-
PDF
- Split View
-
Views
-
Cite
Cite
Yutao Wang, Ning Wang, Jingqiu Lan, Yige Pan, Yidan Jiang, Yongqi Wu, Xuemei Chen, Xianzhong Feng, Genji Qin, Arabidopsis transcription factor TCP4 controls the identity of the apical gynoecium, The Plant Cell, Volume 36, Issue 7, July 2024, Pages 2668–2688, https://doi.org/10.1093/plcell/koae107
- Share Icon Share
Abstract
The style and stigma at the apical gynoecium are crucial for flowering plant reproduction. However, the mechanisms underlying specification of the apical gynoecium remain unclear. Here, we demonstrate that Class II TEOSINTE BRANCHED 1/CYCLOIDEA/PCF (TCP) transcription factors are critical for apical gynoecium specification in Arabidopsis (Arabidopsis thaliana). The septuple tcp2 tcp3 tcp4 tcp5 tcp10 tcp13 tcp17 (tcpSEP) and duodecuple tcp2 tcp3 tcp4 tcp5 tcp10 tcp13 tcp17 tcp24 tcp1 tcp12 tcp18 tcp16 (tcpDUO) mutants produce narrower and longer styles, while disruption of TCPs and CRABS CLAW (CRC) or NGATHAs (NGAs) in tcpDUO crc or tcpDUO nga1 nga2 nga4 causes the apical gynoecium to be replaced by lamellar structures with indeterminate growth. TCPs are predominantly expressed in the apex of the gynoecium. TCP4 interacts with CRC to synergistically upregulate the expression level of NGAs, and NGAs further form high-order complexes to control the expression of auxin-related genes in the apical gynoecium by directly interacting with TCP4. Our findings demonstrate that TCP4 physically associates with CRC and NGAs to control auxin biosynthesis in forming fine structures of the apical gynoecium.
Background: Gynoecium is the female reproductive organ with elegant structures that protect ovules and facilitate pollination in angiosperms. The apical gynoecium contains the stigmatic papillae, style and transmitting tract for pollen tube growth and the successful fertilization.
Question: How is the elegant apical gynoecium specified in angiosperms?
Findings: This study demonstrates that TCP transcription factors synergistically interacted with CRC transcription factor and the NGA transcription factors to finely regulate auxin biosynthesis and specify the elegant structure of apical gynoecium. The disruption of CRC and twelve TCP transcription factors in the tredecuple mutant tcpDUO crc leads to the change of stigma and style into indeterminate lamellar structures. TCP4 interacts with CRC to synergistically promote the expression of NGA genes. NGAs further interact with TCP4-CRC to facilitate auxin biosynthesis in the apical gynoecium. Correspondingly, the compromise of three NGAs and twelve TCPs in the quindecuple tcpDUO nga1 nga2 nga4 mutants recapitulates the phenotype of tcpDUO crc in apical gynoecium.
Next steps: The identity of the indeterminate lamellar structures turned from stigma and style tissues could be further identified, and the integration mechanisms of multiple transcription factors to tightly control the fate of apical gynoecium could be further studied.
Introduction
The gynoecium or pistil is the female reproductive organ in angiosperms and is vital for sexual reproduction. The gynoecium evolves elegant structures to protect ovules and facilitate pollination, and finally develops into the fruit to continually protect seeds and facilitate seed dispersal (Reyes-Olalde et al. 2023). In Arabidopsis (Arabidopsis thaliana), gynoecium development begins with the initiation of the gynoecium primordium at the center of the floral meristem (Zuniga-Mayo et al. 2019). First, a simple tube-like cylindrical structure consisting of two congenitally fused carpels is produced at the center of flower. Then, in the inner or adaxial side of the cylinder structure, two carpel margin meristems are formed at the two opposed fusion sites of the two carpels and develop into two ridge-like structures (Ferrándiz et al. 1999; Reyes-Olalde and de Folter 2019). More complex structures are consecutively differentiated as the two ridges are fused together to form the septum. Most importantly, four rows of placental tissues and ovule primordia are formed at the two flanks of the septum, while the stigmatic papillae, style and transmitting tract are differentiated at the apical site in the mature gynoecium (Reyes-Olalde et al. 2013).
Multiple transcription factors tightly control the formation of fine gynoecium structures in a coordinated manner. According to the ABCDE and floral quartet models, MADS-box protein AGAMOUS (AG) is a key transcription factor that forms quartet complexes with other MADS-box homologs, including SEPALLATA (SEP) proteins, SEEDSTICK (STK), SHATTERPROOF1 (SHP1) and SHP2, to specify gynoecium structures (Theißen and Saedler 2001; Theißen et al. 2016). AG and SEPs form AG–SEP quartets to determine carpel identity, while AG, SEPs, STK, and SHP1/SHP2 form AG–SEP–STK/SHP quartets to specify ovule identity (Favaro et al. 2003). No gynoecium structures are formed in the flower of the ag single mutant, sep1 sep2 sep3 triple mutant, or sep1 sep2 sep3 sep4 quadruple mutant (Yanofsky et al. 1990; Ditta et al. 2004). STK is specifically expressed in ovules and very important for ovule development (Favaro et al. 2003). The ovules are converted into carpelloid structures in the gynoecium of the stk shp1 shp2 triple mutant due to the absence of AG–SEP–STK/SHP quartets (Pinyopich et al. 2003; Theißen et al. 2016). The AG–SEP3 quartet regulates CRABS CLAW (CRC), which encodes a YABBY family transcription factor, by directly binding to its promoter (Gómez-Mena et al. 2005; Ó’Maoiléidigh et al. 2013; Hugouvieux et al. 2018). CRC is critical for the development of the apical gynoecium (Alvarez and Smyth 1999; Bowman and Smyth 1999). Although the disruption of either CRC in the crc single mutant or SPATULA (SPT) encoding a basic helix-loop-helix (bHLH) transcription factor in the spt single mutant leads only to weakly unfused carpels in the apical gynoecium, the crc spt double mutant had carpels that were nearly completely unfused, as well as severely reduced formation of apical gynoecium structures, including the stigmatic papillae, style, and transmitting tract (Alvarez and Smyth 1999; Bowman and Smyth 1999; Groszmann et al. 2010). In addition to SPT, other bHLH family transcription factors, including HECATEs (HECs) and INDEHISCENT (IND), also participate in regulating apical gynoecium development (Liljegren et al. 2004; Gremski et al. 2007). The triple mutant hec1 hec2 hec3 does not form stigmatic papillae or a transmitting tract, while IND acts redundantly with SPT to control the development of the apical gynoecium, as demonstrated by the report indicating that the ind spt double mutant also lacks stigmatic papillae and a transmitting tract (Girin et al. 2011). It has been proposed that transcription factors are coordinately and sequentially activated to form transcriptional complexes to regulate apical gynoecium development. HEC proteins first interact with NGATHA (NGA) transcription factors to promote IND expression (Ballester et al. 2021). IND then forms a complex with NGA and HEC to activate SPT expression (Ballester et al. 2021). SPT further interacts with IND, NGA, and HEC to form high-order transcriptional complexes to control apical gynoecium development by regulating the expression of auxin-related genes (Ballester et al. 2021). NGA transcription factors consist of a small RAV subgroup of the B3-domain transcription factor family that plays redundant and pivotal roles in the control of the apical gynoecium (Alvarez et al. 2009; Trigueros et al. 2009). The single nga mutant displays weakly defective style development, while all apical gynoecium structures, including the stigma and style, are absent in the triple nga1 nga3 nga4, nga2 nga3 nga4 and quadruple nga1 nga2 nga3 nga4 mutants (Alvarez et al. 2009; Trigueros et al. 2009). NGAs cooperatively function with zinc-finger transcription factors belonging to the small SHORT INTERNODES/STYLISH (SHI/STY) family to specify the apical gynoecium by regulating auxin biosynthesis (Alvarez et al. 2009). Recently, the STIGMA AND STYLE STYLIST 1 (SSS1), SSS2, and SSS3 genes have been identified as direct targets of NGA3 (Li et al. 2020). Overexpression of each SSS gene causes short styles, while the triple sss1 sss2 sss3 mutant produced longer styles that affected pollen tube growth (Li et al. 2020). Some other transcription factors or regulators, including ETTIN/AUXIN RESPONSE FACTOR3 (ETT/ARF3), REPLUMLESS (RPL), BREVIPEDICELLUS/ARABIDOPSIS THALIANA HOMEOBOX GENE1 (BP/KNAT1), AINTEGUMENTA (ANT), REVOLUTA (REV), SEUSS (SEU) and LEUNIG (LUG), also play important roles during gynoecium development (Sessions et al. 1997; Liu et al. 2000; Otsuga et al. 2001; Venglat et al. 2002; Azhakanandam et al. 2008; Zhao et al. 2015; Simonini et al. 2016). These indicate that the specification and development of fine gynoecium structures are crucial for the success of sexual reproduction, and plants evolve a complex and elegant transcriptional regulation network to tightly regulate apical gynoecium. Indeed, more than 250 interactions have been identified between 82 transcription factors related to gynoecium development by a recent high-throughput yeast two-hybrid (Y2H) assay study (Herrera-Ubaldo et al. 2023). However, the molecular mechanisms underlying the specification of the apical gynoecium remain to be clarified.
Members of the plant-specific TEOSINTE BRANCHED 1/CYCLOIDEA/PCF (TCP) transcription factor family are classified into Class I and Class II subfamilies according to the protein sequence of the conserved TCP domain (Martin-Trillo and Cubas 2010). Class II TCPs are further divided into CINCINNATA (CIN)-like and CYC/TB1-like TCP subgroups. The Arabidopsis CIN-like TCP subgroup contains TCP2, TCP3, TCP4, TCP5, TCP10, TCP13, TCP17, and TCP24, while the CYC/TB1-like TCP subgroup consists of TCP1, TCP12, and TCP18 (Lan and Qin 2020). Among CIN-like TCPs, TCP2, TCP3, TCP4, TCP10, and TCP24 are post-transcriptionally regulated by microRNA319 (miRNA319), while TCP5, TCP13, and TCP17 are not (Koyama et al. 2017). Class II TCPs play critical roles in diverse aspects of plant development, such as leaf development (Koyama et al. 2007, 2010; Tao et al. 2013), flower development (Zheng et al. 2022), shoot branching, and trichome development (Aguilar-Martinez et al. 2007; Yang et al. 2018). However, the roles of Class II TCPs in the specification of the apical gynoecium are not well defined.
In this study, we generated high-order multiple tcp mutants and demonstrated that Class II TCP transcription factors play highly redundant roles in controlling the fine structures of the apical gynoecium. Disruption of CIN-like TCPs in the septuple tcp2 tcp3 tcp4 tcp5 tcp10 tcp13 tcp17 (tcpSEP) mutant caused narrower and longer styles in the apical gynoecium. The pyramiding of mutants disrupting CYC/TB1-like TCP genes in tcpSEP to obtain a duodecuple tcp2 tcp3 tcp4 tcp5 tcp10 tcp13 tcp17 tcp24 tcp1 tcp12 tcp18 tcp16 (tcpDUO) mutant showed that tcpDUO produced pistils with even longer styles. Interestingly, the tredecuple tcpDUO crc mutant produced previously unidentified lamellar structures instead of normal stigmatic papillae, styles and transmitting tissues. We further showed that TCPs play synergetic and redundant roles in specifying the apical gynoecium with CRC and NGA transcription factors.
Results
Class II TCP transcription factors negatively regulate style length in the apical gynoecium
We previously generated a tcp2 tcp3 tcp4 tcp5 tcp10 tcp13 tcp17 septuple (tcpSEP) mutant and showed that CIN-like TCPs play important and redundant roles in repressing trichome formation and petal greening (Lan et al. 2021; Zheng et al. 2022). We observed that the tcpSEP mutant also displayed longer styles in apical gynoecia. Compared to the styles of wild-type plants, those of tcpSEP were longer and narrower (Fig. 1, A, B, D, E, and I). We further pyramided tcp12 (SALK_023116), tcp16 (GABI_655C10), and tcp18 (SALK_091920), as well as CRISPR/Cas9-derived mutants tcp1 and tcp24, with tcpSEP to obtain a tcp2 tcp3 tcp4 tcp5 tcp10 tcp13 tcp17 tcp24 tcp1 tcp12 tcp18 tcp16 duodecuple (tcpDUO) mutant by genetic crosses (Supplementary Fig. S1; Lan et al. 2023), because Class I TCP16 contains a TCP domain that has more similarities with that of Class II TCP (Viola et al. 2012). The styles of tcpDUO were even longer than those of tcpSEP (Fig. 1, A to F and I). Scanning electron microscopy (SEM) analysis showed that the cell number along the basal–apical axis was obviously increased in the style of tcpSEP and tcpDUO in comparison with that of the wild-type control (Fig. 1, D to F). Statistical analysis showed that the cell number in the style was higher along the longitudinal direction, but lower along the transverse direction in tcpDUO than in wild-type control (Fig. 1, G and H). However, the cell size in the style had no significant differences between tcpDUO and wild type (Supplementary Fig. S2, A and B). We further observed the internal structures of the apical gynoecium using a semithin section. The results showed that the apical gynoecium of tcpDUO contained a transmitting tract. However, it also became longer and narrower accordingly when compared to that of wild type (Supplementary Fig. S3, A and B). To further determine the redundant function of TCPs in apical gynoecium, we observed the apical gynoecium of the tcp4 single mutant, tcp4 tcp10 double mutant, tcp3 tcp4 tcp5 tcp10 tcp13 quintuple mutant and tcp2 tcp3 tcp4 tcp5 tcp10 tcp13 sextuple mutant. The results showed that the apical gynoecium of tcp4 and tcp4 tcp10 had no obvious differences from that of the wild-type control (Supplementary Fig. S4). However, tcp3 tcp4 tcp5 tcp10 tcp13 and tcp2 tcp3 tcp4 tcp5 tcp10 tcp13 produced significantly longer styles (Supplementary Fig. S4). Accordingly, the styles of siliques from wild-type control were clearly shorter than those from tcpSEP, and tcpDUO produced the longest styles in siliques (Supplementary Fig. S5). We then generated TCP4pro-TCP4-GFP and TCP10pro-TCP10-GFP in which TCP4 or TCP10 fused with GFP was driven by the TCP4 or TCP10 promoter. We transformed TCP4pro-TCP4-GFP or TCP10pro-TCP10-GFP into tcpSEP. The results showed that TCP4pro-TCP4-GFP but not TCP10pro-TCP10-GFP completely complemented the longer style of tcpSEP (Fig. 1, I and J to L). These results indicate that TCP4 plays a more important role than TCP10 in regulating the fine structures of apical gynoecia.
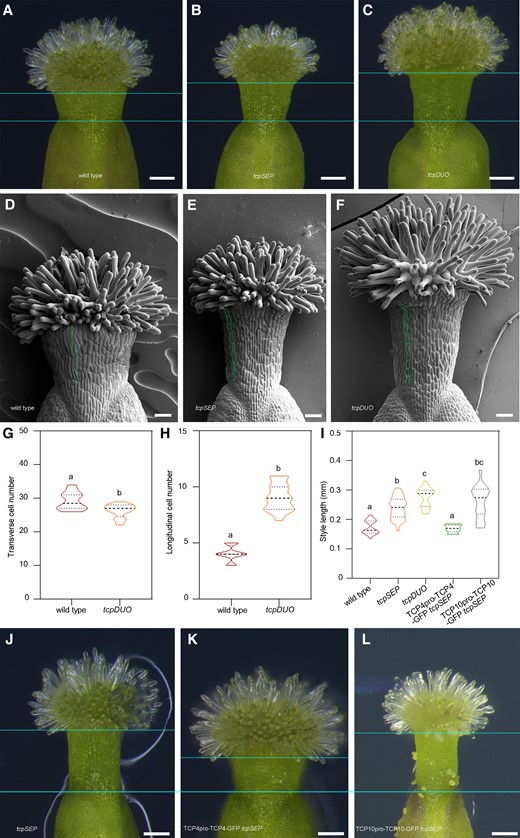
Disruption of Class II TCP transcription factors in septuple tcpSEP or duodecuple tcpDUO caused narrower and longer styles in the apical gynoecium. A to C) Styles from wild type (A), tcpSEP (B), and tcpDUO (C). The flowers of tcpSEP, tcpDUO, and wild-type control were emasculated at flower developmental stage 11. The apical gynoecium was then isolated and observed at 24 h after emasculation. Compared to the wild-type styles, those of tcpSEP and tcpDUO were narrower and longer. Bars = 100 μm. D to F) SEM observation of the styles from wild type (D), tcpSEP (E), and tcpDUO (F). In comparison with the wild type, the cell number along the basal–apical axis was obviously increased in the styles of tcpSEP and tcpDUO. Bars = 50 μm. G) Statistical analysis of the transverse cell number of wild type and tcpDUO. The closed circles represent quartiles, and the short black lines represents the medians in the violin plot. Statistical significance was determined by one-way ANOVA (n = 16). Significant differences at P < 0.05 are indicated by different lowercase letters (Supplementary Data Set 8). H) Statistical analysis of the longitudinal cell number of wild type and tcpDUO. The closed circles represent quartiles, and the short black lines represents the medians in the violin plot. Statistical significance was determined by one-way ANOVA (n = 16). Significant differences at P < 0.05 are indicated by different lowercase letters (Supplementary Data Set 8). I) Statistical analysis of the style lengths of wild type, tcpSEP, tcpDUO, TCP4pro-TCP4-GFP tcpSEP, and TCP10pro-TCP10-GFP tcpSEP. The closed circles represent quartiles, and the short black lines represents the medians in the violin plot. Statistical significance was determined by one-way ANOVA (n ≥ 10). Significant differences at P < 0.05 are indicated by different lowercase letters (Supplementary Data Set 8). J to L) Styles from tcpSEP (J), TCP4pro-TCP4-GFP tcpSEP (K), and TCP10pro-TCP10-GFP tcpSEP (L). The narrower and longer styles of tcpSEP were complemented by transformation of TCP4pro-TCP4-GFP. Bars = 100 μm.
Class II TCP transcription factors participate in specifying the identity of the apical gynoecium
CRC transcription factors act downstream of AG–SEP complexes and play key roles in controlling apical gynoecium development (Bowman and Smyth et al. 1999). To further reveal the important functions of Class II TCP transcription factors in the formation of the apical gynoecium, we used CRISPR/Cas9 technology to create CRC mutations in the tcpDUO background and successfully obtained the tredecuple mutant tcpDUO crc (Supplementary Fig. S6). Observations of tcpDUO crc showed that tcpDUO had a strongly synergistic interaction with crc in the establishment of the apical gynoecium. The apical gynoecium of tcpDUO displayed a longer style and normal stigma (Fig. 2, A and B), while crc produced gynoecia with unfused carpels at the apex and reduced tissues of styles and stigmas (Fig. 2C). However, tcpDUO crc did not form any styles or stigmas (Fig. 2D). Instead, the apical gynoecium of tcpDUO crc produced unknown tissues with indeterminate growth activity. SEM revealed that many lamellar structures grew on the apical gynoecium (Fig. 2, E to G). On the margins of the lamellar structure, many protuberances were initiated and continued to grow in an indeterminate manner (Fig. 2, H to J). In addition, a similar silique phenotype was produced (Fig. 2, K and L), and the abnormal growth of the apical gynoecium caused severe abortion in tcpDUO crc. The striking phenotype of the apical gynoecia of tcpDUO crc indicates that TCP transcription factors play synergetic roles in regulating the identity and determinate growth of the apical gynoecium with CRC transcription factor.
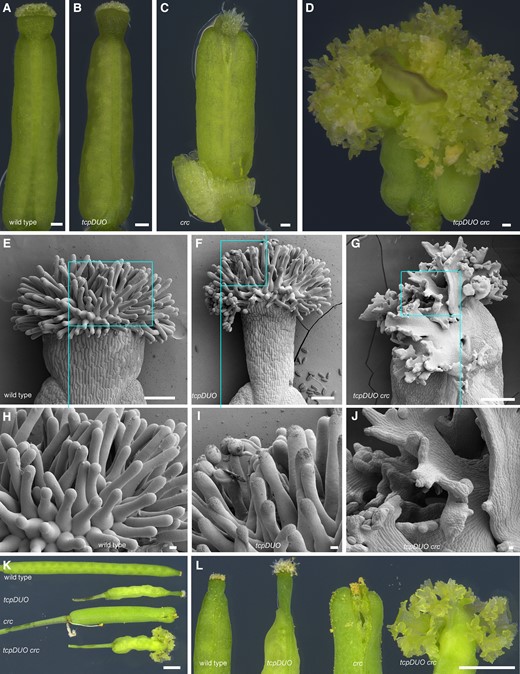
TCP transcription factors play redundant and crucial roles in regulating the identity of the apical gynoecium with CRC. A to D) Gynoecium from wild type (A), tcpDUO(B), crc(C), and tcpDUO crc(D). The flowers of wild type, tcpDUO, crc, and tcpDUO crc were emasculated at flower developmental stage 11. The apical gynoecium was then isolated and observed at 24 h after emasculation. Bars = 100 μm. E to J) SEM analysis of the apical gynoecium from wild type (E, H), tcpDUO (F, I), and tcpDUO crc (G, J). H to J) Close-up view of the apical gynoecium from (E to G). Many lamellar structures with indeterminate protuberances grew on the apical gynoecium of tcpDUO crc. Scale bars, 100 μm in (E to G), 10 μm in (H to J). K, L) Siliques and close-up views showing the apical gynoecium from wild type, tcpDUO, crc, and tcpDUO crc. Bars = 1 mm.
TCP4, TCP5, and TCP12 are predominantly expressed in the apical gynoecium
To provide more evidence showing that TCP transcription factors participate in apical gynoecia development, we first investigated the expression patterns of TCP4, TCP5, and TCP12, representing three subclades of the Class II TCP phylogenetic tree, respectively. We cloned the 2095-bp TCP12 promoter and used it to drive a β-glucuronidase (GUS) reporter gene in the TCP12pro-GUS construct. We obtained transgenic lines by transforming TCP12pro-GUS into wild-type plants. GUS staining showed that fifteen TCP12pro-GUS transgenic lines displayed similar expression patterns, and we selected a stable representative line for detailed analysis. The stable TCP4pro-TCP4-GUS and TCP5pro-GUS transgenic lines were generated as reported previously (Han et al. 2019; Zheng et al. 2022). The results showed that TCP4 protein was expressed in pistils at nearly all flower developmental stages (Fig. 3A). At stage 9, TCP4 protein was weakly detected at the lateral valves of the tube-like gynoecium (Fig. 3B). TCP4 expression increased as flower development progressed (Fig. 3, B to D). Clear GUS staining was found in the stigmas, styles, and valves at flower stage 11 (Fig. 3C). TCP4 was consistently expressed in the apical gynoecium and whole valves in the mature flowers at stage 13 (Fig. 3D). TCP5 and TCP12 genes had similar expression patterns, as shown by the GUS staining results for flowers from the TCP5pro-GUS and TCP12pro-GUS transgenic lines. TCP5 and TCP12 were found to be expressed in the gynoecia of young and old flowers (Fig. 3, E to J), and they were predominantly expressed in the stigma and style of the apical gynoecium.
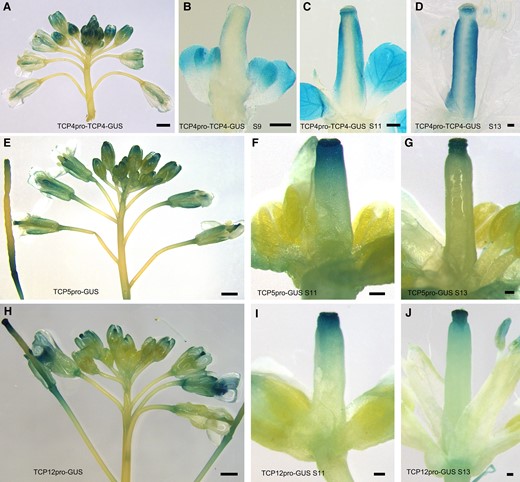
The expression patterns of TCP4, TCP5, and TCP12 at different flower developmental stages. A) GUS staining of TCP4pro-TCP4-GUS showed that TCP4 was expressed in pistils at nearly all flower developmental stages. Bars = 100 μm. B to D) TCP4 protein was detected at stage 9 (B), stage 11 (C), and stage 13 (D). Bars = 100 μm. E, H) GUS staining of inflorescences from TCP5pro-GUS (E) and the TCP12pro-GUS (H) showed that TCP5 and TCP12 were expressed in the gynoecia of young and old flowers. Bars = 100 μm. F, G) The expression patterns of TCP5 at flower developmental stage 11 (F) and stage 13 (G). Bars = 100 μm. I, J) The expression patterns of TCP12 at flower developmental stage 11 (I) and stage 13 (J). TCP5 and TCP12 were predominantly expressed in the stigma and style of the apical gynoecium. Bars = 100 μm.
We further investigated the expression patterns of TCP1, TCP2, TCP3, TCP13, TCP17, and TCP18 in the apical gynoecium using TCP1pro-GUS, TCP2pro-GUS, TCP3pro-GUS, TCP13pro-GUS, TCP17pro-GUS, and TCP18pro-GUS transgenic lines. The results showed that TCP1 was slightly expressed in the apical gynoecium (Supplementary Fig. S7, A to C), while TCP2, TCP3, TCP13, and TCP17 were predominantly expressed in the apical gynoecium (Supplementary Fig. S7, D to O). However, no expression of TCP18 was observed in apical gynoecium (Supplementary Fig. S7, P to R).
CRC and NGAs are key regulators of the apical gynoecium, and CRC and NGAs are expressed in the apical gynoecium (Bowman and Smyth 1999; Alvarez et al. 2009; Trigueros et al. 2009; Martínez-Fernández et al. 2014; Gaillochet et al. 2018). These data indicate that TCPs have overlapping expression patterns with CRC and NGAs, which is consistent with the redundant functions of TCPs in shaping the apical gynoecium.
Transcriptome analysis of the apical gynoecia of crc, tcpDUO, and tcpDUO crc
To reveal the molecular mechanisms by which CRC and TCP transcription factors synergistically regulate the growth and identity of the apical gynoecium, we emasculated flowers collected from crc, tcpDUO, tcpDUO crc, and wild-type control plants to avoid pollen contamination. We dissected and collected apical gynoecia, including style and stigma tissues, for RNA-sequencing (RNA-seq) with three replicates at 24 h after emasculation (DAE). Principal component analysis (PCA) indicated that the results of the three replicates for each sample were consistent (Supplementary Fig. S8).
We then compared the transcriptomes of wild-type apical gynoecia with those from crc (WT vs crc), tcpDUO (WT vs tcpDUO), and tcpDUO crc (WT vs tcpDUO crc). The results showed that 1,915 differentially expressed genes (DEGs, false discovery rate < 0.05; fold-change ≥ 2.0 or ≤ −2.0), including 630 upregulated genes and 1,285 downregulated genes, were identified in WT vs crc (Supplementary Data Sets S1 and S2, Fig. 4A). The comparison of WT and tcpDUO transcriptomes identified 3,422 DEGs (1,786 upregulated genes and 1,636 downregulated genes) (Supplementary Data Sets S3 and S4, Fig. 4A). Finally, the comparison of WT and tcpDUO crc revealed 5,710 DEGs, consisting of 2,496 upregulated genes and 3,214 downregulated genes (Supplementary Data Sets S5 and S6, Fig. 4A). These data suggest that the simultaneous disruption of both TCP and CRC caused more intensive changes in comparison with disruption of either TCPs or CRC in the gynoecium apical transcriptome, consistent with the phenotypic severity exhibited in crc, tcpDUO, and tcpDUO crc.
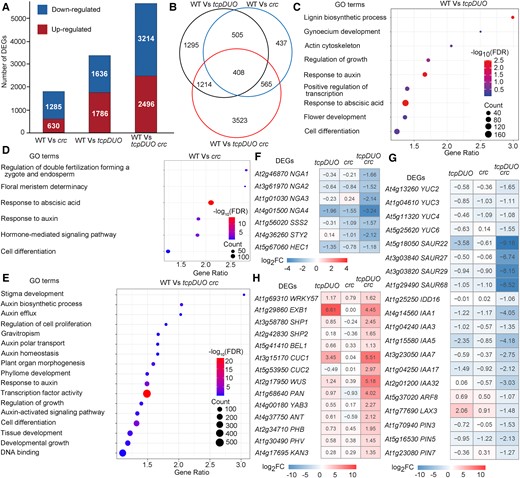
TCPs and CRC synergistically regulate many important transcription factors and auxin-related genes. A) DEGs (fold change ≥ 2.0 or ≤ −2.0 and false discovery rate < 0.05) between the apical gynoecia from wild type, crc, tcpDUO, and tcpDUO crc. B) Overlapping DEGs for the WT vs tcpDUO, WT vs crc, and WT vs tcpDUO crc comparisons. C to E) GO analysis of the DEGs in the WT vs tcpDUO (C), WT vs crc (D), and WT vs tcpDUO crc comparisons (E). The terms stigma development, auxin biosynthetic process, auxin polar transport, and response to auxin and transcription factor activity were significantly enriched in WT vs tcpDUO crc. F, H) Heatmap analysis of DEGs encoding transcription factors related to flower development. G) Heatmap analysis of genes related to auxin biosynthesis, transport and signaling.
Venn diagram analysis showed that nearly half (47.7%) of DEGs in WT vs crc were also identified in WT vs tcpDUO (Fig. 4B), suggesting that CRC and TCPs share many common downstream genes in the control of the apical gynoecia. Interestingly, 3,523 (61.7%) DEGs were specifically found in WT vs tcpDUO crc (Fig. 4B), suggesting that disruption of either TCPs or CRC was insufficient to produce significant changes in the expression of these genes. These findings suggest that TCPs and CRC synergistically regulate more than half of the DEGs identified in WT vs tcpDUO crc. Gene ontology (GO) analysis of the DEGs showed that terms such as gynoecium development, response to auxin, cell differentiation, and regulation of growth were significantly enriched in either WT vs tcpDUO or WT vs crc (Fig. 4, C and D). The significantly enriched terms identified in the WT vs tcpDUO crc GO analysis included stigma development, auxin biosynthetic process, auxin polar transport, and response to auxin and transcription factor activity (Fig. 4E), suggesting that auxin-related pathways and the function of transcription factors were altered in tcpDUO crc.
We then searched for changes in the expression levels of genes encoding transcription factors known to control apical gynoecia in tcpDUO, crc, and tcpDUO crc in comparison with wild-type control. We found that NGA1, NGA2, NGA3, and NGA4 were significantly downregulated in both tcpDUO and crc, and the expression of these genes was even lower in tcpDUO crc (Fig. 4F). The NGA3 downstream gene SSS2 was correspondingly downregulated in the mutants with the greatest decrease in expression in tcpDUO crc (Fig. 4F). NGAs have been reported to directly regulate auxin biosynthesis genes (Trigueros et al. 2009; Fourquin and Ferrandiz 2014; Martínez-Fernández et al. 2014). We next searched for changes in the expression levels of auxin-related genes in the mutants. Consistent with changes in the expression levels of NGA genes, genes related to auxin biosynthesis, transport and signaling, such as YUCCA2 (YUC2), YUC6, PIN-FORMED3 (PIN3), PIN5, PIN7, SMALL AUXIN-UP RNA (SAUR), and AUXIN/INDOLE-3-ACETIC ACID (AUX/IAA) genes, were also downregulated to a greater extent in tcpDUO crc than in tcpDUO or crc (Fig. 4G). To investigate the expression changes of the TCP downstream genes in the gynoecia of flowers at early developmental stage, we further dissected and collected the gynoecium tissues at flower developmental stage 9. The reverse transcription quantitative PCR (RT-qPCR) analysis showed that NGA1, NGA2, NGA3, NGA4, YUC2, and YUC6 were significantly downregulated in tcpDUO at flower development stage 9 (Supplementary Fig. S9). To determine whether TCPs could directly regulate NGAs and YUCs, we first search our chromatin immunoprecipitation assay with sequencing (ChIP-seq) data for the signal of TCP4 binding in the downstream gene loci (Dong et al. 2019). There were clear TCP4 binding signals in the promoters of NGA1, NGA2, NGA3, NGA4, and YUC2 (Supplementary Fig. S10). Second, we performed gel electrophoresis mobility shift assays (EMSA) to confirm that TCP4 could directly bind to the promoters of NGA1 and YUC2 (Supplementary Fig. S11, A and B). Third, the transient expression assay clearly showed that TCP4 activated the promoter of YUC2 in Nicotiana benthamiana leaves (Supplementary Fig. S11, C and D). These data suggest that NGA genes and auxin biosynthesis genes were synergistically upregulated by CRC and TCPs in apical gynoecia. In addition, STY2 and HEC1, which encode proteins that play key roles in apical gynoecium development, were also downregulated in the mutants (Fig. 4F).
We also found that some genes encoding transcription factors were upregulated to a much greater degree in tcpDUO crc than in tcpDUO or crc, including the ovule identity genes SHP1 and SHP2 (Pinyopich et al. 2003), and the meristem activity gene WUS (Yamaguchi et al. 2017) (Fig. 4H). The upregulation of SHP1 and SHP2 was consistent with the ectopic ovules observed in the apical tissues of tcpDUO crc (Fig. 2, G and J). In tcpDUO crc gynoecia, the high expression level of WUS may be responsible for the indeterminate growth of apical tissues associated with high meristematic activity (Fig. 2, G and J). Interestingly, we found that EXB1 and its homolog WRKY57, which encode proteins that we previously showed to be pivotal for shoot branching (Guo et al. 2015), were significantly upregulated in the apical tissues of tcpDUO (Fig. 4H). The expression level of EXB1 was highly increased in tcpDUO, but not altered in crc, indicating that EXB1 expression was specifically regulated by TCPs (Fig. 4H).
These results suggest that TCPs and CRC synergistically regulate many important transcription factors and auxin-related genes to finely control the structures of apical gynoecia, consistent with the synergistical phenotypes of the apical gynoecia of tcpDUO crc.
Disruption of NGA genes in the tcpDUO background recapitulates the phenotype of tcpDUO crc
To determine whether reduced expression of NGA genes could cause the deformed apical gynoecia in tcpDUO crc, we mutated NGA genes in the tcpDUO background using CRISPR/Cas9 technology. We generated a construct carrying sgRNA sequences targeting NGA1, NGA2, NGA3, and NGA4, and the construct was transformed into tcpDUO. After investigating hundreds of lines, we did not find mutations in the NGA3 locus, but we obtained two lines with different mutations in the NGA1, NGA2, and NGA4 loci (Supplementary Fig. S12). Interestingly, the quindecuple tcpDUO nga1 nga2 nga4 mutants displayed a phenotype similar to that observed in the tcpDUO crc mutant (Fig. 5, A to G). The apical gynoecium of the quindecuple mutants did not produce styles or stigmas; indeed, the apical gynoecium was replaced by unknown tissue that consisted of many lamellar structures (Fig. 5, C and D), and SEM analysis showed that a large number of protuberances had grown on the lamellar structures (Fig. 5, F and G). The defects of the apical gynoecium in tcpDUO nga1 nga2 nga4 is similar to that observed in the pistils of 35S:miR319a/35S:amiR-NGA plants (Alvarez et al. 2016), further supporting our above data. These results demonstrate that NGA proteins synergistically specify the identity of apical gynoecia with TCP transcription factors.
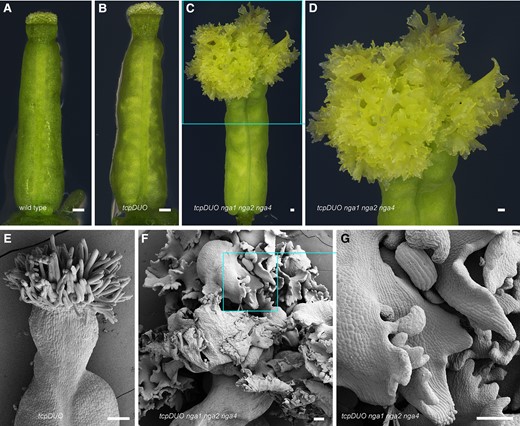
NGA synergistically specifies the identity of the apical gynoecia with TCP transcription factors. A to D) Gynoecium from wild type (A), tcpDUO (B), and tcpDUO nga1 nga2 nga4 (C, D). D) Close-up view of the apical gynoecium from (C). The flowers of wild type, tcpDUO, and tcpDUO nga1 nga2 nga4 were emasculated at flower developmental stage 11. The apical gynoecium was then dissected and observed at 24 h after emasculation. Bars = 100 μm. E to G) SEM analysis of the apical gynoecium from tcpDUO (E) and tcpDUO nga1 nga2 nga4 (F, G). G) Close-up view of the apical gynoecium from (F). The apical gynoecium of tcpDUO nga1 nga2 nga4 displayed an unknown tissue that consisted of many lamellar structures, similar to the tcpDUO crc mutant. Bars = 100 μm.
TCP4 forms a transcriptional complex with CRC and NGA
It has been reported that NGA interacts and cooperates with HEC transcription factors to induce the expression of IND and SPT (Ballester et al. 2021). Subsequently, IND and SPT form larger multimeric complexes with NGA and HEC proteins to control stigma development (Ballester et al. 2021). Since the results described above indicate that NGA genes were cooperatively upregulated by TCPs and CRC, we hypothesized that TCP proteins could interact with CRC transcription factors to form an upstream transcriptional complex controlling the expression of NGA genes. To test this hypothesis, we selected TCP4, TCP5, TCP12, and TCP18 as representatives to investigate their interactions with CRC using Y2H assays. The results showed that CRC had strong interactions with TCP4 and TCP18, as well as relatively weak interactions with TCP5 and TCP12 (Fig. 6A). We next generated the constructs 35S-CRC-nLUC and 35S-cLUC-mTCP4, in which a CRC or miRNA319-resistant mTCP4 fusion with a sequence encoding the N-terminus or C-terminus of LUC was driven by the CaMV 35S promoter. Luciferase complementation imaging (LCI) assays showed clear fluorescence in the N. benthamiana leaves that co-expressed 35S-CRC-nLUC and 35S-cLUC-mTCP4, while no fluorescence was detected in the control combinations (Fig. 6B), suggesting that CRC interacted with TCP4 in planta. We finally generated 35S-CRC-Flag and 35S-GFP-mTCP4, in which a CRC or mTCP4 fusion with a sequence encoding a Flag or GFP tag was driven by the CaMV 35S promoter. We co-transformed 35S-CRC-Flag with 35S-GFP-mTCP4 in N. benthamiana leaves. Co-immunoprecipitation (Co-IP) with anti-GFP beads showed that GFP-mTCP4 pulled down CRC-Flag, confirming that TCP4 interacted with CRC in vivo (Fig. 6C). To test whether the upregulated NGAs formed multimeric complexes with TCP transcription factors, we performed Y2H assays with NGA1, NGA2, NGA3, and NGA4 as bait, and with TCP4 as prey. All four NGA proteins physically interacted with TCP4 in yeast (Fig. 6D). LCI analysis confirmed that TCP4 interacted with NGA proteins in vivo (Fig. 6, E to H). These results suggest that TCP4 interacts with CRC to cooperatively upregulate NGA genes, and NGA proteins subsequently form multimeric complexes to regulate downstream gene expression.
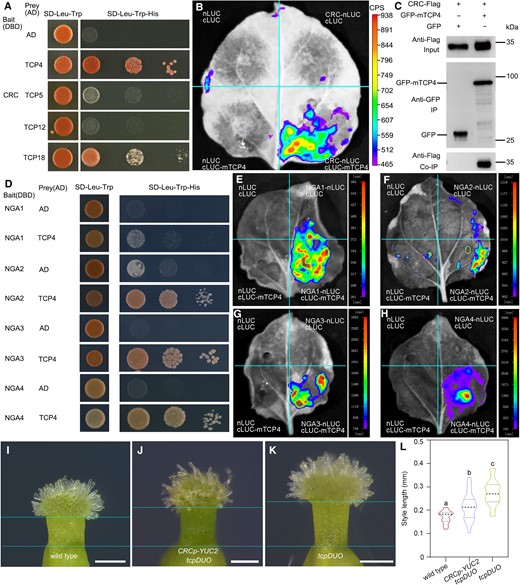
TCP4 forms a transcriptional complex with CRC and NGA. A) TCP4, TCP5, TCP12, and TCP18 interacted with CRC according to the results of Y2H assays. The transformed yeast was spotted on the selection SD-Leu-Trp-His medium with 10-, 100-, and 1,000-fold dilutions. Full-length CRC was used as bait. AD, activation domain; DBD, DNA-binding domain. B) LCI assays showed the interaction between TCP4 and CRC in N. benthamiana leaves. The miR319-resistant TCP4 protein was fused with cLUC, and CRC was fused with nLUC. nLUC and cLUC fused with GAL4BD were used as negative controls. CPS, counts per second. C) Co-IP assays showed the interaction between TCP4 and CRC in vivo. N. benthamiana leaves co-transformed with CRC-Flag and TCP4-GFP or GFP were grown for 2 d. Total proteins were extracted and immunoprecipitated with anti-GFP beads and then detected with an anti-Flag antibody. D) Y2H assays showed that TCP4 was bound to NGA1, NGA2, NGA3, and NGA4. The selection conditions were as described above. E to H) LCI analysis showed that TCP4 interacted with NGA1 (E), NGA2 (F), NGA3 (G), and NGA4 (H). NGA1, NGA2, NGA3, and NGA4 were fused with nLUC. CPS, count per second. I to K) Styles from wild type (I), CRCp-YUC2 tcpDUO (J), and tcpDUO (K). The flowers of wild type, CRCp-YUC2 tcpDUO, and tcpDUO were emasculated at flower developmental stage 11. The apical gynoecium was then dissected and observed at 24 h after emasculation. CRCp-YUC2 tcpDUO can partially complement for the style length phenotype of tcpDUO. Bars = 200 μm. L) Statistical analysis of the style lengths of wild type, CRCp-YUC2 tcpDUO, and tcpDUO. The closed circles represent quartiles, and the short black lines represents the medians in the violin plot. Statistical significance was determined by one-way ANOVA (n ≥ 17). Significant differences at P < 0.05 are indicated by different lowercase letters (Supplementary Data Set 8).
TCP4, CRC, and NGAs control auxin biosynthesis by regulating the transcription of YUC genes (Supplementary Fig. S11, B to D) (Trigueros et al. 2009; Yamaguchi et al. 2018; Zhou et al. 2018). Because both tcpDUO crc and tcpDUO nga1 nga2 nga4 were sterile, we introduced DR5-GFP into tcpDUO. The observation showed that the GFP fluorescence was significantly decreased in the apical gynoecium of tcpDUO DR5-GFP when compared to that of DR5-GFP in wild-type background (Supplementary Fig. S13), suggesting that tcpDUO may have lower auxin concentration in the apical gynoecium. To test whether the lower auxin biosynthesis caused the defects of the apical gynoecium of tcpDUO, we generated the construct CRCpro-YUC2 in which YUC2 gene was driven by the promoter of CRC. We transformed CRCpro-YUC2 into tcpDUO (Fig. 6, I to K). The statistical analysis showed that the expression of YUC2 partially rescued the longer style of tcpDUO (Fig. 6L). These results suggest that TCP4 interacts with CRC to cooperatively upregulate NGA genes, and NGA proteins subsequently form multimeric complexes to control auxin biosynthesis and the identity of the apical gynoecia.
EXB1 is repressed by TCP4 to tightly regulate style elongation
We previously demonstrated that EXB1/WRKY71 is a key regulator in the control of shoot branching (Guo et al. 2015). Our RNA-seq data showed that EXB1 and its homolog WRKY57 were significantly upregulated in tcpDUO (Fig. 4H). We used RT-qPCR to confirm that the expression level of EXB1 was significantly increased in tcpDUO, but unchanged in crc (Fig. 7A). EXB1 was upregulated to a lesser degree in tcpDUO crc (Fig. 7A), suggesting that EXB1 was not synergistically regulated by TCPs and CRC. These findings were consistent with the RNA-seq data (Fig. 4H). We searched for possible binding sites by analyzing our previous TCP4 ChIP-seq data for the EXB1 locus (Dong et al. 2019). We found an obvious TCP4 enrichment site in the promoter region of EXB1 (Fig. 7B), and gel EMSAs confirmed that TCP4 could directly bind to the identified site (Fig. 7C), suggesting that TCP4 may directly repress the expression of EXB1 during apical gynoecium development. To investigate the role of EXB1 in apical gynoecia, we observed the gynoecium phenotype of exb1-D, an activation tagging mutant in which EXB1 is overexpressed (Guo et al. 2015). Interestingly, overexpression of EXB1 caused obviously longer styles (Fig. 7, D and H), and the siliques of exb1-D correspondingly produced even longer styles (Fig. 7, E and I), resembling the style phenotypes exhibited by tcpDUO. We then re-examined the gynoecium phenotype of pEXB1–EXB1–SRDX lines, in which chimeric repressor silencing technology (CRES-T) was used to suppress the functions of EXB1 family members (Guo et al. 2015). We found that pEXB1–EXB1–SRDX-7 and pEXB1–EXB1–SRDX-26 produced shorter and thicker styles, with characteristics opposite those of the longer and thinner styles of tcpDUO and exb1-D (Fig. 7, F to H and I). These results suggest that TCP4 negatively regulates EXB1 at the transcriptional level to finely modulate the morphology of styles.
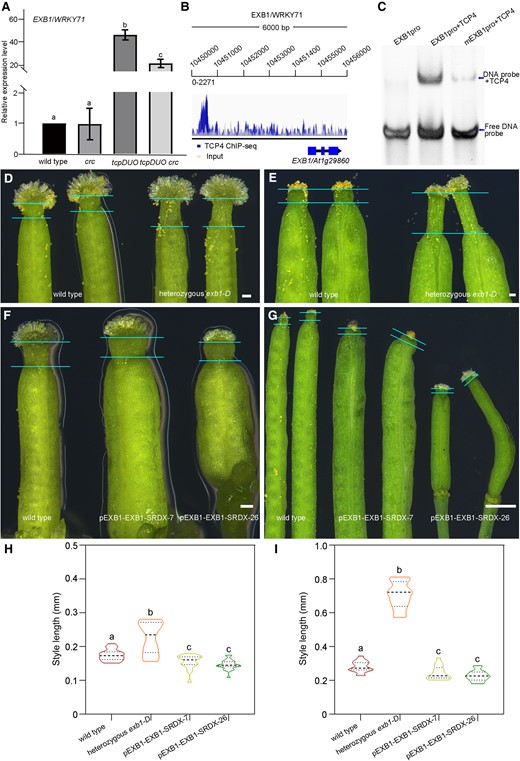
TCP4 directly binds to the EXB1 promoter to negatively regulate EXB1 expression to finely modulate the morphology of styles. A) Relative expression levels of EXB1 in the wild type, crc, tcpDUO, and tcpDUO crc apical gynoecium were detected by RT-qPCR. Actin2 was used as the internal reference. The reported results are the mean (±Sd) of three biological replicates. One-way ANOVA was performed with LSD/Duncan pairwise comparison testing. Different lowercase letters indicate significant differences (P < 0.05). B) ChIP-seq data showed that TCP4 protein was enriched in the promoter region of EXB1 in vivo. C) EMSA analysis indicated that TCP4 protein was directly bound to the promoter of EXB1 in vitro. D) Gynoecium from wild type and heterozygous exb1-D. Bars = 10 μm. E) Siliques from the apical gynoecium of wild type and heterozygous exb1-D. Bars = 1 mm. F) Gynoecium from wild type, pEXB1–EXB1–SRDX-7, and pEXB1–EXB1–SRDX-26. Bars = 10 μm. G) Siliques from the apical gynoecium of wild type, pEXB1–EXB1–SRDX-7, and pEXB1–EXB1–SRDX-26. Bars = 1 mm. H) Statistical analysis of the style lengths of wild type, heterozygous exb1-D, pEXB1–EXB1–SRDX-7, and pEXB1–EXB1–SRDX-26 apical gynoecium at flower development stage 11. The closed circles represent quartiles, and the short black lines represents the medians in the violin plot. Statistical significance was determined by one-way ANOVA (n ≥ 18). Significant differences at P < 0.05 are indicated by different lowercase letters (Supplementary Data Set 8). I) Statistical analysis of the style lengths of wild type, heterozygous exb1-D, pEXB1–EXB1–SRDX-7, and pEXB1–EXB1–SRDX-26 siliques. The closed circles represent quartiles, and the short black lines represents the medians in the violin plot. Statistical significance was determined by one-way ANOVA (n = 15). Significant differences at P < 0.05 are indicated by different lowercase letters (Supplementary Data Set 8).
Discussion
In this study, we demonstrate that TCP4 participates in the formation of the fine structure in the apical gynoecium. Disruption of TCP4 and other Class II TCPs in the septuple mutant tcpSEP and the duodecuple mutant tcpDUO leads to the formation of longer and thinner styles. The expression of TCP4 in the septuple mutant tcpSEP complements the defective phenotypes of the apical gynoecium. We also found that the mutant tcpDUO synergistically interacts with crc. In the tredecuple mutant tcpDUO crc, the style and stigma are replaced by lamellar structures with indeterminate growth activity. Correspondingly, genes encoding auxin-related proteins and transcription factors that play key roles in the development of apical gynoecia are synergistically downregulated in tcpDUO crc. Furthermore, we showed that TCP4 interacts with CRC and NGA transcription factors. We propose a working model for the function of TCP4 in the control of the apical gynoecium (Fig. 8). TCP4 and CRC are co-expressed in the developing apical gynoecia. TCP4 negatively regulates the expression of EXB1 to repress the elongation of styles. TCP4 also independently promotes the expression of NGA genes or forms a complex with CRC to jointly upregulate the transcription of NGA genes. NGA proteins directly bind to the promoter region of SSS2 to enhance its expression, and SSS2 protein facilitates the inhibition of style elongation. TCP4 and CRC independently regulate YUC genes, and also form multimeric complexes with NGAs to cooperatively regulate auxin biosynthesis, establishing the identity of the apical gynoecium (Fig. 8). Our findings reveal that TCP4 combines with different transcription factors to specifically regulate downstream genes, thus specifying the style and stigma of the gynoecium. We also illuminate the mechanisms underlying the finely tuned formation of the elegant structures required for the successful reception, selection and tube growth of pollen during plant reproduction.
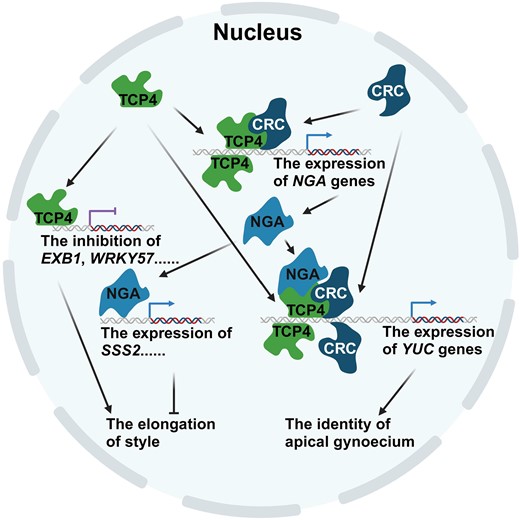
Working model of TCP transcription factors in the control of apical gynoecium identity (created with BioRender.com). TCP4 and CRC are co-expressed in the developing apical gynoecia. TCP4 directly binds to the promoters of EXB1 or NGAs. EXB1 is negatively regulated, and NGAs are positively regulated by TCP4 or TCP4–CRC complex. SSS2 is upregulated by NGAs. The downregulation of EXB1 and the upregulation of SSS2 inhibit the elongation of style. TCP4 and CRC independently upregulate or form complexes with NGAs to upregulate the expression of YUCs in determining the identity of the apical gynoecium.
As the most complex organ among the four flower whorls, the gynoecium is tightly regulated by multiple transcription factors that form a complex regulatory network by interacting with each other in a spatially and temporally regulated manner (Herrera-Ubaldo et al. 2023). For example, the floral quartet model suggests that AG interacts with SEPs to form quartet complexes that function in the specification of carpel identity, while complexes composed of AG, SEPs, STK, and SHP1/SHP2 specify the identity of ovules (Theißen et al. 2016). Ovule initiation is controlled by a complex comprised of ANT, YABBY1/FILAMENTOUS FLOWER (YAB1/FIL), and the SEU-LUG repressor (Azhakanandam et al. 2008). The apical gynoecium is also specified by the dynamic interactions among a specific set of transcription factors. ETT forms a transcriptional repressor complex with IND, RPL, BP, and SEU to specify the correct morphology of the apical gynoecium (Simonini et al. 2018). IND is directly upregulated by NGA–HEC complexes, and greater IND abundance leads to the formation of larger complexes with NGA–HEC, increasing SPT expression (Ballester et al. 2021). SPT forms higher order complexes with NGA–HEC–IND to ensure the proper distribution of auxin in gynoecium (Ballester et al. 2021). In this study, we showed that TCP4 functions in the patterning of the apical gynoecium. We found that TCP4 interacted with CRC to promote the expression of NGA genes, and NGAs formed higher order complexes to enhance auxin biosynthesis during apical gynoecium development. In addition, the expression of HEC1 was downregulated in the apical gynoecia of tcpDUO, crc, and tcpDUO crc in comparison with the wild-type control, suggesting that TCP4 and CRC also positively regulate HEC1 expression. Our data suggest that a longer sequentially activated pathway and larger transcriptional complexes might function in finely tuning the specification of the apical gynoecium. Specifically, TCP4 and CRC synergistically activate NGA and HEC1. NGAs interact with TCP4 and HEC1 to form larger TCP4–CRC–NGA–HEC1 complexes to regulate downstream target genes. Interestingly, the synergistical interaction of TCPs and NGAs has also been observed in the leaves and other organs. The leaves and some other organs of 35S:miR319a/35S:amiR-NGA plants in which TCPs and NGAs were downregulated by overexpression of miR319 and artificial microRNA targeting NGAs exhibit indeterminate growth (Alvarez et al. 2016), similar to the indeterminate growth in the apical gynoecium of tcpDUO nga1 nga2 nga4 and tcpDUO crc.
The establishment of an auxin maximum at the apical gynoecium is vital for the specification of the style and stigma (Larsson et al. 2013). Both auxin polar transport mediated by PINs and auxin local biosynthesis by YUCs play crucial roles in establishing and maintaining the asymmetric distribution of auxin along the apical–basal axis of the gynoecium (Cheng et al. 2006; Zhao 2008, 2018). Transcription factors coordinate to orchestrate auxin biosynthesis and transport to finely tune the spatial and temporal auxin distribution during development of the apical gynoecium. For example, the bHLH transcription factor SPT combines with IND to regulate auxin transport in the apical gynoecium (Girin et al. 2011). The disruption of HEC genes in the hec1 hec2 hec3 triple mutant significantly decreases the expression of YUC4, PIN1 and PIN3, indicating that HECs act as critical regulators of the auxin distribution by affecting both auxin biosynthesis and transport (Schuster et al. 2015). HEC1 directly regulates NGA1 and NGA2 (Gaillochet et al. 2018). NGAs function redundantly in the control of auxin biosynthesis by regulating YUC2, YUC4, YUC8, and TRYPTOPHAN AMINOTRANSFERASE OF ARABIDOPSIS1 (TAA1) (Martínez-Fernández et al. 2014). In addition, NGAs form transcriptional complexes with HEC, IND, and SPT to modulate auxin transport by regulating downstream genes, including PINOID (PID) and WAG2 (Ballester et al. 2021). NGAs also control the formation of the apical gynoecium in collaboration with SHI/STY family proteins (Gomariz-Fernández et al. 2017). These findings suggest that the determination of the apical gynoecium is a complex process, in which different types of transcription factors participate in dynamic transcriptional complexes that finely tune the expression level and/or pattern of genes key for auxin biosynthesis and transport in a spatial and temporal manner. As the direct target of AG, CRC synergistically promotes the expression of YUC4 with AG to control gynoecium formation and floral meristem determinacy (Yamaguchi et al. 2018). Additionally, CRC also regulates auxin homeostasis in the floral meristem by directly repressing TORNADO2 (TRN2) (Yamaguchi et al. 2017). It has been reported that TCP4 directly upregulates YUC5 to facilitate hypocotyl elongation (Challa et al. 2016). Moreover, CIN-like TCPs modulate the auxin response by directly activating INDOLE-3-ACETIC ACID3/SHORT HYPOCOTYL2 (IAA3/SHY2) and SMALL AUXIN UP RNA (SAUR) genes during leaf development (Koyama et al. 2010; Rath et al. 2022). We previously showed that TCP5 promotes the expression of YUC8 by directly binding to its promoter (Han et al. 2019). However, until now, it has been unclear whether Class II TCPs and CRC participate in establishing the auxin distribution and response in the apical gynoecium. In this study, we show that CRC and Class II TCPs play essential roles in the patterning of the apical gynoecium by regulating auxin biosynthesis. Many auxin-related genes are synergistically promoted by Class II TCPs and CRC during the specification of the apical gynoecium, including YUC2, YUC6, PIN3, PIN5, PIN7, IAA1, IAA3, IAA5, IAA7, IAA32, SAUR22, SAUR27, SAUR29, and SAUR68. Additional studies will be required to determine the precise manner in which Class II TCPs and CRC function coordinately with other transcription factors to finely tune the spatial and temporal auxin distribution and response in the apical gynoecium.
TCP family transcription factors play important and redundant roles during plant development. However, the roles of TCPs in gynoecium development are still largely unclear, possibly due to the high degree of redundancy among the members of TCP family, and sometimes among TCPs and other transcription factors. Overexpression of TCP15, a member of the Class I TCP group, causes the formation of unfused carpels and the reduction of tissues in the style and stigma. Inducing expression of TCP15-EAR, in which TCP15 is fused with a sequence encoding the ethylene-responsive element binding factor-associated amphiphilic repression (EAR) motif, overcomes the redundancy of Class I TCPs, leading to ectopic styles and stigmatic papillae along the replum (Uberti-Manassero et al. 2012; Lucero et al. 2015). These results suggest that Class I TCPs may negatively influence the formation of style and stigma tissues. Evidence demonstrating an extended interaction network of transcription factors suggests that TCP4, TCP10, and TCP13 are highly connected nodes related to gynoecium development (Herrera-Ubaldo et al. 2023), whereas their functions in gynoecium formation remain unclear. In this study, we demonstrate that Class II TCPs have highly redundant functions with CRC and NGAs in determining the identity of the apical gynoecium. TCP4 interacts with CRC and NGAs to form a high-order transcriptional complex in the specification of the style and stigma. It is proposed that Class I and Class II TCPs act antagonistically during leaf development (Martin-Trillo and Cubas 2010; Danisman et al. 2012). Interestingly, disruption of Class II TCPs and CRC in tcpDUO crc prevents production of the style and stigma, suggesting that Class II TCPs facilitate formation of the apical gynoecium, in contrast to the negative regulatory effect of Class I TCPs on the formation of style and stigma tissues. Therefore, specification of the style and stigma also might be antagonistically regulated by Class I and Class II TCPs. The generation of higher order mutants in which both Class I and Class II TCPs are disrupted could provide the necessary materials to illuminate the precise nature of these important interactions and their crucial roles in plant development.
Materials and methods
Plant materials and growth conditions
The Arabidopsis (A. thaliana) Columbia-0 (Col-0) ecotype was used as the wild-type control and the background for generating mutants in this study. T-DNA insertion mutants, including tcp2 (SALK_562_D05), tcp3 (wiscDSlox441C3), tcp4 (GABI_363H08), tcp5 (SM_3_29636), tcp10 (SALK_137205), and tcp13 (GABI_182B12), were crossed to generate tcp4 tcp10, tcp3 tcp4 tcp5 tcp10 tcp13, and tcp2 tcp3 tcp4 tcp5 tcp10 tcp13 mutants as reported previously (Zheng et al. 2022; Lan et al. 2023). The tcpSEP septuple mutant was reported previously (Lan et al. 2021). The tcpDUO duodecuple mutant was generated on the basis of the tcpSEP septuple mutant by genetic crosses and CRISPR/Cas9 technology (Wang et al. 2015). The tcp12/brc2 (SALK_023116), tcp16 (GABI_655C10), and tcp18/brc1 (SALK_091920) mutants are T-DNA insertion mutants from public databases. The tcp1, tcp24, tcpDUO crc, crc, and tcpDUO nga1 nga2 nga4 mutants were generated by CRISPR/Cas9 technology (Wang and Chen 2019). The exb1-D mutant and pEXB1-EXB1-SRDX mutant were reported previously (Guo et al. 2015). Arabidopsis seeds were sterilized (10 min in 75% (v/v) ethanol) and plated on half-strength Murashige and Skoog (1/2MS) solid medium with 10 g/L sucrose. The seeds were then placed in a 4°C refrigerator for 48 h of stratification. The plates were then put in 22 °C chambers under long-day conditions with 16 h light/8 h dark and 170 mmol/m2/s light intensity. The seedlings were planted in soil at the sixth day after germination, after which they were placed in a greenhouse under the same long-day conditions described above.
N. benthamiana seeds were directly grown in soil and placed in the greenhouse described above.
Genotyping and gene expression analysis
The T-DNA insertion mutant fragments from wild-type loci of tcp2, tcp3, tcp4, tcp5, tcp10, tcp12, tcp13, tcp16, tcp17, and tcp18 were amplified by using TCPx-G-F and TCPx-G-R primers. Fragments of the T-DNA insertion sites of the mutant were amplified with TCPx-T and TCPx-G-R or TCPx-G-F primers.
For genotyping of mutants generated by CRISPR/Cas9, including tcp1, tcp24, crc, nga1, nga2, and nga4, corresponding genomic fragments were amplified by the X-Genotyping-F and X-Genotyping-R primer. The mutation types were identified by sequencing.
For analysis of gene expression, the apical gynoecium samples were dissected from the flowers of wild type, crc, tcpDUO or tcpDUO crc mutants at flower developmental stage 11. To test gene expression at early developmental stage 9, the gynoecium tissues were dissected and collected from wild type and tcpDUO mutants due to the smaller flowers. The tissues were put in liquid nitrogen immediately after dissection. According to the manufacturer's instructions, the total RNA was extracted by the Plant RNA Extraction Kit (TaKaRa, Japan) and quantified by using a Nanodrop 2000 instrument (Thermo Scientific, USA). Reverse transcription of cDNA was performed using HiScript II Reverse Transcriptase (Vazyme Biotech Co. Ltd, China). Quantitative polymerase chain reaction (qPCR) was performed using ChamQ Universal SYBR qPCR Master Mix (Vazyme Biotech Co. Ltd, China). The primers for RT-qPCR analysis are listed in Supplementary Data Set 7, with the Actin2 as an internal control.
Plasmid construction and plant transformation
For complementation analysis, plasmid construction was performed as described previously (Lan et al. 2021). The 2,818 bp sequence containing TCP4 promoter and TCP4 coding region without the stop codon was amplified, and then cloned into the pENTR/D TOPO vector (Invitrogen) to generate pENTR-TCP4pg. The TCP4pro-TCP4-GFP construct was generated between pENTR-TCP4pg and pK7FWG0 (Invitrogen) by LR reaction. The 986 bp promoter of TCP10 and TCP10 coding region without the stop codon was amplified, and then cloned into the pENTR/D TOPO vector (Invitrogen) to generate pENTR-TCP10pg. The TCP10pro-TCP10-GFP construct was generated between pENTR-TCP10pg and pK7FWG0 (Invitrogen) by LR reaction. The 3,043 bp promoter of TCP1, 2,988 bp promoter of TCP2, 3,179 bp promoter of TCP3, 2,862 bp promoter of TCP13, 2,067 bp promoter of TCP17, 2,428 bp promoter of TCP18, were amplified, and then cloned into the pENTR/D TOPO vector (Invitrogen) to generate pENTR-TCP1p, pENTR-TCP2p, pENTR-TCP3p, pENTR-TCP13p, pENTR-TCP17p, and pENTR-TCP18p. The TCP1pro-GUS, TCP2pro-GUS, TCP3pro-GUS, TCP13pro-GUS, TCP17pro-GUS and TCP18pro-GUS constructs were generated between pENTR-TCP1p, pENTR-TCP2p, pENTR-TCP3p, pENTR-TCP13p, pENTR-TCP17p or pENTR-TCP18p and pB7GUSWG0 (Invitrogen) by LR reaction.
For transient expression in N. benthamiana leaves or Y2H assays, the coding regions of TCP4, TCP5, TCP12, TCP18, CRC, NGA1, NGA2, NGA3, and NGA4 were amplified and cloned into the pENTR/D TOPO vector to generate pENTR-X. As described previously, miR319-resistant TCP4 (mTCP4) was amplified by PCR-based mutagenesis using the primer pair TCP4-mut-F/TCP4-mut-R, and then cloned into pENTR/D TOPO to generate pENTR-mTCP4 (Lan et al. 2021). 35Spro-GFP-mTCP4 was generated by LR reaction between pENTR-mTCP4 and pK7WGF2 (Ghent University). The 35Spro-CRC-FLAG constructs were generated by LR reaction between pENTR-CRC and pB7FLAGWG2. A 2,095 bp region of the TCP12 promoter was amplified and inserted into pKGWFS7 vectors to prepare TCP12pro-GUS constructs. The constructs were introduced into Agrobacterium tumefaciens strain GV3101 for transient expression in N. benthamiana leaves, and Arabidopsis transgenic lines were generated using the floral dip method as described previously (Lan et al. 2021). All primers are listed in Supplementary Data Set 7.
CRISPR/Cas9-induced mutants
A CRISPR/Cas9 system containing an egg cell-specific promoter was used to generate tcp1 and tcp24 as previously described (Wang et al. 2015). To generate the tredecuple mutant tcpDUO crc and quindecuple mutant tcpDUO nga1 nga2 nga4, a CRISPR/Cas9 system containing a UBIQUITIN10 (UBQ10) promoter was used (Wang and Chen 2019). The spacer oligonucleotides were designed on the target gene sequences, ligated into pAtU6-26-M vectors, and cloned into pCAMBIA1300-UBQ10-Cas9-P2A-GFP. The mutated fragments were amplified by using PCR and identified by sequencing. The oligonucleotides and primers used for CRISPR/Cas9-induced mutants are listed in Supplementary Data Set 7.
Microscopy analysis
For the observation of the apical gynoecium, the flowers of tcpSEP, tcpDUO and wild-type control were emasculated at flower developmental stage 11. The apical gynoecium was observed using a Leica M205 FCA stereoscope at 24 h after emasculation.
The observation of DR5-GFP was reported previously (Martínez-Fernández et al. 2014). Briefly, the flowers of DR5-GFP tcpDUO and DR5-GFP were emasculated and the apical gynoecium was observed at the same excitation light intensity using a Leica TCS SP8 confocal laser scanning microscope. GFP emission spectra were collected between 500 and 550 nm and plastid autofluorescence was collected between 650 and 750 nm.
For SEM observation, samples were prepared in accordance with the user's manual of FEI Helios Nanolab G3 UC. Images were captured by the EasyLift EX NanoManipulator and Auto Slice and View G3 software.
Measurement of style length
At flower stage 11, the flowers of different genotypes were emasculated. The style length was measured using ImageJ software (v.1.38, National Institutes of Health; http://rsb.info.nih.gov/ij) at 24 h after emasculation. The average style length was calculated, and statistics were performed with SPSS statistics software (v.17.0). The data were analyzed using one-way ANOVA followed by LSD and Student–Newman–Keuls (S–N–K) post hoc analysis.
GUS staining
GUS staining was performed as previously described (Zheng et al. 2022). GUS activity was detected in the flowers of TCP1pro-GUS, TCP2pro-GUS, TCP3pro-GUS, TCP4pro-TCP4-GUS, TCP5pro-GUS, TCP12pro-GUS, TCP13pro-GUS, TCP17pro-GUS, TCP18pro-GUS and transgenic plants at different flower stages. The flowers of TCP1pro-GUS, TCP2pro-GUS, TCP3pro-GUS, TCP4pro-TCP4-GUS, TCP5pro-GUS, TCP12pro-GUS, TCP13pro-GUS, TCP17pro-GUS and TCP18pro-GUS plants were transferred to GUS staining buffer containing 5-bromo-4-chloro-3-indolyl glucuronide at 37 °C overnight, after which they were placed in 75% ethanol three or four times within 30 min. Finally, the samples were stored in 75% ethanol. The histochemical localization of GUS activity in tissues was determined using a Leica M205 FCA stereoscope.
Structural observation and microscopy
The gynoecium was collected and fixed in 4% paraformaldehyde overnight, then washed with 0.1 M PBS and hydrated sequentially in 15%, 30%, 50%, 70%, 85%, 95%, and 100% ethanol for 1 h per step. The dehydrated samples were transferred into a series of mixtures of ethanol and LR white (Electron Microscopy Sciences, 14381-UC) (3:1→2:1→1:1→1:2→1:3) for 2 h per step, and cleared in pure LR white for 18 h before transferred to 65 °C overnight. Transverse sections of 10 μm were cut using a microtome (Leitz 1512), stained with 1% toluidine blue, and photographed under a Zeiss Axio Imager M2 microscope using the DIC channel.
RNA-seq analysis
For the RNA-seq analysis, the flowers of wild type, crc, tcpDUO, and tcpDUO crc were emasculated at stage 11. The apical gynoecium, including the style and stigma, were isolated at 24 h after emasculation for total RNA extraction. Total RNA was isolated using a Plant RNA Extraction Kit (TaKaRa, Japan). RNA-seq library preparation and high-throughput sequencing were performed by Novogene Inc. (Tianjin, China). The libraries were sequenced on an Illumina NovaSeq 6000 platform by generating 150 bp paired-end reads. Quality control of the raw reads was performed by TrimGalore (https://www.bioinformatics.babraham.ac.uk/projects/trim_galore/). The clean reads were mapped to the Arabidopsis reference genome (TAIR10) by STAR (Dobin et al. 2013). Expression counts were generated by featureCounts of Subread 2.0.1 with default parameters (Liao et al. 2014). The Cufflinks assembler and abundance estimation algorithms were used for gene expression analysis (http://cufflinks.cbcb.umd.edu/). Genes with a Bonferroni-adjusted P-value (padj) ≤ 0.05 and a log2 fold change ≤ −1 or ≥ 1 were considered to be significantly differentially expressed. The free online tool Venny was used to generate Venn diagrams. An online interactive tool was used to compare lists with Venn diagrams (https://bioinfogp.cnb.csic.es/tools/venny/index.html). GO analysis was performed using the publicly accessible platform agriGO (http://systemsbiology.cau.edu.cn/agriGOv2/) (Tian et al. 2017). Gene ratio represents the number of input genes that was annotated in a certain GO term/total number of Arabidopsis genes (TAIR10) that was annotated in the GO database. Heatmap plots were generated using the R package ggplot2 (Wickham 2016).
Electrophoretic mobility shift assay
The TCP4 DNA-binding domain was amplified and cloned into pET-21b with His-tag, and then the vector was transformed into Escherichia coli strain BL21. After the bacteria were cultured at 18 °C overnight, 0.5 mM IPTG was added to induce the protein. After the cells were collected, they were resuspended in buffer A (25 mM Tris and 1 M NaCl [pH 8.0]). The target protein enriched on Ni chelating columns (GE Healthcare, USA) was eluted with 200 to 500 mM imidazole. The crude protein was subjected to size-excluded chromatography (Superdex 75, GE Healthcare) to obtain the purified protein.
DNA fragments containing a potential TCP4 binding motif (GGNCCAC) upstream of EXB1/NGA1/YUC2 were synthesized. The binding motif was mutated to AACTTGT in the mutated DNA fragments. Double-stranded DNA was obtained by annealing the DNA fragments with equimolar concentrations of annealing buffer (25 mM Tris and 100 mM NaCl [pH 8.0]). Double-stranded DNA (about 200 ng) was mixed with purified TCP4 DNA-binding domain proteins (about 180 ng). The mixture was incubated at 4 °C for 20 min, and then loaded on 7% nondenaturing polyacrylamide gel for electrophoresis with 0.5× TBE buffer. Electrophoresis was performed at 120 V for 40 min. DNA was detected using ethidium bromide staining under the Tanon 2500 gel imaging system.
GUS/LUC activity determination
Transactivation assays were performed as previously described (Zhao et al. 2021). To analyze the activation of the YUC2 promoter by TCP4, the constructs 35Spro-mTCP4 in pCAMBIA1300 vector, YUC2pro-GUS in the pCAMBIA1391 vector and the vector containing the firefly luciferase (LUC) gene driven by the 35S promoter were co-transfected into A. tumefaciens strain (GV3101). GUS activity was measured using a fluorometric assay on an F-7000 fluorescence spectrophotometer (Hitachi, Tokyo, Japan), with 4-methylumbelliferyl-β-d-glucuronide (MUG) as a substrate. LUC activities were measured using a Promega GLOMAX 20/20 luminometer. GUS activities were normalized to the LUC activities, and the GUS/LUC ratios were calculated. The relative GUS/LUC activity without TCP4 was used as the control.
Yeast two-hybrid assays
Y2H assays were performed as described previously (Lan et al. 2021). To test the interaction between TCP proteins with NGA proteins and CRC in yeast, bait constructs were generated by LR reactions between pENTR-CRC/NGA1/NGA2/NGA3/NGA4 and pDEST32 (Invitrogen). The prey constructs were generated by LR reactions between pENTR-TCP4/TCP5/TCP12/TCP18 and pDEST22 (Invitrogen). The primers for the Y2H assay are listed in Supplementary Data Set 7.
Both bait and prey constructs were co-transformed into Saccharomyces cerevisiae strain AH109. The blank pDEST22 was co-transformed with each prey as a negative control. Yeast selection was performed by the medium supplemented with SD-Leu-Trp-His with or without 5 mM 3-amino- 1,2,4-triazole (3-AT).
Luciferase complementation imaging assay
The LCI assay was conducted according to a previously described method (Lan et al. 2021). The pCAMBIA1300-cLUC-GW and pCAMBIA1300-nLUC-GW vectors were used. To test the interaction between TCP4 and CRC, NGA1, NGA2, NGA3 or NGA4 in planta, 35Spro-cLUC-mTCP4, 35Spro-CRC-nLUC, 35Spro-NGA1-nLUC, 35Spro-NGA2-nLUC, 35Spro-NGA3-nLUC, and 35Spro-NGA4-nLUC were generated by LR reactions between pCAMBIA1300-cLUC-GW or pCAMBIA1300-nLUC-GW and pENTR-mTCP4, pENTR-CRC, pENTR-NGA1, pENTR-NGA2, pENTR-NGA3 or pENTR-NGA4.
The constructed plasmids were transformed into A. tumefaciens strain (GV3101) competent cells and briefly expressed in N. benthamiana leaves for 2 d in different combinations. The N. benthamiana leaves were dissected and 200 μM luciferin was injected. After dark processing for 10 min, a low-light cooled CCD imaging apparatus (NightOWL II LB983) with indiGO software was used to take images, and the exposure time was 3 min.
Co-immunoprecipitation assay
Co-IP assays were performed in N. benthamiana leaves that expressed 35S-CRC-Flag and 35S-GFP-mTCP4, and 35S-CRC-Flag and 35S-GFP were co-transformed into N. benthamiana leaves as a control. The two combinations were injected into N. benthamiana leaves for 2 d. Samples were ground into powder with liquid nitrogen, then 1 mg powder was mixed into the extraction buffer (0.1 M HEPES (pH 7.5), 5 mM EGTA, 5 mM EDTA, 5 mM NaF, 0.05 M glycerin phosphate, 1% Triton X-100, 10% glycerin, protease inhibitor cocktail, PMSF) on ice for 1 h. The samples were then centrifuged at 4 °C for 30 min. The supernatant was incubated with 30 µL anti-GFP beads (MBL, Japan) for 2 h and the beads were washed with 1× PBS buffer. The interaction with anti-FLAG antibody (Sigma, USA; 1:5,000 dilution) was detected.
Statistical analysis
Statistical analyses were performed as described in the figure legends. Statistical data are provided in Supplementary Data Set 8.
Accession numbers
Sequence data from this article can be found in Arabidopsis Genome Initiative database under the following accession numbers: TCP1 (AT1G67260), TCP2 (AT4G18390), TCP3 (AT1G53230), TCP4 (AT3G15030), TCP5 (AT5G60970), TCP10 (AT2G31070), TCP12 (AT1G68800), TCP13 (AT3G02150), TCP16 (AT3G45150), TCP17 (AT5G08070), TCP18 (AT3G45150), TCP24 (AT1G30210), CRC (AT1G69180), NGA1 (AT2G46870), NGA2 (AT3G61970), NGA3 (AT1G01030), NGA4 (AT4G01500), EXB1 (AT1G29860), YUC2 (AT4G13260), YUC6 (AT5G25620).
Acknowledgments
We thank Professor Yuval Eshed (Weizmann Institute of Science, Israel) for kindly providing the tcp5 tcp13 tcp17 triple mutant seeds. We thank Professor Tomotsugu Koyama (Kyoto University) for kindly providing the seeds of tcp3 tcp4 tcp10 and tcp3 tcp4 tcp5 tcp10 tcp13. We also thank the Core Facilities of Life Sciences, Peking University and the National Center for Protein Sciences at Peking University for assistance with the SEM assay. We are grateful to Dr. Yiqun Liu, Miss Yingying Guo, Ms. Li Zhang for technical support for the SEM analysis.
Author contributions
G.Q. conceived and designed the project. Y.W., N.W., J.L., Y.J., and Y.W. conducted the experiments. G.Q., Y.W., N.W., J.L., Y.P., Y.J., X.C., and X.F. analyzed the data. G.Q., Y.W., N.W., and J.L. wrote the manuscript.
Supplementary data
The following materials are available in the online version of this article.
Supplementary Figure S1. Schematic diagram of the tcp12, tcp16, tcp18, tcp1, and tcp24 mutants used for the generation of tcpDUO.
Supplementary Figure S2. No significant differences were observed in cell size between tcpDUO and wild type.
Supplementary Figure S3. The apical gynoecium internal structure of wild type and tcpDUO were observed using semithin section.
Supplementary Figure S4. TCP transcription factors redundantly controlled the apical gynoecium.
Supplementary Figure S5. The styles became narrower and longer in the apical gynoecia of the tcpSEP or tcpDUO siliques.
Supplementary Figure S6. Schematic diagram of crc mutants used for the generation of tcpDUO crc.
Supplementary Figure S7. The expression patterns of TCP1, TCP2, TCP3, TCP13, TCP17, and TCP18 at different flower developmental stages.
Supplementary Figure S8. PCA of RNA-seq data of wild type, crc, tcpDUO, and tcpDUO crc apical gynoecium.
Supplementary Figure S9. Relative expression levels of downstream genes in the gynoecium of wild type and tcpDUO at flower developmental stage 9.
Supplementary Figure S10. TCP4 protein enrichment diagram based on ChIP-seq data.
Supplementary Figure S11. TCP4 directly bound to the promoters of NGA1/YUC2 and regulated the expression of YUC2.
Supplementary Figure S12. Schematic diagram of the nga1, nga2, and nga4 mutants used for the generation of tcpDUO nga1 nga2 nga4.
Supplementary Figure S13. The fluorescence intensity of the apical gynoecium of DR5-GFP and DR5-GFP tcpDUO.
Supplementary Data Set 1. Genes upregulated in crc mutant.
Supplementary Data Set 2. Genes downregulated in crc mutant.
Supplementary Data Set 3. Genes upregulated in tcpDUO mutant.
Supplementary Data Set 4. Genes downregulated in tcpDUO mutant.
Supplementary Data Set 5. Genes upregulated in tcpDUO crc mutant.
Supplementary Data Set 6. Genes downregulated in tcpDUO crc mutant.
Supplementary Data Set 7. Primers used in this study.
Supplementary Data Set 8. Statistical tests for figures.
Funding
This research was supported by the National Natural Science Foundation of China (Youth project) (Grant No. 32300291), the China Postdoctoral Science Foundation (Grant No. 2023M730083), and the National Science Fund for Distinguished Young Scholars of China (Grant No. 31725005).
Data availability
The data that support the findings of this study are available from the corresponding authors upon request. The high-throughput sequencing data used in this study were deposited in the Sequence Read Archive (SRA) database with the accession number PRJNA984960 for RNA-seq.
Dive Curated Terms
The following phenotypic, genotypic, and functional terms are of significance to the work described in this paper:
References
Author notes
Yutao Wang and Ning Wang contributed equally to this work.
The author responsible for distribution of materials integral to the findings presented in this article in accordance with the policy described in the Instructions for Authors (https://dbpia.nl.go.kr/plcell/pages/General-Instructions) is: Genji Qin ([email protected]).
Conflict of interest statement. None declared.