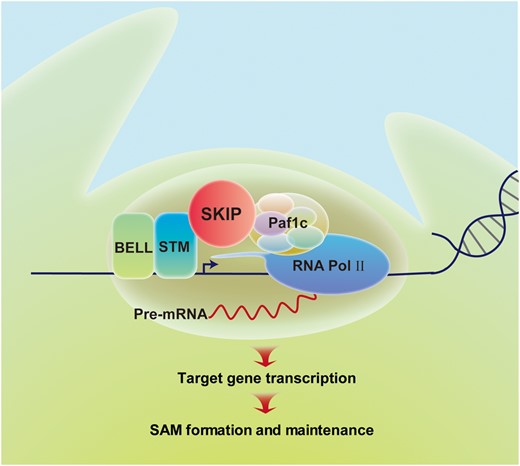
Proposed model for SKIP-STM-regulated gene expression and SAM formation in Arabidopsis. SKIP works as a cofactor with STM to recruit transcriptional machinery (e.g. Paf1c) to STM target genes. This promotes the initiation and elongation phases of transcription from these target genes, leading to formation and maintenance of the SAM in Arabidopsis.
SKIP interacts with STM and possibly other KNOX family members required for the formation of axillary and floral meristems
There are four members of the KNOXI family in Arabidopsis (Hay and Tsiantis, 2010), and they exhibit identical yet distinct functions. For example, KNAT6 (but not KNAT2) acts redundantly with STM to regulate SAM activity and organ separation, while KNAT1 functions antagonistically with KNAT6 and KNAT2 in inflorescence development (Belles-Boix et al., 2006; Ragni et al., 2008). In addition, KNOX proteins are required for the formation of axillary and floral meristems (Roth et al., 2018; Cao et al., 2020). Considering that SKIP is a flexible protein (Supplemental Figure S3), it would be interesting to know whether SKIP interacts with KNOX family proteins other than STM, and whether SKIP is required for the formation of axillary and floral meristems to mediate branching and floral development in Arabidopsis.
Both SKIP and KNOX proteins are conserved among plants and possibly even among eukaryotes, and KNOX proteins function in diverse developmental contexts (Kimura et al., 2008; Hay and Tsiantis, 2010; Kierzkowski et al., 2019). It will thus be interesting to determine whether our model for SKIP-KNOX function in Arabidopsis applies to diverse species and in distinct developmental contexts.
Materials and methods
Plant materials and growth conditions
All Arabidopsis (A.thaliana) plant materials used in this study were of the Columbia-0 (Col-0) or Landsberg erecta (Ler) ecotype. The following mutants were described previously: skip-1 (Col-0) (Wang et al., 2012), skip-2 (Col-0) (Li et al., 2016), stm-1 (Ler) (Long et al., 1996), and STM-VENUS/WT (Ler) (Heisler et al., 2005; Shi et al., 2016), stm-bum1 (Col-0) (Shi et al., 2016), and elf7-3 (Col-0) (Cao et al., 2015). All mutations were confirmed by PCR and sequencing. The primers used to characterize the mutants by PCR are given in Supplemental Table S1. Seedlings were grown in a Percival CU36L5 growth chamber (Percival Scientific, Perry, IA, USA) under 16 h of white light at an intensity of 70 mmol m−2 s−1 at 22°C, followed by 8 h of darkness at 18°C. Adult plants were cultivated in a greenhouse and grown at 22°C for 16 h in the light at a white light intensity of 150 mmol m−2 s−1 and at 18°C for 8 h in the dark.
Construction of transgenic plants
For pSKIP::SKIP construction, the pSKIP::SKIP genomic region (1,800-bp promoter region and whole coding region) was amplified from Col-0 genomic DNA then cloned into Pst I and Sac I sites of the binary vector pCAMBIA1300 containing the NOS terminator. For pSTM::GFP-SKIP construction, the SKIP genomic region fused to GFP driven by the native STM promoter (Landrein et al., 2015) was cloned into Pst I and Sac I sites of the binary vector pCAMBIA1300 containing the NOS terminator. For pSTM::Cas9-SKIP-sgRNA construction, the SKIP-sgRNA cassette was obtained by PCR amplification from pCBC-DT1T2 using the primer pair DT1-F0/BsF and DT2-R0/BsR. The PCR products were digested with Bsa I and the digested fragment was inserted into pHEE401 (Wang et al., 2015), resulting in the production of Cas9-SKIP-sgRNA. The STM promoter was inserted into the Spe I and Xba I sites of pHEE401, resulting in the production of pSTM::Cas9-SKIP-sgRNA. For pSTM::STM-2NLS-GFP construction, the STM genomic region fused to the 2NLS-GFP tag driven by its native promoter was cloned into Pst I and Sac I sites of the binary vector pCAMBIA1300 containing the NOS terminator. For pSKIP::SKIP-CERULEAN construction, the SKIP genomic region fused to the CERULEAN tag driven by its native promoter was cloned into the Pst I and Sac I sites of binary vector pCAMBIA1300 containing the NOS terminator. For pSKIP::SKIP-GFP construction, the SKIP genomic region fused to the GFP tag driven by its native promoter was cloned into the Pst I and Sac I sites of binary vector pCAMBIA1300 containing the NOS terminator. The primers and restriction sites used to construct for expression vector are given in Supplemental Table S1.
Yeast two-hybrid assays
The coding regions of SKIP and various SKIP deletion derivatives were cloned into pGADT7, while the coding region of STM was cloned into pGBKT7. Different combinations of vectors were cotransformed into Saccharomyces cerevisiae strain AH109. The primers and restriction sites used to clone the coding sequences of SKIP and STM are included in Supplemental Table S1. Transformation, yeast growth, and protein extraction were performed as described in the Clontech Yeast Protocols Handbook.
FRET assays
FRET assays were conducted as described previously (Li et al., 2016). The coding regions of SKIP and STM were fused to GFP and RFP, or the coding regions of ELF7 and STM were fused to CFP and YFP, respectively, and then cloned into the binary vector pCAMBIA1300. The primers used to clone the coding sequences of SKIP and STM are included in Supplemental Table S1. The two constructs were transiently expressed in N.benthamiana leaves infiltrated with Agrobacterium tumefaciens strains carrying the appropriate binary plasmids.
Two days after transformation, a FRET assay was performed with a Zeiss 780 laser scanning confocal microscope (Jena, Germany). Prebleached images of the donor (GFP/CFP) and acceptor (RFP/YFP) were acquired using the GFP/CFP channel and RFP/YFP channel. Next, the fluorescence intensity in a region of interest in the nucleus was recorded using the software provided by the manufacturer (Zeiss, Jena, Germany). STM-RFP/STM-YFP was then photobleached by repeated scanning of the selected region with a 561-/514-nm laser beam. Postbleached images of GFP-SKIP/CFP-ELF7 and STM-RFP/STM-YFP were acquired after the photobleaching of RFP/YFP, respectively. After correcting for changes in image registration, the fluorescence intensities of the two GFP images were calculated. The energy transfer efficiency between the two interacting proteins was calculated as follows: Efficiency of FRET = (post ID-pre ID)/post ID, where ID is the intensity of the donor (GFP/CFP). The experimental setup used for the confocal work is given in Supplemental Table S2.
Co-IP
Inflorescences containing only unopened flowers in a Ler or pSTM::STM-Venus background were dissected for immunoprecipitation. Total protein was extracted with immunoprecipitation buffer (50 mM Tris–HCl, pH 8.0, 150 mM NaCl, 0.3% [v/v] NP40, 10% [v/v] glycerol, 1 mM PMSF, and protease inhibitor] and immunoprecipitated with GFP-Trap magnetic beads. The immunoprecipitates were separated by sodium dodecyl sulfate-polyacrylamide gel electrophoresis and analyzed by Western blotting with anti-GFP or anti-SKIP antibodies.
Histology and microscopy
Each specimen was prepared as described previously (Lee et al., 2010). To prepare semi-thin sections, shoot tips were fixed in a solution containing 2.5% (v/v) glutaraldehyde in 0.1 M PBS buffer (pH 7.2) under vacuum conditions for 30 min. After fixing for 2 days at 4°C, the shoot tips were rinsed twice with phosphate buffer and then dehydrated at room temperature in a graded series of ethanol starting at 30% then going to 40%, 50%, 60%, 70%, 80%, 90%, 95%, and 100% (v/v). The specimens were embedded in Spurr’s low-viscosity embedding medium. Infiltration was started in a 2:1 ethanol/embedding medium. The specimens were left in the mixture with swirling of the container for 2 h after which the medium was replaced with a 1:1 ethanol/embedding medium and left in the container, which was swirled, for 4 h. Next, the medium was replaced with 1:3 ethanol/embedding medium and incubated with swirling of the container for another 6 h. After that, the mixture was drained and embedding medium was added to the container and left overnight. After curing the specimens for 48 h at 65°C, the specimens were mounted and sectioned at a thickness of 1 μm using a UC6 microtome (Leica, Wetzlar, Germany). After staining briefly with 0.1% toluidine blue, the sections were observed under bright-field optics using an Axio Imager M2 microscope (Zeiss).
Modified pseudo-Schiff propidium iodide staining
Each specimen was prepared as described previously (Truernit et al., 2008) with some modifications. For modified pseudo-Schiff propidium iodide (mPS-PI) staining, shoot tips were fixed in 50% (v/v) methanol and 10% (v/v) acetic acid overnight at 4°C then washed with water and dehydrated in an ethanol series (30%, 50%, 70%, 80%, 90%, 95%, and 100% [v/v]). The shoot tips were partially rehydrated through a graded ethanol series from 100% to 15% (v/v). The shoot tips were then rinsed with water and then treated with 1% (w/v) periodic acid for 40 min at room temperature. The shoot tips were then rinsed with water again and incubated in Schiff reagent (0.2 g of NaHSO3, 200 μg of PI, 62.5 μL of HCl, and 9.8 mL of ddH2O) for 2 h. The shoot tips were next covered with chloral hydrate solution (8 g of chloral hydrate, 2 mL of glycerol, and 4 mL of ddH2O) for 10 min and mounted in Hoyer’s solution (30 g of gum arabic, 200 g of chloral hydrate, 20 g of glycerol, and 50 mL of water) on slides for observation. The size of each cleared shoot meristem was measured using the software provided by Zeiss for image analysis.
Image acquisition
An Axio Zoom V16 dissecting microscope (Zeiss) was used to photograph living seedlings. The mPS-PI-stained samples, CERULEAN signal, and VENUS signal in vivo were observed with a Zeiss 780 laser scanning confocal microscope. The DAPI-stained samples and GFP signal in vivo were imaged with a Leica SP8 X confocal microscope (Wetzlar, Germany). The experimental setup used for the confocal work is given in Supplemental Table S2.
Tissues clearing and SAM observation
Each specimen was prepared as described previously (Su et al., 2020) with some modifications. Shoot tips were fixed in 50% (v/v) methanol and 10% (v/v) acetic acid 3 h at room temperature. Then washed with water and dehydrated in an ethanol series (100%, 95%, 85%, and 70% [v/v]). Shoot tips were mounted in chloral hydrate solution (8 g of chloral hydrate, 2 mL of glycerol, and 4 mL of ddH2O) for 3 h. Next, the cleared tissues with SAM were examined by differential interference contrast microscopy using an Axio Imager M2 microscope (Zeiss). The size of each cleared shoot meristem was measured using software provided by Zeiss for image analysis.
Collect samples at T1 generation from CRISPR lines
Enough T0 seeds were generated and more than 200 individual T1 seedlings were collected for the first replicate of DNA sequencing and RT-qPCR experiments, and >50 individual T1 seedlings for other two biological replicates of DNA sequencing and RT-qPCR experiments.
RNA extraction and RT-qPCR
Total RNA was isolated from 9-day-old wild-type or mutant seedlings using Trizol reagent (Invitrogen, Carlsbad, CA, USA) and then treated with RNase-free DNase I (Promega, Madison, WI, USA) to degrade remaining DNA. Next, 3 μg of total RNA was used for cDNA synthesis with a RevertAid First Strand cDNA Synthesis Kit with oligo(dT) primer (Thermo Fisher Scientific, Waltham, MA, USA). RT-qPCR was performed using the cDNA as a template; the primers are shown in Supplemental Table S1. Takara SYBR Premix Ex Taq (Takara Bio, Otsu, Japan) and a 7500 Real-Time PCR instrument (Applied Biosystems, Foster City, CA, USA) were used for RT-qPCR.
ChIP assays
ChIP was performed as described previously (Cheng et al., 2013) with some modifications. Inflorescences containing only unopened flowers from pSKIP::SKIP-GFP/skip-2 and pSTM::STM-Venus/WT (Ler) plants were dissected and ground in liquid nitrogen. The powder was fixed in PBS buffer containing 1% (v/v) formaldehyde for 10 min at 4°C and the unreacted formaldehyde was quenched with glycine for 10 min. Following the isolation of nuclei and immunoprecipitation with anti-GFP antibodies, the DNA products were eluted with elution buffer. Quantitative PCR was performed using the bound DNA fragments as templates. The DNA samples underwent 45 cycles of amplification using the primers shown in Supplemental Table S1.
Statistical analysis
The statistical analysis in this study was conducted by two-sided unpaired t test.
Accession numbers
Sequence data from this article can be found in the GenBank/EMBL data libraries under the following accession numbers: SKIP, NM_001036214.3; STM, NM_104916.4; ELF7, NM_106622.5; KNAT1, NM_116884.4; CLV3, NM_128283.2; GA2OX1, NM_106491.2; WUS, NM_127349.4; PAT10, NM_114998.5.
Supplemental data
The following materials are available in the online version of this article.
Supplemental Figure S1. Characterization of the skip-2 mutant.
Supplemental Figure S2. skip-1 has a semi-dwarf phenotype.
Supplemental Figure S3. The interaction between STM and full-length or truncated SKIP as determined in yeast two-hybrid assays.
Supplemental Figure S4. The subcellular localization of STM and co-localization of SKIP and STM in Arabidopsis.
Supplemental Figure S5. STM promoter-driven expression of SKIP and STM in the SAM during early embryogenesis.
Supplemental Figure S6. SKIP expression in the SAM region complemented the life cycle defects observed in skip-2.
Supplemental Figure S7. pSTM::Cas9-SKIP-sgRNA-edited SKIP in Arabidopsis.
Supplemental Figure S8. The knockout of SKIP in the SAM using pSTM::Cas9-SKIP-sgRNA caused a defect in SAM at later developmental stages in Arabidopsis.
Supplemental Figure S9. The transformation of nuclear-localized STM in skip-2 had no effect on SAM formation in skip-2 plants.
Supplemental Figure S10. Mutation in SKIP down-regulated the expression of genes required for SAM development.
Supplemental Figure S11. The effect of SKIP mutation on the splicing of genes required for SAM development and salt tolerance.
Supplemental Table S1. The primers used in this study.
Supplemental Table S2. The experimental setup used for the confocal work in this study.
Acknowledgments
We thank Dr. Jessica Habashi for critical reading of the manuscript. We also thank Dr. Xianyong Sheng of the Imaging Center, College of life Sciences, Capital Normal University (Beijing, China), for performing the FRET and localization of proteins assays.
Funding
This work was supported by grants from the National Natural Science Foundation of China (31972857, 32000454).
Conflict of interest statement. None declared.
R.L., Y.C., L.D., and L.M. designed the concept. R.L., Z.W., Y.L., and X.S. did the experimental work. Y.C. and L.D. supervised parts of this project. R.L. and L.M. wrote the manuscript with input from all the authors.
The author responsible for distribution of materials integral to the findings presented in this article in accordance with the policy described in the Instructions for Authors (https://dbpia.nl.go.kr/plphys/pages/general-instructions) is: Ligeng Ma ([email protected]).
References
Author notes
Senior author