-
PDF
- Split View
-
Views
-
Cite
Cite
Annalisa John, Moritz Krämer, Martin Lehmann, Hans-Henning Kunz, Fayezeh Aarabi, Saleh Alseekh, Alisdair Fernie, Frederik Sommer, Michael Schroda, David Zimmer, Timo Mühlhaus, Helga Peisker, Katharina Gutbrod, Peter Dörmann, Jens Neunzig, Katrin Philippar, Horst Ekkehard Neuhaus, Degradation of FATTY ACID EXPORT PROTEIN1 by RHOMBOID-LIKE PROTEASE11 contributes to cold tolerance in Arabidopsis, The Plant Cell, Volume 36, Issue 5, May 2024, Pages 1937–1962, https://doi.org/10.1093/plcell/koae011
- Share Icon Share
Abstract
Plants need to acclimate to different stresses to optimize growth under unfavorable conditions. In Arabidopsis (Arabidopsis thaliana), the abundance of the chloroplast envelope protein FATTY ACID EXPORT PROTEIN1 (FAX1) decreases after the onset of low temperatures. However, how FAX1 degradation occurs and whether altered FAX1 abundance contributes to cold tolerance in plants remains unclear. The rapid cold-induced increase in RHOMBOID-LIKE PROTEASE11 (RBL11) transcript levels, the physical interaction of RBL11 with FAX1, the specific FAX1 degradation after RBL11 expression, and the absence of cold-induced FAX1 degradation in rbl11 loss-of-function mutants suggest that this enzyme is responsible for FAX1 degradation. Proteomic analyses showed that rbl11 mutants have higher levels of FAX1 and other proteins involved in membrane lipid homeostasis, suggesting that RBL11 is a key element in the remodeling of membrane properties during cold conditions. Consequently, in the cold, rbl11 mutants show a shift in lipid biosynthesis toward the eukaryotic pathway, which coincides with impaired cold tolerance. To test whether cold sensitivity is due to increased FAX1 levels, we analyzed FAX1 overexpressors. The rbl11 mutants and FAX1 overexpressor lines show superimposable phenotypic defects upon exposure to cold temperatures. Our results show that the cold-induced degradation of FAX1 by RBL11 is critical for Arabidopsis to survive cold and freezing periods.
Background: Plants are exposed to constantly changing environmental conditions, and temperature differences, such as a cold snap, can occur relatively quickly. A key mechanism for acclimation to low temperatures is the reorganization of the protein and lipid composition of organellar membranes to keep the membranes fluid and protect cell integrity. For example, at cold temperatures, cryoprotective galactolipids are increasingly incorporated into membranes. These lipids are generated via the prokaryotic lipid biosynthesis pathway within the chloroplast. Accordingly, only small amounts of fatty acids (FAs) are usually exported from the plastid at cold temperatures and used in the endoplasmic reticulum to synthesize phospholipids via the eukaryotic lipid biosynthesis pathway.
Questions: Is the protein abundance of chloroplast FATTY ACID EXPORT PROTEIN1 (FAX1) regulated by the envelope serine protease RHOMBOID-LIKE11 (RBL11) under cold growth conditions? Will unregulated FA export from the plastid affect cold and frost tolerance in Arabidopsis (Arabidopsis thaliana)?
Findings: Several interaction experiments revealed that FAX1 is a potential substrate of the envelope protease RBL11, whereby FAX1 is degraded only under cold conditions. Investigation of Arabidopsis FAX1 overexpression lines and rbl11 mutants showed a similar phenotype on the molecular level. This includes strong reactive oxygen species production and a shift in lipid biosynthesis toward the eukaryotic pathway compared with wild-type plants. FAX1 overexpression and rbl11 loss-of-function mutants overproduce phospholipids compared with galactolipids due to unregulated FA export, due to high FAX1 abundance, from the plastid. As consequence, FAX1 overexpression lines and rbl11 mutants exhibit clear cold and frost sensitivity. In summary, regulation of FA export via FAX1 by the protease RBL11 is crucial for proper acclimation of Arabidopsis to cold growth conditions.
Next steps: Future studies are required to test whether RBL11 directly degrades FAX1. It will also be of interest to investigate whether posttranslational modifications regulate the interaction of both proteins.
Introduction
Plants have remarkable abilities to cope with a wide range of environmental conditions. When light intensity, temperature, or water and nutrient availability leave certain ranges, corresponding stress stimuli trigger systemic responses such as genetic, metabolic, and, to some extent, morphological changes. These processes lead to a new metabolic homeostasis that allows the plant to cope with the environmental challenge (Obata and Fernie 2012; Koevoets et al. 2016; Choudhury et al. 2017; Pommerrenig et al. 2018; Garcia-Molina et al. 2020; Wang et al. 2020).
Changes in growth temperature are usually more rapid than changes in water or nutrient availability. Accordingly, plants must rapidly initiate the appropriate acclimation programs. These efficient molecular responses are essential because temperature affects the 2 main processes of photosynthesis, i.e. the light-driven electron transport across the thylakoid membrane and the subsequent enzyme-catalyzed Calvin–Benson cycle, in different ways. Thus, photosynthesis is markedly responsive to temperature changes, as demonstrated in several species representing a broad spectrum of CO2-fixing organisms (Lin et al. 2012; Mackey et al. 2013; Walker et al. 2013; Song et al. 2014).
The composition of membrane lipids in plant cells undergoes dynamic remodeling after onset of low temperatures (Moellering et al. 2010; Li, Gügel, et al. 2015; Li, Zheng, et al. 2015; Barnes et al. 2016; Barrero-Sicilia et al. 2017). These structural changes include a higher degree of desaturation and altered abundancies of different phospho-, sulfo-, or galactolipid species, which ensure sufficient extent of membrane fluidity under unfavorable environmental conditions (Smallwood and Bowles 2002).
In plants, lipid biosynthesis represents a complex metabolic network in which initial metabolic steps in the plastids (e.g. chloroplasts) are connected to subsequent processes at the endoplasmic reticulum (ER) (Li-Beisson et al. 2010; Nakamura 2017; Hölzl and Dörmann 2019; Lavell and Benning 2019). Generally, for the de novo synthesis of both classes of lipids, namely storage lipids (triacylglycerols, TAG) or membrane lipids (in chloroplasts mainly glyco- and phosphoglycerolipids and extraplastidic membranes, phosphoglycerolipids, sphingolipids, and sterol lipids), fatty acid (FA) synthesis in plastids provides acyl-chains for subsequent steps in the plastid and the ER (Rawsthorne 2002; Li-Beisson et al. 2010). Accordingly, the subsequent lipid biosynthesis takes either place via the plastid located “prokaryotic pathway” or via the ER located “eukaryotic pathway” (Roughan and Slack 1982).
TAG is synthesized in the ER, but the biosynthesis of membrane lipid occurs in the ER and plastids (Li-Beisson et al. 2010). During membrane lipid synthesis, the usage of FAs in chloroplasts or in the ER leads to different types of structural lipids. Generally, plastids represent the major site for phosphatidylglycerol (PG) synthesis and the exclusive site for mono- and digalactosyl-diacylglycerol (MGDG and DGDG) biosynthesis, as well as for sulfoquinovosyl-diacylglycerol (SQDG) assembly. The ER and plastids are responsible for the provision of diacylglycerol (DAG) backbones, which serve as a precursor for all ER-produced phospholipids, including phosphatidylcholine (PC) and phosphatidylethanolamine (PE) (Hagio et al. 2002; Andersson and Dörmann 2009; Li-Beisson et al. 2010; Lavell and Benning 2019). (Hagio et al. 2002; Andersson and Dörmann 2009; Li-Beisson et al. 2010; Lavell and Benning 2019). DAG synthesis in chloroplasts is particularly pronounced under cold conditions because the SENSITIVE TO FREEZING2 (SFR2) enzyme transfers galactosyl groups from MGDG to other galactolipids, resulting in the formation of oligogalactolipids (Moellering et al. 2010; Barnes et al. 2016). Besides the plastid-produced DAG, the ER-derived DAG moieties also act, after import into plastids, as precursors for plastid lipid biosynthesis (Li-Beisson et al. 2010).
Each membrane type is defined by a characteristic lipid composition. This composition is dynamic in response to changing environmental conditions with individual lipid mixtures giving rise to specific membrane properties (van Meer et al. 2008; Li-Beisson et al. 2010; Moellering et al. 2010). For example, at low temperatures, lipid remodeling maintains membrane fluidity to prevent ion leakage, to keep carrier and receptor proteins functional or to integrate novel protective proteins (Steponkus et al. 1977; Barrero-Sicilia et al. 2017). Accordingly, plant mutants exhibiting altered activities of (i) selected FA biosynthesis enzymes, (ii) FA desaturases, (iii) lipid transfer proteins, or (iv) lipases might exhibit modified cold tolerance and photosynthesis properties (Miquel et al. 1993; Welti et al. 2002; Khodakovskaya et al. 2006; Guo et al. 2013; Gao et al. 2020; Schwenkert et al. 2023). The latter studies emphasize the impact of a proper membrane lipid remodeling after the onset of low temperatures. In fact, it has been shown for various species that low environmental temperatures lead to an upregulation of the chloroplast-located lipid biosynthesis pathway (Li, Gügel, et al. 2015; Li, Zheng, et al. 2015). Although several proteins involved in this process have been identified, the precise regulation responsible for this metabolic shift is still unknown.
In contrast to chloroplasts, which are the site of FA de novo synthesis in the cell (Li-Beisson et al. 2010), the lipid biosynthesis pathway localized in the ER depends on import of FAs from plastids. The molecular nature of transport proteins mediating export of newly synthesized FAs from plastids had remained unknown for a long time (Wang and Benning 2012). However, with the identification of the protein FATTY ACID EXPORT PROTEIN1 (FAX1), a first candidate was identified (Li, Gügel, et al. 2015; Li, Zheng, et al. 2015) and the ability of FAX1 to promote shuttling of FAs across membranes has been shown in recombinant baker's yeast cells (Li, Gügel, et al. 2015; Li, Zheng, et al. 2015). Furthermore, the absence of FAX1, which resides in the plastid inner envelope membrane, leads to male sterility (Li, Gügel, et al. 2015; Li, Zheng, et al. 2015; Zhu et al. 2020) and decreased levels of ER-derived eukaryotic lipids but increased relative contents of PG, which is synthesized via the prokaryotic pathway. In contrast, FAX1 overexpressor lines exhibit an increased level of lipids assembled via the eukaryotic pathway, e.g. more TAG (Li, Gügel, et al. 2015; Li, Zheng, et al. 2015).
The chloroplast serves as a cellular hub coordinating genetic and molecular responses required to acclimate to altered environmental conditions (Schwenkert et al. 2022). Thus, all signals and each metabolite emitted from or received by the chloroplast must pass the inner envelope membrane. Therefore, the inner envelope proteome undergoes profound changes in response to the onset of stress conditions (Nishimura et al. 2016; Pottosin and Shabala 2016; Wagner et al. 2016). Strikingly, FAX1 belongs to a group of inner envelope proteins which exhibit a proteolytic degradation after exposure to low temperatures, while other inner envelope proteins increase in their abundancy (Trentmann et al. 2020). The mechanism responsible for the decrease of FAX1 in response to cold temperatures is elusive. In addition, it is unknown whether this phenomenon represents an important molecular process required for a maximal tolerance against low temperatures and/or frost.
Chloroplasts contain more than 2,000 different soluble or membrane-bound proteins (Abdallah et al. 2000), and more than 20 different proteases ensure proteome homeostasis within the organelle (Nishimura et al. 2016). So far, 2 major classes of proteases have been identified to cleave intrinsic inner envelope proteins, the metallo-dependent AAA type FTSH proteases (with the isoforms FTSH 7, 9, 11, and 12), and the rhomboid-like (RBL) proteases (with the isoforms RBL 10 and 11) (Knopf et al. 2012; Wagner et al. 2016; Adam et al. 2019).
Thus, the function of FAX1 and the pronounced impact of lipid remodeling on plant acclimation to cold raise 2 timely questions: first, what is the mechanism responsible for the rapid decline of FAX1 upon onset of cold? Second, is the cold-induced downregulation of FAX1 a pleotropic response or is it important for low temperature tolerance? To answer both questions, we searched for an interaction of a selective protease with FAX1. It turned out that FAX1 and RHOMBOID-LIKE 11 (RBL11) can physically interact and that this protease is responsible for the cold-induced downregulation of FAX1. In addition, we have shown that under cold conditions, rbl11 mutants exhibit physiological and molecular phenotypes similar to FAX1 overexpressors. Furthermore, we show that a lack of FAX1 degradation in response to cold impairs the ability of Arabidopsis plants to cope with low temperature and freezing. These findings contribute to our understanding of how plants tolerate adverse environmental conditions, one of the most impressive traits required to ensure plant productivity.
Results
Transcript abundance of the plastid protease RBL11 responds to cold treatments
It was previously shown that the protein FAX1 belongs to a small group of inner envelope proteins which are downregulated in their abundance upon onset of cold temperatures (Trentmann et al. 2020). While FTSH12 is critical for chloroplast development (Mielke et al. 2020), FTSH11 and RBL10 activity affect either the temperature response or the membrane lipid composition, respectively (Chen et al. 2006; Wagner et al. 2011; Lavell et al. 2019). Thus, in addition to FTSH11 and RBL10, we initially focused on RBL11 (the, besides RBL10, only other rhomboid-like protease at the inner envelope) which was shown to be active in fully developed mesophyll chloroplasts (Knopf et al. 2012).
To gain insight into possible molecular interactions between the proteases and FAX1, we analyzed the transcription level of corresponding genes after transfer to 4 °C (Fig. 1A), the standard temperature in our laboratory to study cold effects (Patzke et al. 2019; Cvetkovic et al. 2021). Initially, transcript levels of all 3 envelope proteases accumulated in response to the shift toward cold growth conditions. After 1 wk at 4 °C, the most pronounced RBL11 mRNA change was detected, which had about 14 times higher abundance when compared with the beginning of this treatment (Fig. 1A). Both, RBL10 and FTSH11 mRNA amounts peaked at 4 or 7 times higher levels, respectively, after 7 d of cold treatment versus the start of the cold period (Fig. 1A). The highest levels of all 3 mRNAs were present after 14 d in cold conditions, and from then on, these 3 transcript levels declined in their abundance (Fig. 1A).
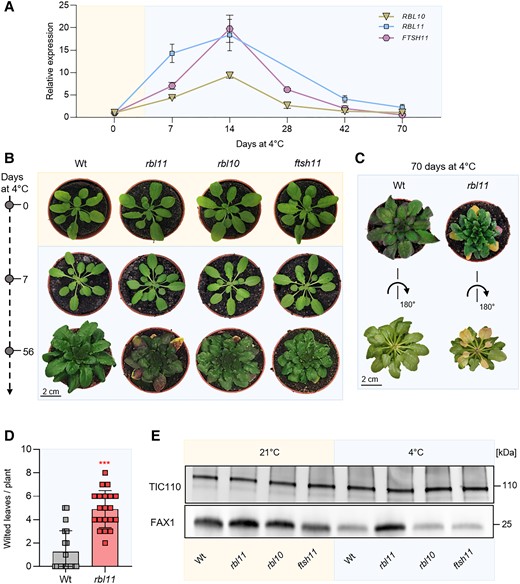
Gene expression, phenotypic, and immunoblot analysis of Arabidopsis protease loss-of-function mutants rbl11, rbl10, and ftsh11 grown under standard and cold (4 °C) conditions. Plants were grown under standard conditions (21 °C day and night temperature, 10 h day length and 120 µE light intensity) for 3 wks and then treated with cold (4 °C day and night temperature, 10 h day length, and 120 µE light intensity). A) Gene expression levels of RBL11, FTSH11, and RBL10 in the Wt by qRT-PCR under standard growth conditions (0 d at 4 °C) and several days during cold treatment (7, 14, 28, 42, and 70 d at 4 °C). Data represent relative mean expression levels of 3 biological replicates and are normalized to standard conditions (0 d at 4 °C) using UBQ as an internal control. B) Phenotypic analysis of rbl11, rbl10, and ftsh11 Arabidopsis plants. Images were taken from plants grown under standard conditions and after chilling for 7 and 56 d. C) Wt and rbl11 rosettes were cut after 70 d of cold treatment and rotated to highlight the wilted leaves. D) Number of wilted leaves per plant in Wt and rbl11 after 70 d of cold treatment. E) Immunoblot analysis via FAX1 antibody in isolated chloroplast envelopes of Wt, rbl11, rbl10, and ftsh11 from plants grown under standard conditions (21 °C) and plants grown for 7 d at 4 °C. Immunoblot detection via Tic110 antibody is used as a control and shows an equal loading of the protein samples at 3 µg per lane. Error bars in A) represent ± Sem. Error bars in D) are ± Sd. Asterisks indicate significant differences between Wt and mutant using a t test: ***P-value ≤ 0.001 (Supplementary Data Set 3).
Rbl11 mutants exhibit impaired growth at cold temperature since RBL11 controls FAX1 abundance in the cold
To search for specific responses in mutants lacking 1 of the 3 proteases, we grew wild-type (Wt) and respective loss-of-function lines under either control conditions (21 °C) or for 7 or 56 d at 4 °C.
When grown at 21 °C, none of the mutants exhibited a phenotypic pattern distinct from Wt plants (Fig. 1B). When grown at 4 °C for 7 d, all genotypes showed slightly decreased chlorophyll levels, as indicated by brighter leaves (Fig. 1B). However, after 56 d at 4 °C, all mutant plants exhibited impaired growth, i.e. smaller rosette sizes when compared with the Wt (Fig. 1B). We previously showed that plants with impaired tolerance against cold temperatures exhibited increased numbers of wilted leaves when exposed to 4 °C (Trentmann et al. 2020). Interestingly, after 56 d at 4 °C, rbl11 mutants exhibited wilted leaves (Fig. 1B). This effect was further exacerbated in rbl11 plants after 10 wks at 4 °C (Fig. 1C, pictures from top and bottom of the rosette). A quantification of this observation showed that after 10 wks at 4 °C, Wt exhibited 1.25 wilted leaves/plant, while rbl11 mutants displayed in 5 wilted leaves per plant on average (Fig. 1D).
The observation that from all 3 proteases tested the RBL11 mRNA is the fastest responding transcript within 7 d after transfer to 4 °C (Fig. 1A) rendered the corresponding enzyme a prime candidate for cold-induced FAX1 degradation. To test the effect of RBL11 on FAX1 levels, we isolated chloroplast envelopes from Wt and rbl11 loss-of-function mutant plants and conducted immunoblots using a previously established FAX1 antibody (Li, Gügel, et al. 2015; Li, Zheng, et al. 2015). The parallel immunoblotting of the inner envelope protein TRANSLOCON AT THE INNER ENVELOPE MEMBRANE OF CHLOROPLASTS 110 (TIC110) (Balsera et al. 2009) confirmed similar levels of total envelope proteins in each lane (Fig. 1E). Interestingly, already in rbl11 mutants grown at control temperature, the FAX1 protein appeared increased by about 50% when compared with Wt plants (Fig. 1E). After 7 d in cold conditions, the FAX1 protein in Wt plants decreased markedly (Fig. 1E), which confirms our previous observations (Trentmann et al. 2020). Such cold-induced FAX1 degradation was almost absent in rbl11 mutants compared with Wt controls, while cold-induced FAX1 degradation occurred similarly in rbl10 and ftsh11 mutants (Fig. 1E).
Rbl11 mutants exhibit impaired frost tolerance
The observation that rbl11 plants show an increased number of wilted leaves when grown at cold temperatures (Fig. 1, B to D) led us to test the freezing tolerance of this mutant. The ability of vascular plants to withstand freezing temperatures depends on a pretreatment of several days at temperatures above 0 °C, called cold acclimation (Alberdi and Corcuera 1991). To investigate whether the altered cold response in mutants affects freezing tolerance, we compared the ability of Wt and rbl11 plants to recover from exposure to freezing temperatures. To this end, plants were grown at 21 °C and shifted to 4 °C for 4 d to acclimate the cold. The temperature was then gradually reduced (2 °C/h) until −10 °C was reached. This temperature was maintained for 15 h and then gradually increased (2 °C/h) to 21 °C before relighting (Trentmann et al. 2020). We assessed the appearance of frost-treated plants after 1 and 2 additional weeks of growth at 21 °C and quantified the number of wilted leaves as well as the survival rate after 2 wks of recovery.
As expected, unacclimated Wt and rbl11 plants are unable to survive a freezing treatment (Fig. 2A). As seen previously (Trentmann et al. 2020), Wt plants are able to recover from a period of freezing with an efficiency of about 80% (Fig. 2, A and B) and exhibited 42% wilted leaves (Fig. 2C). In contrast, rbl11 mutants showed a significantly reduced ability to recover from freezing treatment, as only 28% of all plants survived the freezing period and more than 60% of the leaves were wilted (Fig. 2, A to C).

Recovery from freezing is impaired in rbl11 loss-of-function mutants. Plants were grown under standard growth conditions for 3 wks. Prior to freezing, the temperature was lowered to 4 °C for 4 d (day and night temperature) for cold acclimation. The lowering of the temperature for freezing was done stepwise (2 °C/h) and in complete darkness. The temperature for freezing was maintained at −10 °C for 15 h before being gradually increased to 21 °C (2 °C/h). A) Representative Wt and rbl11 plants recovered from −10 °C freezing. Images were taken 7 and 14 d after freezing, from unacclimated and unfrozen (control) plants. B) Comparison of survival between Wt and rbl11 mutants 7 d after −10 °C treatment. Data represent the mean of 4 independent experiments with 10 to 20 plants per line and experiment. C) Quantification of wilted leaves from Wt and rbl11 plants recovered from −10 °C freezing for 7 d under standard growth conditions. Data are the mean of 4 independent experiments. Statistical differences between Wt and overexpressor lines in B) and C) were analyzed by Student's t test followed by Welch's correction: *P-value ≤ 0.05; **P-value ≤ 0.01 (Supplementary Data Set 3).
RBL11 and FAX1 interact physically at the inner envelope membrane of chloroplasts
Having established that RBL11 presence is mandatory for cold-induced FAX1 degradation (Fig. 1E), we were next interested to probe for a direct physical interaction between these 2 proteins. To this end, we exploited the bimolecular fluorescence complementation (BiFC) analysis (Kerppola 2008) based on the association of complementary yellow fluorescent protein (YFP) fragments fused to putative partner proteins. Only when both partners are in close proximity, a functional fluorescent protein can be formed, indicating the protein–protein interaction and its subcellular location within the living cell.
We infiltrated Nicotiana benthamiana leaves with the constructs FAX1:YFPC-n and RBL11:YFPC-c for synthesis of the respective YFP fragment proteins. Coexpression of both constructs resulted in yellow fluorescing spots in epidermis cells (Fig. 3A), indicating that both proteins interact in corresponding plastids. To further visualize the complex formation of both proteins at the chloroplast envelope, we infiltrated N. benthamiana leaves with both constructs and subsequently isolated mesophyll protoplasts. The combined expression of FAX1:YFPC-n and RBL11:YFPC-c led to a yellow fluorescence of chloroplasts (Fig. 3B). The ring-shaped fluorescence (Fig. 3B) resembles the YFP fluorescence emitted by other inner envelope–associated YFP fusion proteins (Witz et al. 2014; Patzke et al. 2019). To validate the BiFC data, we performed further control experiments using inner envelope–associated protease FTSH11. In contrast to the coexpression of FAX1:YFPC-n and RBL11:YFPC-c, the infiltration of the plasmids with FAX1:YFPC-n and FTSH11:YFPC-c did not yield fluorescence complementation (Fig. 3, C and D). In addition, the combined infiltration of the constructs FTSH11:YFPC-n and RBL11:YFPC-c also did not give rise to a fluorescence signal (Fig. 3, E and F). These 2 independent control experiments indicate that protein–protein interaction at the inner envelope does not occur by chance, highlighting the specificity of the RBL11 and FAX1 interaction (Fig. 3, A and B).
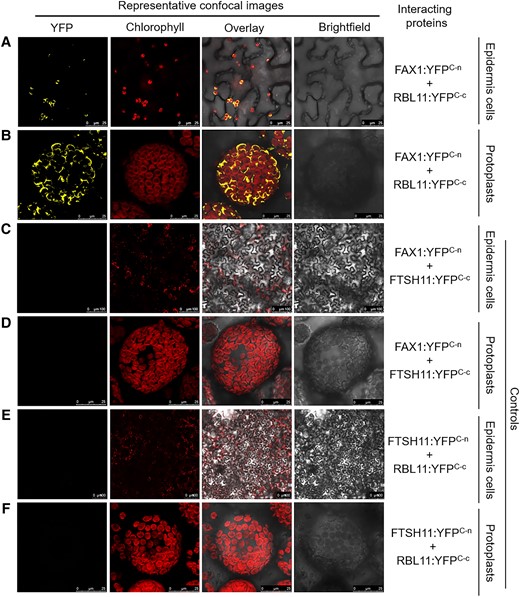
Probing of putative RBL11 targets. A to F) Constructs of full-length coding sequences were fused upstream to the C-terminus of YFP and transiently expressed in N. benthamiana leaves by agroinfiltration. YFP and chlorophyll fluorescence signals were recorded 4 d after infiltration in epidermis cells as well as in isolated protoplasts by confocal microscopy. Please note the different scale bar size between panels.
Induction of RBL11 leads to a specific degradation of FAX1
The FAX1 protein level remains elevated in rbl11 plants after onset of cold, compared with Wt (Fig. 1E). To further test our hypothesis that RBL11 is responsible for cold-induced FAX1 degradation, we generated a stable RBL11-HA (HA = hemagglutinin) overexpressor line in which the recombinant rbl11 gene was placed under the control of a dexamethasone (DEX)-inducible promoter (Aoyama and Chua 1997) (Fig. 4A). In the presence of DEX, the RBL11-HA protein is synthesized in mutant leaf discs at 21 °C and at 4 °C (Fig. 4, B and C), and neither DEX-induced overexpression nor the C-terminal HA tag alters the membrane localization of RBL11 (Fig. 4, B and C). Subsequent enrichment of total leaf membranes, followed by immunoblot analysis using the FAX1-specific antibody, showed that RBL11 overexpression does not lead to FAX1 degradation from leaf discs incubated with DEX at 21 °C (Fig. 4D). In contrast, DEX incubation at 4 °C leads to FAX1 degradation (Fig. 4D); note that extraction of total membrane proteins from whole membranes enriched from cold-treated leaf discs generally resulted in a higher abundance of FAX1 (Fig. 4D, left and right panels).
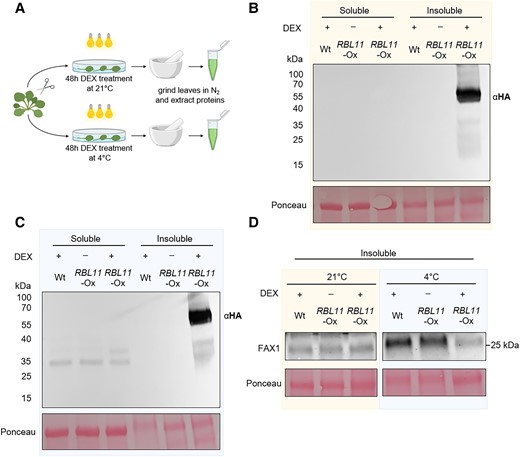
Cold-induced degradation of FAX1 by RBL11 in Arabidopsis. A) Scheme of experimental setup. B) Immunoblot analysis via HA antibody of soluble and insoluble (membrane) protein extracts from Wt and transgenic plants expressing RBL11 tagged with a HA epitope (∼32 kDa) under the control of a 35S promoter after induction with DEX for 48 h at 21 °C. C) Immunoblot detection via HA antibody of soluble and insoluble (membrane) protein extracts from Wt and transgenic plants expressing RBL11 tagged with a HA epitope (∼67 kDa) under the control of a 35S promoter after induction with DEX for 48 h at 4 °C. Note that the size of the protein (∼67 kDa) comes from the presence of an additional biotinylase (∼35 kDa), which was not relevant in this experiment. D) Immunoblot of insoluble protein extracts from Wt and transgenic plants overexpressing RBL11 under the control of a 35S promoter after induction with DEX for 48 h at 21 °C or 4 °C with a FAX1 antibody. Please note that the extraction of whole membranes enriched from cold-treated leaves generally resulted in a higher abundance of FAX1 and is not comparable with results from leaves treated at 21 °C. Ponceau staining in B), C), and D) is representing equal loading of protein samples with 18 µg per lane.
The membrane lipid composition of rbl11 mutants indicates a shift to the eukaryotic biosynthesis pathway under low temperature
The abundance of FAX1 strongly decreases after transfer of the Wt to cold conditions (Fig. 1E and Trentmann et al. (2020)). Because the absence of RBL11 prevents cold-induced FAX1 degradation (Fig. 1E), we aimed to determine changes in lipid levels under these growth conditions. For this purpose, Wt and rbl11 mutant plants were first grown for 4 wks under control conditions and then for 14 more days at 21 °C or 4 °C, prior to extraction and quantification of leaf lipids.
Growth of plants at 21 °C led to lower leaf levels of the major glycolipids MGDG and DGDG in rbl11 plants compared with the Wt (Fig. 5). In contrast, the levels of SQDG and the phospholipids phosphatidic acid (PA), phosphatidylserine (PS), phosphatidylinositol (PI), PG, PE, and PC were similar in leaves from the 2 plant lines (Fig. 5). These absolute amounts summed up to 230 nmol/mg dry weight (DW) of phospho- and glycolipids in rbl11 mutants and 274 nmol/mg DW of phospho- and glycolipids in Wt plants (Fig. 5A). However, after recalculating the data from total levels into mol%, no obvious changes in the relative individual lipid species between rbl11 mutant and Wt plants were found (Fig. 5B). Interestingly, growth for 14 d under cold temperature conditions led to higher total lipid levels in the rbl11 plants (Fig. 5C). This increase of total lipids in the rbl11 mutants is due to higher levels of MGDG, DGDG, and SQDG but also to higher levels of all phospholipids (Fig. 5C). Furthermore, after recalculating the data into mol%, it appeared that growth at 4 °C did not only lead to higher total lipid contents in rbl11 plants but that especially the relative proportions (in mol%) of the phospholipid species PA, PI, PE, and PC to the total lipids were increased in the rbl11 plants, while proportions of MGDG and DGDG are decreased (Fig. 5D).
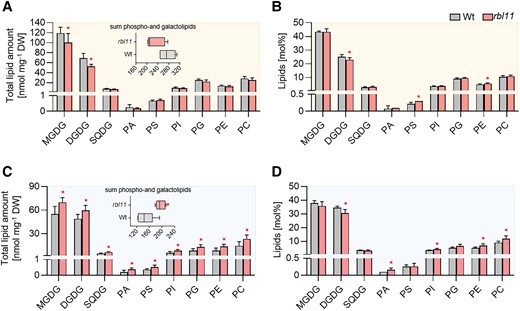
Analysis of galacto- and phospholipids in Wt and rbl11 loss-of-function mutants. Changes in A) total contents and B) relative amounts of different galacto- and phospholipids in rosette leaves of 3-wk-old Arabidopsis plants grown under standard conditions. The box plot in A) shows changes in the sum of the measured total lipid contents. Differences in C) total lipid contents and D) relative galacto- and phospholipid amounts in plants grown under standard conditions for 3 wks before lowering the temperature to 4 °C for 14 d. The box plot in C) shows the changes in the sum of the measured total lipid contents. Data represent the mean of 5 plants per row. Error bars indicate ± Sd. Significance of differences between Wt and mutant was analyzed by Student's t test: *P-value ≤ 0.05 (Supplementary Data Set 3).
Identification of other putative RBL11 protease substrates
By analyzing the envelope proteome of rbl10 rbl11 double mutants, an overrepresentation of nondegraded envelope proteins compared with the Wt emerged (Knopf et al. 2012). However, this type of analysis did not allow for the identification of RBL11-specific substrates. Thus, we compared the envelope proteome from Wt plants with that of rbl11 single mutants (Table 1, Supplementary Tables S1, S2, and S3, Fig. S1, and Data Set 1). Since we were especially interested in RBL11 substrates degraded after onset of cold temperatures, these analyses were carried out on plants grown at 21 °C or at 4 °C (Table 1).
Fifteen top hits of proteins that are increased by more than 30% in the mutant at low temperature
Protein . | Gene locus . | Description . | Log2FC rbl11/Wt . | qValue_interaction genotype temperature . |
---|---|---|---|---|
TGD5 | At1g27695 | Encodes a small glycine-rich protein that is localized to the chloroplast envelope and is a component of the ER to plastid lipid trafficking pathway. | 4.54 | 0.033 |
PORA | At5g54190 | Light-dependent NADPH:PORA | 4.08 | 0.030 |
HCF107 | At3g17040 | Involved in regulating plastidial gene expression and biogenesis | 1.56 | 0.002 |
Unknown | At3g28220 | TRAF-like family protein, contains MATH domain | 2.73 | 0.044 |
KIN14A | At5g10470 | Kinesin-like protein required for chloroplast movements and anchors to the plasma membrane | 2.18 | 0.032 |
DVR | At5g18660 | Encodes a protein with 3,8-divinyl protochlorophyllide a 8-vinyl reductase activity | 1.85 | 0.031 |
Unknown | At1g08530 | Chitinase-like protein | 1.35 | 0.019 |
Unknown | At5g19850 | Alpha/beta-hydrolases superfamily protein | 1.47 | 0.027 |
Unknown | At1g64850 | Calcium-binding EF hand family protein | 1.22 | 0.015 |
Unknown | At5g39410 | Saccharopine dehydrogenase | 1.06 | 0.018 |
Unknown | At2g43630 | Nucleus envelope protein | 0.48 | 0.004 |
TGD2 | At3g20320 | Encodes a permease-like component of an ABC transporter involved in lipid transfer from the ER to chloroplast. A PA-binding protein. | 0.98 | 0.022 |
DEGP2 | At2g47940 | Encodes DegP2 protease | 0.83 | 0.015 |
SPPA | At1g73990 | Encodes a light-inducible chloroplast protease complex | 0.78 | 0.015 |
FAX1* | At3g57280 | Involved in FA and lipid homeostasis and likely functions as a FA transporter that exports FAs from the plastid | 0.38 | 0.028 |
Protein . | Gene locus . | Description . | Log2FC rbl11/Wt . | qValue_interaction genotype temperature . |
---|---|---|---|---|
TGD5 | At1g27695 | Encodes a small glycine-rich protein that is localized to the chloroplast envelope and is a component of the ER to plastid lipid trafficking pathway. | 4.54 | 0.033 |
PORA | At5g54190 | Light-dependent NADPH:PORA | 4.08 | 0.030 |
HCF107 | At3g17040 | Involved in regulating plastidial gene expression and biogenesis | 1.56 | 0.002 |
Unknown | At3g28220 | TRAF-like family protein, contains MATH domain | 2.73 | 0.044 |
KIN14A | At5g10470 | Kinesin-like protein required for chloroplast movements and anchors to the plasma membrane | 2.18 | 0.032 |
DVR | At5g18660 | Encodes a protein with 3,8-divinyl protochlorophyllide a 8-vinyl reductase activity | 1.85 | 0.031 |
Unknown | At1g08530 | Chitinase-like protein | 1.35 | 0.019 |
Unknown | At5g19850 | Alpha/beta-hydrolases superfamily protein | 1.47 | 0.027 |
Unknown | At1g64850 | Calcium-binding EF hand family protein | 1.22 | 0.015 |
Unknown | At5g39410 | Saccharopine dehydrogenase | 1.06 | 0.018 |
Unknown | At2g43630 | Nucleus envelope protein | 0.48 | 0.004 |
TGD2 | At3g20320 | Encodes a permease-like component of an ABC transporter involved in lipid transfer from the ER to chloroplast. A PA-binding protein. | 0.98 | 0.022 |
DEGP2 | At2g47940 | Encodes DegP2 protease | 0.83 | 0.015 |
SPPA | At1g73990 | Encodes a light-inducible chloroplast protease complex | 0.78 | 0.015 |
FAX1* | At3g57280 | Involved in FA and lipid homeostasis and likely functions as a FA transporter that exports FAs from the plastid | 0.38 | 0.028 |
For each protein, a 2-way ANOVA was performed, which means that only proteins with a significance level below 0.05 were considered. The qValue_Interaction Genotype Temperature indicates that there is a temperature effect that differs between mutant and Wt. All proteins were annotated by name, gene locus, and description. The proteins written in bold are increased under standard conditions as well as after 7 d at 4 °C (see Supplementary Table S1) to the described selected criteria (abundance > 30%; q-value < 0.05). FAX1 was labeled with an asterisk because the protein is not within the top hits but also fulfills the selected criteria (Supplementary Fig. S1 and Supplementary Data Set 1).
Fifteen top hits of proteins that are increased by more than 30% in the mutant at low temperature
Protein . | Gene locus . | Description . | Log2FC rbl11/Wt . | qValue_interaction genotype temperature . |
---|---|---|---|---|
TGD5 | At1g27695 | Encodes a small glycine-rich protein that is localized to the chloroplast envelope and is a component of the ER to plastid lipid trafficking pathway. | 4.54 | 0.033 |
PORA | At5g54190 | Light-dependent NADPH:PORA | 4.08 | 0.030 |
HCF107 | At3g17040 | Involved in regulating plastidial gene expression and biogenesis | 1.56 | 0.002 |
Unknown | At3g28220 | TRAF-like family protein, contains MATH domain | 2.73 | 0.044 |
KIN14A | At5g10470 | Kinesin-like protein required for chloroplast movements and anchors to the plasma membrane | 2.18 | 0.032 |
DVR | At5g18660 | Encodes a protein with 3,8-divinyl protochlorophyllide a 8-vinyl reductase activity | 1.85 | 0.031 |
Unknown | At1g08530 | Chitinase-like protein | 1.35 | 0.019 |
Unknown | At5g19850 | Alpha/beta-hydrolases superfamily protein | 1.47 | 0.027 |
Unknown | At1g64850 | Calcium-binding EF hand family protein | 1.22 | 0.015 |
Unknown | At5g39410 | Saccharopine dehydrogenase | 1.06 | 0.018 |
Unknown | At2g43630 | Nucleus envelope protein | 0.48 | 0.004 |
TGD2 | At3g20320 | Encodes a permease-like component of an ABC transporter involved in lipid transfer from the ER to chloroplast. A PA-binding protein. | 0.98 | 0.022 |
DEGP2 | At2g47940 | Encodes DegP2 protease | 0.83 | 0.015 |
SPPA | At1g73990 | Encodes a light-inducible chloroplast protease complex | 0.78 | 0.015 |
FAX1* | At3g57280 | Involved in FA and lipid homeostasis and likely functions as a FA transporter that exports FAs from the plastid | 0.38 | 0.028 |
Protein . | Gene locus . | Description . | Log2FC rbl11/Wt . | qValue_interaction genotype temperature . |
---|---|---|---|---|
TGD5 | At1g27695 | Encodes a small glycine-rich protein that is localized to the chloroplast envelope and is a component of the ER to plastid lipid trafficking pathway. | 4.54 | 0.033 |
PORA | At5g54190 | Light-dependent NADPH:PORA | 4.08 | 0.030 |
HCF107 | At3g17040 | Involved in regulating plastidial gene expression and biogenesis | 1.56 | 0.002 |
Unknown | At3g28220 | TRAF-like family protein, contains MATH domain | 2.73 | 0.044 |
KIN14A | At5g10470 | Kinesin-like protein required for chloroplast movements and anchors to the plasma membrane | 2.18 | 0.032 |
DVR | At5g18660 | Encodes a protein with 3,8-divinyl protochlorophyllide a 8-vinyl reductase activity | 1.85 | 0.031 |
Unknown | At1g08530 | Chitinase-like protein | 1.35 | 0.019 |
Unknown | At5g19850 | Alpha/beta-hydrolases superfamily protein | 1.47 | 0.027 |
Unknown | At1g64850 | Calcium-binding EF hand family protein | 1.22 | 0.015 |
Unknown | At5g39410 | Saccharopine dehydrogenase | 1.06 | 0.018 |
Unknown | At2g43630 | Nucleus envelope protein | 0.48 | 0.004 |
TGD2 | At3g20320 | Encodes a permease-like component of an ABC transporter involved in lipid transfer from the ER to chloroplast. A PA-binding protein. | 0.98 | 0.022 |
DEGP2 | At2g47940 | Encodes DegP2 protease | 0.83 | 0.015 |
SPPA | At1g73990 | Encodes a light-inducible chloroplast protease complex | 0.78 | 0.015 |
FAX1* | At3g57280 | Involved in FA and lipid homeostasis and likely functions as a FA transporter that exports FAs from the plastid | 0.38 | 0.028 |
For each protein, a 2-way ANOVA was performed, which means that only proteins with a significance level below 0.05 were considered. The qValue_Interaction Genotype Temperature indicates that there is a temperature effect that differs between mutant and Wt. All proteins were annotated by name, gene locus, and description. The proteins written in bold are increased under standard conditions as well as after 7 d at 4 °C (see Supplementary Table S1) to the described selected criteria (abundance > 30%; q-value < 0.05). FAX1 was labeled with an asterisk because the protein is not within the top hits but also fulfills the selected criteria (Supplementary Fig. S1 and Supplementary Data Set 1).
Putative substrates of RBL11 are expected to be more abundant in rbl11 mutants than in Wt plants. In a previous study on proteins accumulating in the inner envelope of rbl10 rbl11 double mutants, the TRAF-like family protein containing a MATH domain was also found to accumulate in the double mutant (Knopf et al. 2012). Similarly, the TRAF-like family protein accumulated in rbl11 single mutants (Supplementary Table S1), indicating that this protease is responsible for its degradation. Interestingly, the abundance of the protein TRIGALACTOSYLDIACYLGLYCEROL 5 (TGD5), which is involved in ER to plastid lipid transfer (Fan et al. 2015), is also higher in rbl11 mutants than in the Wt, and this effect is independent of the environmental temperature (Supplementary Table S1 and Table 1). Besides TGD5, the abundance of another TGD protein, namely TRIGALACTOSYLDIACYLGLYCEROL 2 (TGD2), is also higher in rbl11 mutants when exposed for 4 d to 4 °C (Table 1).
In addition, the chloroplast-located protease systems DEGRADATION OF PERIPLASMIC PROTEINS 2 (DEGP2) and SIGNAL PEPTIDE PEPTIDASE (SPPA) are also more abundant in cold-treated rbl11 mutants when compared with the Wt (Table 1). Similarly, the inner envelope–associated PROTOCHLOROPHYLLIDE OXIDOREDUCTASE A (PORA) (Barthélemy et al. 2000) appears, besides the TGD components and FAX1, as a further RBL11 substrate (Supplementary Table S1), especially after onset of cold temperature (Table 1). It seems likely that the decrease of some proteins in rbl11 chloroplasts under warm or cold conditions represents pleiotropic reactions of the mutant induced by altered FAX1 abundance (Supplementary Tables S2 and S3 and Fig. S1). Nevertheless, among these proteins, we also found proteins associated with lipid metabolism, e.g. LONG CHAIN ACYL-COA SYNTHETASE 9 (LACS9), representing a major long chain acyl-CoA synthetase (Schnurr et al. 2002), or BILE ACID:SODIUM SYMPORTER 1 (BASS1), representing a sodium/pyruvate cotransporter (Furumoto et al. 2011) (Supplementary Tables S2 and S3).
FAX1 overexpressor lines exhibit markedly increased levels of FAX1 protein
The above data indicate a molecular relationship between increased FAX1 protein abundance (due to an absence of RBL11) and the levels of membrane lipids and Arabidopsis cold tolerance (Figs. 1 and 3). This encouraged us to analyze the impact of increased FAX1 abundance on plant cold tolerance. rbl11 mutants are not conducive to this, because the absence of this protease does not exclusively affect FAX1 levels but also the abundance of other envelope-associated proteins (Table 1 and Supplementary Fig. S1). To avoid the study of pleiotropic effects, we decided to investigate the cold tolerance of Arabidopsis mutants with constitutively increased FAX1 levels (Li, Gügel, et al. 2015; Li, Zheng, et al. 2015).
Our observations that rbl11 loss-of-function mutants have increased FAX1 levels under cold conditions (Fig. 1E) and that directed synthesis of RBL11 leads to a decrease in FAX1 in the cold (Fig. 2) provide evidence that the RBL11 protease is involved in this process. Nevertheless, for a comprehensive understanding of FAX1's impact on the cold response, it is mandatory to study FAX1 transcript levels after transfer to cold conditions. In fact, the observation that FAX1 mRNA rises markedly in Wt plants after exposure to 4 °C (Fig. 6A) supports our conclusion that RBL11 is responsible for the cold-induced decrease of the level of the FAX1 protein (Fig. 1F).
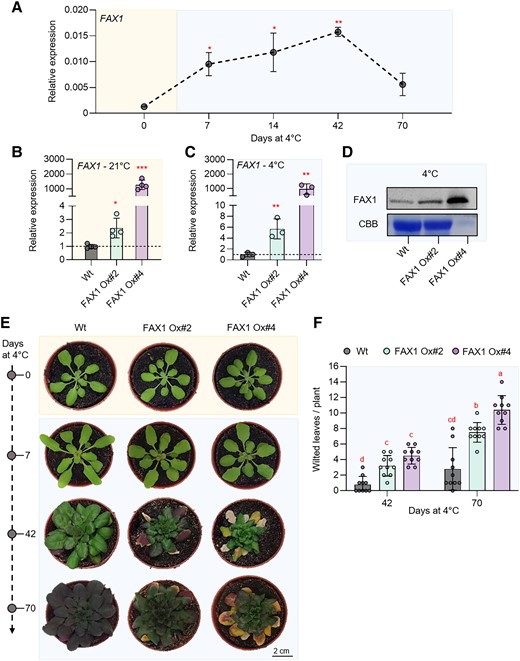
Gene expression, immunoblot, and phenotypic analysis of 2 independent Arabidopsis FAX1 overexpression lines (FAX1 Ox#2 and FAX1 Ox#4) and Wt plants grown under standard and cold (4 °C) conditions. Plants were grown under standard conditions (21 °C day and night, 10 h day length, and 120 µE light intensity) for 3 wks and then treated with cold (4 °C day and night, 10 h day length, and 120 µE light intensity). A) Expression of FAX1 by qRT-PCR under standard growth conditions (0 d at 4 °C) and several days during cold treatment (7, 14, 42, and 70 d at 4 °C). Data represent relative mean expression levels of 3 biological replicates and are normalized to standard conditions (0 d at 4 °C) using UBQ as an internal control. Relative expression of FAX1 by qRT-PCR under B) standard growth conditions and C) 7 d after cold treatment. Data represent relative mean expression levels and are normalized to the Wt using UBQ as an internal control. D) Immunoblot analysis of FAX1 in crude extract from plants grown at 4 °C for 7 d. The image of the Coomassie stained gel (CBB) shows equal loading of protein samples from Wt and FAX1 Ox#2 with 30 µg per lane. The amount of FAX1 Ox#4 protein is reduced to 3 µg. E) Rosette phenotype of representative Wt, FAX1 Ox#2, and FAX1 Ox#4 under standard growth conditions (0 d at 4 °C) and after cold treatment (7, 42, and 70 d at 4 °C). F) Number of wilted leaves per plant after 42 and 70 d of cold treatment. Error bars in A) represent ± Sem. Error bars in B), C), and F) are ± Sd. Statistical differences between Wt and overexpressor lines in A), B), and C) were analyzed by Student's t test: *P-value ≤ 0.05; **P-value ≤ 0.01; ***P-value ≤ 0.001. Letters above error bars in F) indicate significant differences by 2-way ANOVA followed by Tukey’s test (P < 0.05) (Supplementary Data Set 3).
Previously, we generated 2 independent FAX1-overexpressing lines, FAX1 Ox#2 and FAX1 Ox#4, both containing the FAX1 gene under the control of the constitutive cauliflower mosaic virus 35S promoter (Li, Gügel, et al. 2015; Li, Zheng, et al. 2015). So far, it has only been shown that these mutant lines have both increased FAX1 mRNA and increased FAX1 protein levels when grown at ambient temperature (Li, Gügel, et al. 2015; Li, Zheng, et al. 2015). We verified that the FAX1 mRNA accumulation is a stable feature when FAX1 overexpressor mutants are cultivated at cold temperature. For this purpose, the FAX1 mRNA levels were quantified in plants grown under the standard temperature of 21 °C with those grown for 1 wk at 4 °C. The 2 FAX1 overexpressor lines exhibited a substantial accumulation of FAX1 mRNA when compared with Wt plants. Under warm temperature, FAX1 Ox#2 plants contained about 2.1-fold higher FAX1 mRNA levels compared with the Wt, while FAX1 Ox#4 plants contained 1,170-fold higher FAX1 mRNA levels (Fig. 6B). This marked difference of FAX1 mRNA in the 2 overexpressors concurs with previous observations (Li, Gügel, et al. 2015; Li, Zheng, et al. 2015). After 1 wk of growth at 4 °C, FAX1 Ox#2 plants contained about 5.7-fold higher FAX1 mRNA levels, and FAX1 Ox#4 plants contained more than 970-fold higher FAX1 mRNA levels than present in the Wt (Fig. 6C). Thus, even at 4 °C, both FAX1 overexpressor lines exhibited higher FAX1 mRNA than present in the Wt.
A previous study showed that both FAX1 overexpressors contain higher levels of FAX1 protein when grown at 21 °C (Li, Gügel, et al. 2015; Li, Zheng, et al. 2015). To confirm that this is also true under cold conditions, we conducted an immunoblot analysis (Li, Gügel, et al. 2015; Li, Zheng, et al. 2015). After 1 wk of growth at 4 °C, the relative FAX1 protein level in both overexpressor plants was higher than observed in Wt plants (Fig. 6D), and a corresponding quantification experiment revealed that Ox#2 plants contained about 3-fold more FAX1 protein than present in the Wt, while Ox#4 plants contained about 115-fold more FAX1 protein (Fig. 6D; please note: loaded protein extracted from Ox#4 plants was 1:10 diluted when compared with proteins extracted from Wt and Ox#2 mutants).
Similar to rbl11 mutants, FAX1 overexpressor plants exhibit decreased chilling and frost tolerance
The data above indicate that rbl11 mutants exhibit increased cold sensitivity (Fig. 1, B and C). Because rbl11 mutants had higher FAX1 levels than present in the Wt, it was interesting to analyze whether the 2 lines constitutively expressing the FAX1 gene also show altered responses to low temperatures.
Therefore, we cultivated all plants at ambient temperature (21 °C) prior to cultivation at 4 °C. Subsequently, we inspected plant appearance and the number of wilted leaves in the respective growth phase. After 7 d at 4 °C, none of the 3 plant lines developed wilted leaves (Fig. 6E). However, after 42 d at 4 °C, and even more pronounced after 70 d at 4 °C, leaves from both FAX1 overexpressors gradually wilted more rapidly than observed in the Wt (Fig. 6E).
To quantify this observation, we counted the wilted leaves as a proxy for chilling sensitivity (Trentmann et al. 2020). After 42 d at 4 °C, Wt plants exhibited on average 1 wilted leaf, while FAX1 overexpressor Ox#2 exhibited in average 3 wilted leaves, and Ox#4 mutants showed on average 4.5 wilted leaves (Fig. 6F). After growth for 10 wks at 4 °C, Wt plants displayed on average 2.8 wilted leaves per plant, while FAX1 overexpressors Ox#2 and Ox#4 exhibited on average 7.5 and 10.4 wilted leaves, respectively (Fig. 6F).
As done for rbl11 plants (Fig. 2), we compared the frost tolerance of FAX1 overexpressor plants and Wt. In contrast to both FAX1 overexpressors, Wt plants that survived the postfreezing phase restored efficient growth within the next 14 d, as indicated by a larger rosette size and less wilted leaves when compared with overexpressor mutants (Fig. 7A). FAX1 overexpressor plants able to recover from freezing exhibited about 80% of wilted leaves, while Wt only exhibited about 46% of wilted leaves (Fig. 7, A and B). With about 87%, the survival rate of Wt plants reached a value similar to previous observations (Trentmann et al. 2020). In contrast, only 57% of the FAX1 Ox#2 plants survived this stress, and only 43% of FAX1 Ox#4 plants recovered from frost (Fig. 7C).
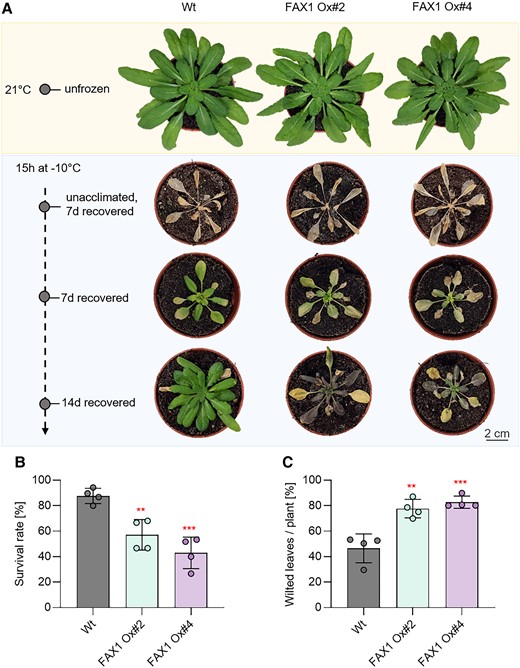
Recovery from frost is impaired in FAX1 overexpression mutants. Plants were cultivated for 3 wks under standard growing conditions. Before freezing, the temperature was lowered to 4 °C for 4 d (day and night temperature) for cold acclimation. Lowering of the temperature for freezing was done stepwise (2 °C/h) and in completely dark. For 15 h, −10 °C was kept before a stepwise temperature raising to 21 °C (2 °C/h). A) Representative Wt and FAX1 overexpression plants recovered from −10 °C freezing. Pictures were taken 7 and 14 d after freezing, from unacclimated and unfrozen (control) plants. B) Comparison of survival rate between Wt and FAX1 overexpression mutants 7 d after −10 °C treatment. Data represent the mean value from 4 independent experiments with 11 to 15 plants per line and experiment. C) Quantification of wilted leaves from Wt and FAX1 overexpressor plants recovered for 7 d from −10 °C freezing under standard growing conditions. Data represent the mean value of 4 independent experiments. Statistical differences between Wt and the overexpressor lines in B) and C) were analyzed by Student's t test: **P-value ≤ 0.01; ***P-value ≤ 0.001 (Supplementary Data Set 3).
Similar to rbl11 mutants, lipid biosynthesis in FAX1 overexpressors is shifted to the eukaryotic biosynthesis pathway under low temperature
Under cold conditions, rbl11 mutants exhibited a shift toward ER-synthesized membrane lipids when compared with their corresponding Wt (Fig. 5). To check whether this response is due to high FAX1 protein abundance, we analyzed the lipid composition in FAX1 overexpressors. For a first overview of cold effects on membrane lipid homeostasis in FAX1 overexpressor plants, we grew Wt and the representative FAX1 Ox#4 line (Li, Gügel, et al. 2015; Li, Zheng, et al. 2015) under either control conditions or for 2 wks at 4 °C. Subsequently, leaf lipids were extracted and quantified via MS.
When grown at 21 °C, the total levels (nmol/mg DW) and the relative proportions (mol%) of the glycolipids MGDG, DGDG, and SQDG or of the phospholipids PA, PS, PI, PG, PE, and PC were similar in leaves from Wt and FAX1 Ox#4 plants (Supplementary Fig. S2, A and B). In contrast, after 2 wks at 4 °C, FAX1 overexpressor plants contained higher levels of MGDG and DGDG when compared with Wt (Supplementary Fig. S2C). In addition, the levels of PS, PG, PE, and PC FAX1 Ox#4 plants appeared to be slightly higher than in corresponding Wt (Supplementary Fig. S2C). This increase led in sum to 158 nmol/mg DW of membrane lipids in FAX1 Ox#4 plants, while Wt contained only 125 nmol/mg DW membrane lipids (Supplementary Fig. S1C). Similar to the situation at 21 °C, the proportions of all lipid species in both plant lines are nearly identical (Supplementary Fig. S2D).
Since FAX1 overexpressor plants revealed impaired cold tolerance after long exposure to low temperatures and because the observed changes in lipid composition of FAX1 overexpressors after 2 wks of growth at cold temperature appeared in parts to be moderate (Supplementary Fig. S2), we extracted lipids from plants exposed for 10 wks to 4 °C (Fig. 8). Similar to plants exposed to only 2 wks at 4 °C (Supplementary Fig. S2), both FAX1 overexpressor lines exhibited increased total levels of the galactolipids MGDG and DGDG and also of the phospholipids PI, PG, PE, and PC (Fig. 8A). The increase of the 2 groups of lipids led to an overall higher level of total membrane lipids in FAX1 overexpressor lines (FAX1 Ox#2, 205 nmol/mg DW; FAX1 Ox#4, 188 nmol/mg DW; Wt, 125 nmol/mg DW) (Fig. 8A). The levels of the low abundant glycolipid SQDG and the phospholipid PS were not altered in FAX1 overexpressors, when compared with the Wt (Fig. 8A). Although total amounts of glycolipids in both FAX1 overexpressors were higher when compared with Wt (Fig. 8A), the relative contribution (mol %) of MGDG, DGDG, and SQDG in FAX1 Ox#2 and #4 to total membrane lipids was similar to the Wt (Fig. 8B), while the relative contribution of the phospholipids PG, PE, and PC in FAX1 Ox#2 and #4 to total membrane lipids was increased, when compared with the Wt (Fig. 8B). The most pronounced alterations of phospholipids were noted for PC and PE (Fig. 8B), which were 40% higher in FAX1 overexpressors than in Wt plants (Fig. 8B).
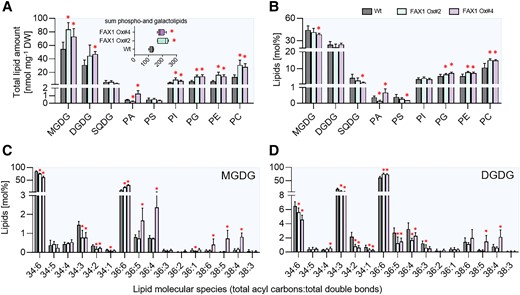
Lipid analysis of Wt and FAX1 overexpression lines. Galacto- and phospholipids as well as galactolipid molecular species were determined in leaves of plants grown for 3 wks under standard conditions before lowering the growth temperature to 4 °C for 10 wks. Changes in A) total contents and B) relative amounts of different galacto- and phospholipids. Boxplot in A) indicates changes in the sum of measured total lipid contents. Lipid composition of C) MGDG and D) DGDG molecular species. Data represent mean values of 5 plants per line. Error bars represent ± Sd. Significance of differences between Wt and mutant lines was analyzed by Student's t test: *P-value ≤ 0.05 (Supplementary Data Set 3).
MGDG and DGDG represent the 2 most abundant glycerolipids in Arabidopsis leaves (Fig. 8A). Interestingly, a closer inspection of the contents of the 2 MGDG molecular species 34:6 and 36:6, which are indicative for either plastid-generated MGDG (34:6) or ER-born MGDG (36:6), revealed clear differences between the Wt and overexpressors. Wild-type plants exhibited about 85 mol% of 34:6 MGDG, while leaves from FAX1 Ox#2 and Ox#4 plants accumulated only 75 and 62 mol% of this MGDG species, respectively (Fig. 8C). In marked contrast to this, the Wt contained only 10 mol% of the MGDG molecular species 36:6, while the FAX1 overexpressor plants Ox#2 and Ox#4 accumulated 21 and 30 mol% of 36:6 MGDG, respectively (Fig. 8C). The relative levels of 34:6 and 34:3 DGDG in Wt leaves were higher than in correspondingly grown FAX1 Ox#2 and Ox#4 plants. 34:6 DGDG in the Wt amounted at 6.5 mol%, while FAX1 Ox#2 and Ox#4 plants contained only 5.6 or 4.6 mol%, respectively (Fig. 8D). Eukaryotic ER-produced 36:6 DGDG in Wt amounted to a relative abundance of 61 mol%, while, similar to the increase of 36:6 MGDG, the 2 FAX1 overexpressors contained 75 and 74 mol% of 36:6 type DGDG, respectively (Fig. 8D). In summary, we can conclude that FAX1 overexpressor lines at cold temperatures accumulate ER-produced phospholipids—namely PC and PE—as well as galactolipids (MGDG, DGDG) with DAG backbones from the eukaryotic pathway, while the proportions of prokaryotic 34:x galactolipids are reduced.
FAX1 overexpressor lines show symptoms of impaired photosynthesis at early time points of cold exposure
One marked phenotype of FAX1 overexpressor plants after transfer to cold conditions is the appearance of wilted, decayed leaves after some weeks of growth (Fig. 6, F and H). However, degradation of the FAX1 protein and first changes of the lipid composition are already observed after a few d at 4 °C (Fig. 1F and Supplementary Fig. S1). To search for additional responses, we quantified photosynthetic parameters after short and longer exposure to 4 °C. To this end, we grew all plants for 28 d at 21 °C prior to transfer to at 4 °C. At the beginning of the transfer, and after 1 or 6 wks of growth at 4 °C, we quantified the photosynthetic performance by measuring the following parameters: the ratio of variable to maximal fluorescence (Fv/Fm), PSII efficiency Y(II), nonphotochemical quenching Y(NPQ), nonregulated quenching Y(NO), and the rate of electron transport (ETR). These comprehensive analyses have been done using the pulse amplitude modulation (PAM) fluorometry method (Schreiber 2004).
Prior to transfer to the cold, all Wt and the 2 FAX1 overexpressor plants exhibited similar Fv/Fm ratios (Fig. 9A) which ranged around 0.79 (Fig. 9B). After onset of cold temperatures, the Fv/Fm ratio decreased gradually in all 3 lines. Already after 1 wk, the Fv/Fm ratio in both FAX1 overexpressor lines was significantly lower than displayed by the Wt, and after 6 wks in the cold, Wt plants showed a Fv/Fm ratio of 0.73, while both mutants exhibited a Fv/Fm ratio of about 0.68 (Fig. 9, A and B). This altered Fv/Fm ratio in the cold is not reflected by an increased NPQ, although FAX1 Ox#2 plants showed a slightly increased NPQ, after 7 d in the cold. However, this was not found for FAX1 Ox#4 plants (Fig. 9) and, even after 5 wks in the cold, NPQ in all 3 lines was similarly decreased (Fig. 9C). The photosynthetic quantum yield Y(II) of all 3 plant lines was similar when grown under control temperature (Fig. 9C). In contrast, both FAX1 overexpressors showed after 7 or 42 d in the cold a decreased Y(II) and an increased energy dissipation via nonregulated quenching Y(NO), which was especially pronounced after 42 d (Fig. 9C). While the chloroplastic ETR of all 3 plant lines was similar at the beginning of the cold treatment, the ETR in both FAX1 overexpressor plants was after 7 d at 4 °C markedly lower, when compared with corresponding Wt plants (Fig. 9C).
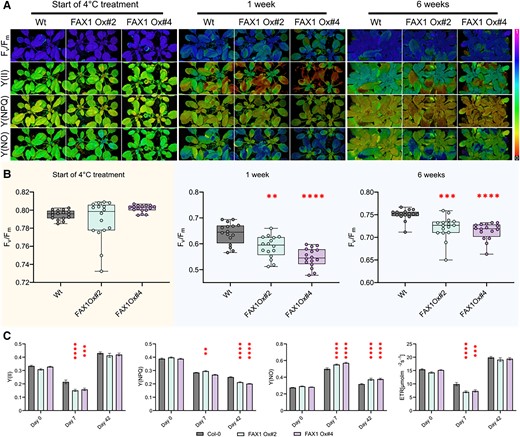
Cold-dependent PSII alterations in FAX1 overexpressor lines. After 28 d of growth under short-day (10/14 h; 110 PAR) conditions at RT, plants (Col-0; FAX1 Oex#2; FAX1 Oex#4) were shifted to a 4 °C short-day (110 PAR) chamber. PAM induction curve measurements at 110 PAR were performed on Day 0 as well as after 7 and 42 d of the cold treatment. A) Representative PAM images are depicted for PSII capacity (Fv/Fm), yield (Y(II)), quantum yield of light-induced nonphotochemical fluorescence quenching (Y(NPQ)), and quantum yield of nonregulated energy dissipation (Y(NO)). B)Fv/Fm determination of the 3 genotypes at the start of the cold treatment, after 1 and 5 wks. C) Induction curves were generated until a steady-state phase of Y(II), Y(NPQ), Y(NO), and ETR (µmol/m2/s) was reached. The data shown represent this steady-state after 600 s. N = 16; mean; ± Sem; P-value: (1-way ANOVA): *, 0.033; **, 0.0021; ***, 0.0002; and ****, 0.0001 (Supplementary Data Set 3).
FAX1 overexpressor and rbl11 mutants exhibit signs of reactive oxygen species accumulation
Several systemic changes in FAX1 overexpressors and rbl11 mutants occur quite rapidly after transfer to cold conditions. For example, first, changes in the lipid composition of both types of mutants are already present after 2 wks at 4 °C (Fig. 5 and Supplementary Fig. S2) and alterations in photosynthetic parameters of FAX1 overexpressors are already present after 1 wk of growth at low temperature (Fig. 7). Given that both types of mutants contain higher FAX1 abundance than present in Wt (Figs. 1 and 4) and show similar shifts in lipid biosynthesis (Fig. 5 and Supplementary Fig. S2), we searched for further similarities in their molecular responses after transfer into cold conditions.
The accumulation of anthocyanins and reactive oxygen species (ROS) can be taken as early responses upon onset of abiotic stress stimuli (Chalker-Scott 1999; Baxter et al. 2014). When grown at 21 °C, rbl11 plants and the 2 FAX1 overexpressor lines contain similar levels of anthocyanins as Wt plants (Fig. 10A). In contrast, when exposed to 4 °C for only 1 wk, rbl11 mutants and FAX1 overexpressors accumulated significantly more anthocyanins than the correspondingly grown Wt (Fig. 10A). The levels of the transcript coding for the protein BON ASSOCIATION PROTEIN 1 (BAP1), which indicates the cold-induced occurrence of ROS (Yang et al. 2007; Zhu et al. 2011), were unchanged between the plant lines when grown at 21 °C (Fig. 10B). Similar to the anthocyanin accumulation, BAP1 mRNA accumulated to much higher extents in both types of mutants after 1 wk of growth at 4 °C than in Wt plants (Fig. 10B).
![Anthocyanin and ROS accumulation in rbl11 loss-of-function mutants and FAX1 overexpressors at low temperatures. A) Impact of cold on anthocyanin accumulation under ambient conditions and after 7 d at 4 °C. B) Relative transcript levels of BAP1 during standard conditions and after 7 d at 4 °C. C) NBT staining of O2- accumulation. Plants were grown for 2 wks under control conditions and subsequently cultivated at 4 °C for 4 d. Error bars in A) and B) represent ± Sd. Letters displayed over the error bars indicate significant differences analyzed by 2-way ANOVA followed by Tukey's multiple comparisons test (P-value < 0.05 [Supplementary Data Set 3]).](https://oup.silverchair-cdn.com/oup/backfile/Content_public/Journal/plcell/36/5/10.1093_plcell_koae011/1/m_koae011f10.jpeg?Expires=1750553618&Signature=HtVDUgcxLQhstFckJu0Dea-Xn9toVowItVRNq4UUcttaSbYvvdJT2e7ZUCWPp8~fNzZhqv9KGkKFW7YlZYNNRarKaPOnmVPkDWLOrXb19eVijIXsFQt1BvzrO9ZGworWAQuVPI6GeERF47CJfNOv1TU4hXo9LKoL9kA1Ba~2ss4Tx-GJc7eu~XMK1Qg0dlOF140UONy32Wchj7r2XlvpoKL1fgNnDOXddu9B3PQPWLBrOeMBgfUHMVOac0~MHTBsm6gQER8nzVfwC8Zo6KeOESxeYYhshY2GtBuM-IJIIZWo9cpowOirb9ZWXcdPNaxBUdPdH6kck-eNT3VEBQKqNQ__&Key-Pair-Id=APKAIE5G5CRDK6RD3PGA)
Anthocyanin and ROS accumulation in rbl11 loss-of-function mutants and FAX1 overexpressors at low temperatures. A) Impact of cold on anthocyanin accumulation under ambient conditions and after 7 d at 4 °C. B) Relative transcript levels of BAP1 during standard conditions and after 7 d at 4 °C. C) NBT staining of O2- accumulation. Plants were grown for 2 wks under control conditions and subsequently cultivated at 4 °C for 4 d. Error bars in A) and B) represent ± Sd. Letters displayed over the error bars indicate significant differences analyzed by 2-way ANOVA followed by Tukey's multiple comparisons test (P-value < 0.05 [Supplementary Data Set 3]).
To corroborate the differences in cold-induced ROS levels in Wt and the 2 types of mutants, we analyzed the relative increase in leaf superoxide by nitroblue tetrazolium (NBT) staining (Doke 1983; Hoffmann et al. 2013). NBT staining of Wt tissue at the start of cold treatment and after 4 d at 4 °C does not result in a detectable increase in superoxide (Fig. 10C). However, both types of mutants showed a stronger NBT staining after 4 d at 4 °C than at the beginning of cold treatment (Fig. 10C).
FAX1 overexpressor plants exhibit altered levels of metabolites when exposed to cold temperatures
To gain additional insight into metabolic causes for the impaired cold and frost tolerance pattern of FAX1 overexpressor lines, we analyzed the levels of 71 primary metabolites via GC-MS. Thus, Wt plants and both FAX1 overexpressor lines were grown for 28 d at 21 °C prior to growth at 4 °C for 2 wks.
When cultivated at 4 °C for 2 wks, the FAX1 overexpressor lines showed the largest differences in their metabolic readjustment compared with correspondingly grown Wt plants (Fig. 11, Supplementary Table S4). The 2 FAX1 overexpressor lines contained nearly doubled levels of β-alanine, asparagine, serine, and ethanolamine, 1.7-fold increased amounts of glycerol-phosphoglycerol, and 1.7-fold more malate when compared with Wt (Fig. 11). Similar to malate, 3 further intermediates of the tricarboxylic acid cycle, namely citrate, fumarate, and succinate, were also significantly higher in cold-treated FAX1 overexpressors than in Wt plants (Fig. 11). In addition, cold-treated FAX1 overexpressors contained about 38% more proline, about twice as much putrescine, and 2.3-fold more spermidine when compared with corresponding Wt, whereas the trehalose concentration in FAX1 Ox#2 plants was substantially lower, compared with Wt (Fig. 11).
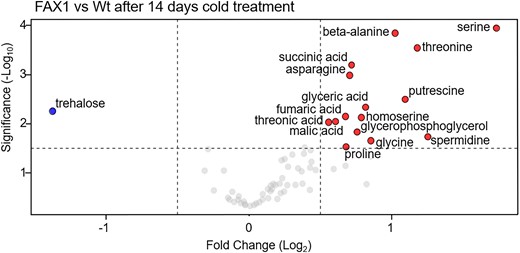
Volcano plot of the metabolic differences measured between Wt and FAX1 Ox#2 rosette leaves, cold treated for 14 d at 4 °C. The dots represent decreased and increased metabolites with a log2 fold change ≥ 0.5 and P-value ≤ 0.05. The complete data set is available in Supplementary Table S4. Significant differences between the 21 °C and 4 °C treatments were analyzed using a t test (Supplementary Data Set 3).
Discussion
The dynamic modification of organelle proteomes allows plants to achieve new homeostatic levels and cope with challenging environmental conditions (Taylor et al. 2009). As seen in many systems, cellular mRNA levels and the respective protein amounts do not necessarily correlate to a high degree (Gygi et al. 1999; Greenbaum et al. 2003; Koussounadis et al. 2015). Thus, other factors are also important for controlling protein abundance. The chloroplast envelope proteome undergoes substantial remodeling in response to changes in light or temperature conditions (Knopf et al. 2012; Nishimura et al. 2016; Adam et al. 2019; Mielke et al. 2020), and it can thus be expected that proteases must play a role in this.
Interestingly, cold-induced changes in the proteome are accompanied by modifications of membrane lipids. These modifications maintain membrane fluidity at low temperatures and stabilize membrane integrity (preventing rigidification) to ensure proper organelle function (Moellering et al. 2010; Zheng et al. 2011; Barnes et al. 2016; Barrero-Sicilia et al. 2017; Guo et al. 2018). Lipid biosynthesis, especially for the generation of phospholipids, requires FA export from the chloroplast. FAX1 is the best characterized chloroplast envelope–located protein presumed to be involved in this transport process so far (Li, Gügel, et al. 2015; Li, Zheng, et al. 2015; Li et al. 2016; Xiao et al. 2021).
In Arabidopsis, FAX1 abundance rapidly decreases after transfer to cold temperatures, while other envelope proteins increase (Trentmann et al. 2020). Taking the proteins NTT (the chloroplast ATP importer (Tjaden et al. 1998) or MEX1 (the chloroplast maltose exporter) (Niittylä et al. 2004) as examples, we demonstrated that the relative changes of these carriers are key to proper cold tolerance (Trentmann et al. 2020). However, in the case of FAX1, it is unknown whether the decreased protein abundance is a controlled process required to tolerate low environmental temperatures. In addition, no protease has been discovered that could mechanistically explain the decreased abundance of selected envelope-associated proteins in the cold.
Compared with RBL10 and FTSH11 mRNAs, the RBL11 transcript accumulates early after cold exposure (Fig. 1A). This correlation is a first indication of a specific molecular interaction between RBL11 and FAX1, and a physical contact between these 2 proteins, which is a prerequisite for FAX1 degradation, was confirmed by BiFC analysis (Fig. 3). The suggestion of a specific effect of RBL11 on FAX1 abundance in the cold is supported by 2 observations. First, cold-induced FAX1 degradation did not occur in rbl11 mutants (Fig. 1E), whereas rbl10 or ftsh11 mutants degraded FAX1 in the cold similarly to Wt plants (Fig. 1E). Second, DEX-induced expression of RBL11 leads to a decrease in FAX1 protein (Fig. 4C).
In general, RBL11 appears to impact the levels of multispanning membrane proteins like FAX1 (Li, Gügel, et al. 2015; Li, Zheng, et al. 2015), along with other rhomboid family members found in bacteria such as GlpG from Escherichia coli (Erez and Bibi, 2009). However, since DEX-induced expression of RBL11 leads to FAX1 degradation only at cold temperature and not at 21 °C (Fig. 4C), we propose that posttranslational modification of RBL11 and/or FAX1 is a prerequisite for catalytic protease activity (Fig. 4C). The observation that RBL11 activity might also be involved in the dynamic change TGD5 and TGD2 (Table 1), 2 components of the TGD complex involved in the unidirectional ER to plastids import of eukaryotic lipids (Xu et al. 2010; Li-Beisson et al. 2017), points to a central function of this protease in modification of plant lipid homeostasis under challenging temperature conditions. Notably, RBL10, the closest RBL11 homolog, has also been shown to affect lipid metabolism as it interacts with ACYL CARRIER PROTEIN4 (ACP4) and modulates MGDG biosynthesis (Lavell et al. 2019; Xu et al. 2023). Because rbl11 mutants exhibit a shift toward eukaryotic lipid biosynthesis, which is due to decreased FAX1 abundance but not observed in the Wt (Figs. 3 and 6, Supplementary Fig. S2), we hypothesize that rbl11 cells attempt to reduce the unintended stimulation of eukaryotic lipid biosynthesis by downregulating the core plastid lipid importer TGD (Table 1). This assumption is supported by the observation that Arabidopsis, as a 16:3 plant, normally stimulates the plastid membrane lipid pathway after exposure to cold conditions (Li, Gügel, et al. 2015; Li, Zheng, et al. 2015; Yu et al. 2023).
Apart from FAX1 and TGD components, which act as substrates for RBL11, it is worth mentioning that the protochlorophyllide oxidoreductase PORA strongly accumulates in rbl11 mutants (Table 1). PORA is responsible for the stromal conversion of protochlorophyllide to chlorophyllide, and increased protochlorophyllide oxidoreductase activity leads to ROS formation (Pattanayak and Tripathy 2011). Thus, RBL11 might not only contribute to the regulation of FAX1 activity but also influence envelope-located mechanisms preventing cold-induced ROS formation.
In cold conditions, rbl11 mutants exhibit higher FAX1 protein levels than observed in the Wt (Fig. 1E) and their lipid composition is shifted toward accumulation of phospholipids (Fig. 5). The latter observation is in accordance with both the function of FAX1 as a chloroplast to ER FA export protein (Li, Gügel, et al. 2015; Li, Zheng, et al. 2015; Li et al. 2016; Li-Beisson et al. 2017; Takemura et al. 2019; Tian et al. 2019) and the function of the ER as the cellular site of phospholipid biosynthesis (Li, Gügel, et al. 2015; Li, Zheng, et al. 2015; Li et al. 2016; Li-Beisson et al. 2017; Takemura et al. 2019; Tian et al. 2019). In fact, apart from a stimulation of the eukaryotic pathway of lipid biosynthesis in the cold, rbl11 plants and FAX1 overexpressors share further similarities, e.g. impaired tolerance to low temperatures and frost (Figs. 1, 2, and 7) and increased levels of anthocyanins, ROS, and BAP1 transcripts in the cold when compared with Wt (Fig. 10, A to C). The latter changes are independent molecular markers for a reinforced stress situation in mutants (Chalker-Scott 1999; Choudhury et al. 2017).
Although in sum these similarities are indicative for an important function of the downregulation of FAX1 during cold tolerance, a detailed analysis of the impact of FAX1 on this process cannot be made in the rbl11 mutants, since RBL11 acts on several inner envelope proteins, which trigger pleiotropic effects in rbl11 plants (Supplementary Tables S1 and S3 and Table 1). Thus, the impact of FAX1 on cold and frost tolerance was analyzed using FAX1 overexpressors. Although the relative abundances of FAX1 mRNA and protein differed substantially between Ox#2 and Ox#4 mutants, it turned out that the physiological responses of the 2 plant lines were very similar (Li, Gügel, et al. 2015; Li, Zheng, et al. 2015; and below). Accordingly, these lines are suitable for experiments searching for a potential impact of the cold-induced downregulation of FAX1 levels for tolerance to low temperatures.
The observation that FAX1 overexpressor leaves exhibited higher levels of ER-derived membrane lipids (PE, PC) when exposed to cold temperature (Fig. 8 and Supplementary Fig. S2B) indicates that the cold-induced decrease of FAX1 in Wt (Trentmann et al. 2020) is a limiting factor for synthesis of lipid backbones via the eukaryotic pathway at low temperature. Our assumption that a relative stimulation of lipid biosynthesis in the ER is causative for increased total levels of galacto- and phospholipids in FAX1 overexpressors gains independent support by observations made on mutants lacking the envelope proteins TGD2 or TGD3. Corresponding loss-of-function mutants show decreased levels of MGDG and DGDG with ER-derived DAG backbones, while ER-borne phospholipid levels are increased (Awai et al. 2006; Lu et al. 2007). In other words, a higher substrate availability such as acyl residues at the ER might lead to increased levels of membrane lipids with ER-derived DAG backbones (Fig. 12).
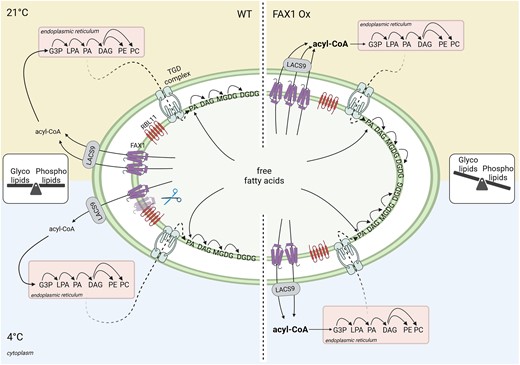
Proposed model for the influence of the FAX1 abundance, controlled by the envelope-located RBL11, on cold adaptation via balancing the glyco- and phospholipid contents in Arabidopsis. In Wt, the glyco- and phospholipid levels are balanced to gain ideal conditions for growth and development under diverse environmental conditions. During low temperature, RBL11 interacts with FAX1, which leads to a decrease of FAX1 abundance. The associated reduced export of FAs, and concomitant slowdown of the eukaryotic pathway for lipid biosynthesis, seems to be an efficient mechanism for cold acclimation. In FAX1-overexpressing (Ox) plants however, the permanent increased export of FAs from the chloroplast, which stimulates lipid biosynthesis in the ER, leads to a shift to phospholipid synthesis. In FAX1 Ox lines, RBL11 is unable to decrease FAX1 protein abundance, and the disturbed glyco- to phospholipid ratio impairs the efficient acclimation to cold temperatures, resulting in cold- and frost-sensitive mutants.
MGDG is the most abundant chloroplast-located galactolipid (Dorne et al. 1990; Kobayashi 2016), and in the 16:3 plant Arabidopsis, 2 main molecular species occur, i.e. plastid-derived 34:6 type MGDG and, as ER derived, 36:6 type MGDG (Boudière et al. 2014). When compared with the Wt, Ox#4 plants grown at 21 °C exhibit a relative decrease of 34:6 type MGDG, which is nearly balanced by a corresponding increased level of 36:6 type MGDG (Fig. 8C), and the proportion of 34:6 DGDG is decreased, while 36:6 DGDG is increased when comparing the Wt with Ox#4 plants grown at 4 °C (Fig. 8D). Thus, the latter observation indicates that the stimulated FA export in Ox#2 and Ox#4 plants leads to a shift from plastid-derived to ER-derived galactolipids. Such flexible shift of lipid biosynthesis from plastids to the ER has already been observed for mutants lacking plastid glycerol-3-phosphate acyltransferase (ACT) activity (Falcone et al., 2004; Lusk et al., 2022), and in act1 mutants (synonymous: ats1), a stimulation of membrane lipid biosynthesis in the ER largely compensates for the impaired prokaryotic lipid biosynthesis (Kunst et al. 1988). The observation that FAX1 overexpressors exhibit not only an increased ratio of 36:6 to 34:6 type MGDG (Fig. 8C) but also increased ratios of 36:6 to 34:6- and 36:6 to 34:3-type DGDGs (Fig. 8D) further underlines the shift from prokaryotic to eukaryotic lipid biosynthesis in these lines. Finally, the increase of the relative proportions of the 2 most abundant phospholipids, PC and PE, supports the conclusion that the eukaryotic lipid biosynthesis pathway is stimulated in FAX1 overexpressors.
The analyses of the molecular responses of the FAX1 mutants to cold and freezing conditions showed that these changes are associated with a strongly impaired ability to resist low environmental temperatures, as evidenced by an increased number of wilted leaves in the cold and a decreased ability to recover from freezing (Figs. 2 and 7). A similar gradual decay of leaves was also seen for other Arabidopsis mutants exhibiting decreased amounts of polyunsaturated FAs in membrane lipids (Miquel et al. 1993) and of course for the rbl11 mutant (Fig. 1). It seems likely that the increased number of wilted leaves of FAX1 overexpressors when grown at 4 °C (Fig. 6, E and F) is the result of an impaired photosynthetic performance (Fig. 9, A and C).
The exact reasons for these marked effects are not clear, but it was shown that changes in the composition of membrane lipids, induced by modifications of different lipid biosynthesis genes, affect photosynthetic properties (Botté et al. 2011; Kobayashi 2016; Gao et al. 2020). Thus, it seems conceivable that the altered chloroplast membrane composition observed in FAX1 overexpressors (Supplementary Fig. S2 and Fig. 8) is causative for the impaired photosynthetic performance. Indeed, the decreased Fv/Fm ratio of FAX1 overexpressor plants (Fig. 9, A and B) is an indicator of impaired PSII function (Murchie and Lawson 2013) and a temperature-stressed PSII can lead to ROS production (Pospíšil 2016), as observed in FAX1 overexpressor plants (Fig. 10, C to F). However, it cannot be excluded that the marked effects of RBL11 mutation or FAX1 overexpression on PC and PA levels (Figs. 5, C and D and 8, A and B) also contribute to ROS accumulation (Fig. 10). This is because the signaling molecule PA has been shown to bind directly to the plasma membrane NADPH oxidoreductase RBOHD, leading to the activation of this enzyme. Accordingly, this process stimulates O2− production, which ultimately leads to H2O2 accumulation (Zhang et al. 2009).
Wild-type plants and FAX1 overexpressor lines exhibit a quite similar metabolite pattern when grown at 21 °C. In contrast, the metabolite composition of FAX1 Ox plants during growth at 4 °C is to some degree indicative for reinforced cold stress. For example, the comparably high accumulation of polyamines in the form of putrescine and spermidine and of amino acids like proline and asparagine or the accumulation of the nonproteinogenic amino acid β-alanine (Fig. 11) represents independent evidences for a pronounced metabolic response to cold temperatures (Alet et al. 2011; Liang et al. 2013; Zhang et al. 2016; Marco et al. 2019; Parthasarathy et al. 2019). β-alanine is a metabolic indicator for abiotic stress (Parthasarathy et al. 2019), and it also acts as precursor for CoA synthesis and is thus required for FA and phospholipid biosynthesis (Perrett et al. 2017). Therefore, β-alanine accumulation is coincident with higher total levels of galacto- and phospholipids in FAX1 overexpressors in the cold (Fig. 11A). Under many stress conditions, asparagine and proline levels change similarly (Curtis et al. 2018). While the exact function of asparagine during stress is unclear, the role of proline as a general stress metabolite is well established (Liang et al. 2013; Ghosh et al. 2022). For example, proline is able to diminish PSII defects caused by rising ROS levels (Alia and Mohanty 1997) and because cold-treated FAX1 overexpressors exhibit defects in PSII activity (Fig. 9, A to C), the induce proline accumulation might represent a process to tune down such negative effects.
In summary, we propose a model in which the envelope protease RBL11 is responsible for the degradation and downregulation of FAX1 in cold-treated Arabidopsis plants (Fig. 12). This process represents a molecular response that is critical for optimal low temperature acclimation. Most likely, the initial cold response in Wt plants is represented by a preference for the prokaryotic lipid synthesis pathway to protect chloroplast membranes and the photosynthetic machinery from cold damage. Since the corresponding FAX1 mRNA is not decreased in cold-treated Wt plants (Fig. 6A), the decreased FAX1 protein level is most likely due to a specific effect of RBL11. In the near future, it will be interesting to search for factors that activate RBL11 and/or convert selected membrane proteins on the inner envelope proteins into specific substrates (Fig. 12). It seems worthwhile to mention that the cold-induced degradation of FAX1 further supports findings on the importance of various chloroplast envelope–associated processes for plant cold and frost tolerance (Moellering et al. 2010; Barnes et al. 2016; Guan et al. 2019; Schwenkert et al. 2023).
Materials and methods
Plant cultivation and growth conditions
Arabidopsis (Arabidopsis thaliana) ecotype Columbia (Col-0) and transgenic plants were sown on standard soil (type ED73, Einheitserde Patzer; Sinntal-Altengronau, Germany) with 10% (v/v) sand, stratified at 4 °C for 48 h, and then grown under short-day regime (10 h light/14 h dark) at 60% relative humidity and 120 µmol/m2/s light intensity (NS1 Valoya growth lights, Helsinki, Finland) at 21 °C, representing standard growth conditions. For cold treatment, plants were grown for 21 d at 21 °C and then transferred to a cultivation chamber (Fitotron SGR223, Weis-Gallenkamp Technik, Heidelberg, Germany) and incubated for several days at 4 °C, while all other parameters were kept constant.
We described the FAX1 Ox#2 and FAX1 Ox#4 lines earlier (Li, Gügel, et al. 2015; Li, Zheng, et al. 2015). rbl10 mutants (Lavell et al. 2019) were provided by Dr. Christoph Benning (Michigan State University, Wisconsin, United States), and ftsh11 and rbl11 mutants (Knopf et al. 2012; Adam et al. 2019) were provided by Dr. Zach Adam (Hebrew University, Jerusalem, Israel).
For RNA extraction and metabolite and anthocyanin analysis, Arabidopsis rosette leaves were collected 5 h after onset of light, transferred immediately into liquid nitrogen, and stored at −80 °C until preparation. Leaf material for chloroplast envelope isolation was used directly after harvesting 1 h before the start of illumination. For lipid isolation, rosette leaves were collected and directly transferred in a glass tube containing boiling water.
Generation of RBL11 overexpressor lines
The cloning steps to generate the DEX-inducible RBL11 overexpression lines were performed using S7 Fusion Polymerase (MD-S7-100, Mobidiag, Espoo, Finland). The sequence of BirA-HA was first amplified from the Vector pcDNA3.1 MCS-BirA(R118G)-HA (Roux et al. 2012) using gene-specific primers and ligated C-terminally of RBL11 in the pBSK vector (Short et al. 1988) by overlap extension PCR. The RBL11-BirA-HA construct was amplified using gene-specific primers with attB-sites attached and inserted first into pDONR and then into the destination vector pTA7001-DEST (Aoyama and Chua 1997) via the Gateway cloning system.
Wild-type Arabidopsis plants were transformed via floral dip using Agrobacterium tumefaciens (GV3101). Positive transformed plants were selected by hygromycin selection (Harrison et al. 2006) and confirmed by Western blot analysis using an HA antibody after induction of leaves with 30 µm DEX. Sequences of gene-specific primers, which were used for cloning, are provided in Supplementary Data Set 2.
Protein extraction from RBL11 overexpressor plants
Prior to protein extraction, ∼50 leaves of Arabidopsis Wt or RBL11-HA overexpressor plants were cut and placed in 30 µm DEX or water, as control. Leaves were incubated on a shaker for 48 h in a plant chamber at either 21 °C or 4 °C. Preparation of soluble and insoluble (membrane) protein fraction and detection of RBL11-HA using HA antibody via immunoblot was performed as described earlier (Khan et al. 2018).
Arabidopsis chloroplast envelope preparation
Arabidopsis chloroplast envelope membranes were isolated according to an established protocol (Bouchnak et al. 2018) with few modifications. In a cold room (4 °C), before onset of light, 100 to 200 g of rosette leaves were harvested from 6-wk-old plants and ground in a Waring blender (3 cycles, each of 2 s, average intensity) in grinding medium (Tricine-KOH [20 mm, pH 8.4], sorbitol [0.4 m], EDTA [10 mm, pH 8], NaHCO3 [10 mm], BSA (0.1% [w/v]). The homogenate was filtered through 1 layer of Miracloth and centrifuged for 2 min at 2,070 × g at 4 °C. The supernatant was discarded, and the sediment was gently resuspended on ice with a soft natural bristle paint brush in washing medium (1×) (Tricine-KOH [10 mm, pH 7.6], sorbitol [0.4 m], MgCl2 [2.5 mm], and EDTA [1.25 mm]) with a final volume of the combined chloroplast solutions = 24 mL). Six mL of the suspension was equally distributed and loaded on top of 4 continuous Percoll (Sigma Aldrich, Heidelberg, Germany) gradients (containing 50% Percoll/0.4 m sorbitol, prepared by centrifugation at 38,700 × g, for 55 min at 4 °C). Loaded gradients were centrifuged for 10 min at 13,300 × g, 4 °C using a swinging-bucket rotor. The intact chloroplasts present in the lower phase were retrieved with a 10 mL pipet. The intact chloroplast suspension was washed twice with 30 mL washing buffer (1×) and centrifuged for 2 min at 2,070 × g at 4 °C. After washing, the purified chloroplasts were lysed by resuspending in hypotonic medium (MOPS [10 mm, pH 7.8], MgCl2 [4 mm], phenylmethylsulfonyl fluoride [PMSF] [1 mm, dissolved in isopropanol], benzamidine hydrochloride hydrate [1 mm], and ε-amino caproic acid [0.5 mM]). Three mL of lysed chloroplasts were loaded on top of 2 prepared sucrose gradients (4 mL of 0.93 m, 3 mL of 0.6 m, and 2.5 mL of 0.3 m sucrose). Gradients were ultracentrifuged for 1 h at 70,000 × g, 4 °C in a swinging-bucket rotor. The yellow band of both gradients (containing the envelope fraction) was retrieved and pooled in 1 tube. The envelope suspension was washed in 12 mL membrane washing buffer medium (MOPS [10 mm, pH 7.8], PMSF [1 mm], benzamidine hydrochloride hydrate [1 mm], ε-amino caproic acid [0.5 mm], and ultracentrifuged again for 1 h at 110,000 × g, 4 °C). Supernatants were aspirated by using a water pump. Approximately 100 µL of membrane washing buffer was used to resuspend the envelope sediment. Isolated envelopes were stored in liquid nitrogen until use.
BiFC for interaction studies
For the cloning of the BiFC constructs, the full-length sequences of RBL11, FAX1, and FTSH11 were used. The coding sequences were amplified by PCR using S7 Fusion Polymerase (MD-S7-100, Mobidiag, Espoo, Finland) and inserted first into pDONR and then into the pUBC-cYFP and the pUBC-nYFP vectors via the Gateway cloning system (Grefen et al. 2010). Half of a YFP (nYFP or cYFP) is thus fused to the C-terminus of RBL11, FAX1, or FTSH11. The resulting constructs were then transformed into A. tumefaciens strain GV3101. Transient expression in N. benthamiana leaves of RBL11, FAX1, and FTSH11, each fused to an nYFP or cYFP, was performed as described (Walter et al. 2004). N. benthamiana leaves were infiltrated through the lower epidermis. After 5 d, leaves were analyzed using a Leica TCS SP5II fluorescence microscope (Leica Instruments, Wetzlar, Germany) (514 nm excitation and 525 to 582 nm detection of emission through an HCX PL APO 63 × 1.2 W water immersion objective).
Sequences of gene-specific primers, which were used for cloning, are provided in Supplementary Data Set 2.
Frost recovery experiment
For detection of the ability to recover from frost, a freezing tolerance test was performed according to an established approach (Trentmann et al. 2020; Cvetkovic et al. 2021). Survival rates and the numbers of wilted leaves were documented after 7 d of recovery under standard growth conditions.
PAM fluorescence measurements
Photosystem II parameters at constant light intensities were monitored using an imaging PAM M-Series IMAG-K7 and the ImagingWinGigE V2.56p (WALZ, Würzburg, Germany) software. Induction curve settings were on default with 110 PAR as light intensity, 40 s delay, and 20 s clock-time. Dark adaptation of plants lasted 10 min, followed by a 615 s long measurement monitoring PSII capacity (Fv/Fm), PSII effective photochemical quantum yield (Y(II)), quantum yield of light-induced nonphotochemical fluorescence quenching (Y(NPQ)), and quantum yield of nonregulated energy dissipation (Y(NO)) (Genty et al. 1989; Kramer et al. 2004). All plants analyzed (Col-0, FAX1 Ox#2, and FAX1 Ox#4) were grown for 28 d under short-day conditions (10/14 h; 110 µE) at room temperature (RT) before being shifted for the duration of 6 wks to 4 °C (10/14 h, 110 µE). PAM measurements were carried out on the day of the shift to 4 °C, after 1 or 6 wks in the cold.
Determination of anthocyanin content
For anthocyanin quantification, 1 mL of extraction buffer composed of H2O, propanol, and HCl (81:18:1) was added to 100 mg of fine grounded rosette plant material and incubated for 3 min at 95 °C while shaking at 650 rpm and stored overnight at RT in full darkness. After centrifugation for 15 min at 12,500 rpm at RT, the supernatant was used for photometric quantification at E1 = 535 nm and at E2 = 650 nm. The extinction was determined and corrected (Ecorr = [E535 − {2.2*E650}]/mg FW).
Metabolomics
Metabolite profiling was performed according to established protocols (Roessner et al. 2001; Lisec et al. 2006; Erban et al. 2007). In brief, from 4 plants per genotype and growth condition, 50 mg fresh weight (Fw) of ground rosette material was mixed, in a 1.5 mL reaction tube, with 180 µL of cold (−20 °C) methanol containing internal standards (10 μL ribitol, 0.2 mg/mL in water, and 10 μL 13C-sorbitol, 0.2 mg/mL in water). After 15 min of incubation at 70 °C, the extract was cooled down to RT and carefully mixed with 100 μL of chloroform and 200 µL of water. To force phase separation, a 15 min centrifugation step at full speed was performed. Fifty μL of the upper (polar) phase was dried in vacuo and stored at −80 °C. For derivatization, the pellet was resuspended in 10 μL of methoxyamin hydrochloride (20 mg/mL in pyridine) and incubated for 90 min at 40 °C. After addition of 20 µL of BSTFA (N,O-bis[trimethylsilyl]trifluoroacetamide) containing 2.5 μL retention time standard mixture of linear alkanes (n-decane, n-dodecane, n-pentadecane, n-nonadecane, n-docosane, n-octacosane, n-dotriacontane), the preparation was incubated at 40 °C for further 45 min.
One μL of each sample was injected into a GC–TOF–MS system (Pegasus HT, Leco, St Joseph, United States). Samples were automatically processed by an autosampler system (Combi PAL, CTC Analytics AG, Zwingen, Switzerland). Helium acted as carrier gas at a constant flow rate of 1 mL/min. GC was performed on an Agilent GC (7890A, Agilent, Santa Clara, CA, United States) using a 30 m VF-5 ms column with 10 m EZ-Guard column. The temperature of the split/splitless injector was set to 250 °C, as well as the transfer line and the ion source. The initial oven temperature (70 °C) was linearly increased to a final temperature of 350 °C by a rate of 9 °C/min. Metabolites were ionized and fractionated by an ion pulse of 70 eV. Mass spectra were recorded at 20 scans/s with an m/z 50 to 600 scanning range. Chromatograms and mass spectra were evaluated using ChromaTOF 4.72 and TagFinder 4.1 software (Luedemann et al. 2008).
RNA extraction, cDNA synthesis, and qRT-PCR
RNA was extracted from 50 mg of frozen and fine ground rosette leaf material from 4 biological replicates per genotype and growth condition using the NucleoSpin RNA Plant Kit (Macherey-Nagel, Düren, Germany), according to the manufacturer's protocol. The synthesis of cDNA from RNA was performed with the qScript cDNA Synthesis Kit (Quantabio, Beverly, MA, United States). Primers used for gene expression analysis via qRT-PCR are listed in Supplementary Data Set 2. AtUBQ was used as reference gene for normalization.
NBT staining
For ROS staining, O2− was detected by NBT staining (Fryer et al. 2002) in whole rosettes of 2-wk-old Arabidopsis plants cultivated as described above. For cold treatments, plants were transferred to 4 °C for 4 d, while control plants were kept under standard conditions.
Measurement of galacto-, phospho-, and sulfolipids
For the analysis of lipids, plants were cultivated as described above and transferred from standard conditions to 4 °C for 2 wks, 10 wks, or used directly. From 5 plants per genotype, 100 mg Fw of rosette leaf material was harvested and immediately placed into a glass tube containing boiling water to prevent degradation of phospholipids through phospholipase D activity. The lipid extraction was performed with chloroform/methanol after deactivation of lipase activities by boiling the tissue in water as described earlier (Gasulla et al. 2013). Lipids were measured by tandem MS (Q-TOF 6530 Agilent Technologies) and quantified by MS/MS experiments with internal standards following the strategy developed earlier (Gasulla et al. 2013; Welti et al. 2002).
Immunoblotting
Per lane, 8 µg of isolated chloroplast envelope protein or 30 µg of freshly prepared protein extract from Arabidopsis leaf material were separated via SDS–PAGE (12%). The proteins in the gel were transferred onto a nitrocellulose membrane by a semi-dry blotting system (TransBlot Turbo Transfer System, BIO RAD, Göttingen, Germany). The membrane was blocked in phosphate-buffered saline plus 0.1% (v/v) Tween 20 (PBS-T) with 3% milk powder for 1 h at RT and then washed 3 times in PBS-T for 10 min. The membrane was incubated with a polyclonal rabbit antibody raised against FAX1 (Li, Gügel, et al. 2015; Li, Zheng, et al. 2015) overnight at 4 °C at 1:1,000 dilution. After 3 times of washing with PBS-T for 10 min, the membrane was incubated with a horseradish peroxidase (HPR)-conjugated anti-rabbit antibody (Promega, Walldorf, Germany) diluted 1:10,000 in PBS-T with 3% milk powder for 1 h. The immunoreaction was visualized by chemiluminescence using ECL Prime Western blotting reagent (GE Healthcare, Karlsruhe, Germany) and a Fusion Solo S6 (Vilber-Lourmat, Eberhardzell, Germany).
Peptide MS
Enriched envelope fractions were precipitated in 80% acetone and digested in solution using Lys-C and trypsin, and resulting peptides were desalted as previously described (Hammel et al. 2018). Peptide MS was performed using a nanoUHPLC-IM-MS system (nanoElute coupled to timsTOF Pro2, Bruker Daltonics, Bremen, Germany). Samples were directly loaded on a 25 cm, 75 µm ID, 1.6 µm particle size, C18 column with integrated emitter (Odyssey/Aurora IonOpticks, Melbourne, Australia) set to 50 °C, and peptides were separated under a flow rate of 0.3 µL/min using buffers A (water, 0.1% formic acid) and B (acetonitrile, 0.1% formic acid). The gradient employed ramped from 2% B to 25% B within 67 min and then to 37% B within 10 min, followed by washing and equilibration steps. The MS was operated in positive mode, electrospray voltage was set to 1.4 kV, and spectra were recorded from 100 to 1,700 m/z. A total of 10 MS/MS PASEF ramps (1/K0 0.6 to 1.43 V*s/cm2) with 100 ms duration were acquired per cycle, and target intensity for MS/MS was set to 14,500 whereafter the precursors were excluded from fragmentation for 0.4 min.
Protein identification and quantification
Acquired data were searched against the Uniprot protein sequences for A. thaliana (UP000006548) using the FragPipe v19.1 processing pipeline choosing the default LFQ-MBR workflow with minor modifications: peptide length was set to a minimum of 6 amino acids, missed cleavages were set to 3, and normalization of intensities across runs was omitted. MS raw and processed data have been deposited at the ProteomeXChange Consortium via the PRIDE partner repository (Perez-Riverol et al. 2021) with the data set identifier PXD041219 and in Supplementary Data Set 1 “Supplemental_Data_Set_1_Analysis_complete.”
Data normalization and missing value imputation
Prior to computation of protein-level statistics, replicate groups were normalized using the median-of-ratios method (Anders and Huber 2010). Subsequently, we computed global variance estimates and local gene-wise mean estimates to impute missing data points as independent draws from normal distributions. When a replicate group did not contain any measurement, the normal distribution was centered at an intensity corresponding to the 5% quantile of all intensities. Proteins were excluded from statistical analysis according to 2 filter criteria. First, proteins were considered ineligible for downstream analysis if there was no biological replicate group with at least 1 reading. Second, only proteins reported to be localized to the envelope membrane were considered, either by association with matching Gene Ontology terms retrieved from UniProt or by entry in the manually curated AT_CHLORO database (Ashburner et al. 2000; Bruley et al. 2012; Consortium 2021).
Statistical analyses
Statistical analyses regarding the proteomic data were based on log2 transformed imputed values. According to the experimental design which consisted of 2 factors (genotype and treatment) at each of 2 levels (Wt/mutant and normal/cold), changes in protein abundance were evaluated using 2-way ANOVA (Fisher 1925). To account for multiple hypothesis testing, we controlled the false discovery rate (FDR) by computing q-values based on the ANOVA P-values as previously described by Storey (Storey and Tibshirani 2003; Storey et al. 2004). All calculations were carried out using the FSharp.Stats library for statistical computing (Venn et al. 2023). Changes were considered to be significant if a q-value of 0.05 was not exceeded.
For the statistical analysis, GraphPad Prism 9 and Microsoft Office Excel were used in addition to the proteomic data. The heatmapper.ca software (http://www.heatmapper.ca/expression) was used to create the heat map containing the proteomic data. Significant differences between 2 groups were analyzed by 2-tailed Student's t test. The software Shiny application (https://houssein-assaad.shinyapps.io/TwoWayANOVA/) was used for letter-based representation of all pairwise comparisons using popular statistical tests in 2-way ANOVA. Statistical data are provided in Supplementary Data Set 3.
Accession numbers
Sequence data from this article can be found in the ARAMEMNON GenBank data library (http://aramemnon.uni-koeln.de/) under the following accession numbers: FAX1 (At3g57280), FTSH11 (At5g53170), RBL10 (At1g25290), RBL11 (At5g25752), and BAP1 (At3g61190).
Acknowledgments
Work in the labs from M.L., H.-H.K., A.F., M.S., T.M., and H.E.N. was supported by the Deutsche Forschungsgemeinschaft (DFG) within the SFB/Transregio (TRR) 175, The Green Hub. Work in the lab of HEN was supported by the federal research program BioComp and by the University Kaiserslautern-Landau (RPTU) during his sabbatical leave.
Author contributions
A.J. designed the research, analyzed and interpreted data, and wrote the paper with H.E.N.; M.K. performed the PAM experiments and prepared the corresponding figure; H.-H.K. interpreted the PAM data; metabolite analysis was done by M.L. as well as by F.A. and S.A. supervised by A.F.; H.P. and K.G. performed the lipid analyses supervised by P.D.; F.S. and M.S. performed the peptide MS; D.Z .and T.M. provided statistical tools and analyzed the proteomic data; J.N. and K.P. contributed to data interpretation; H.E.N. contributed to project design and data interpretation, provided overall project oversight, and wrote the paper with A.J.
Supplementary data
The following materials are available in the online version of this article.
Supplementary Figure S1. MA plot and heatmap based on proteomic data.
Supplementary Figure S2. Lipid analysis of Wt and FAX1 overexpression lines.
Supplementary Table S1. Increased proteins in rbl11 under control conditions.
Supplementary Table S2. Decreased proteins in rbl11 under control conditions.
Supplementary Table S3. Decreased proteins in rbl11 after 7 d at 4 °C.
Supplementary Table S4. Complete GC-MS metabolite data set.
Supplementary Data Set 1. Compiled proteomics results.
Supplementary Data Set 2. Primers used in this study.
Supplementary Data Set 3. Summary of statistical analysis.
Dive Curated Terms
The following phenotypic, genotypic, and functional terms are of significance to the work described in this paper:
References
Author notes
The author responsible for distribution of materials integral to the findings presented in this article in accordance with the policy described in the Instructions for Authors is Ekkehard Neuhaus ([email protected]).
Conflict of interest statement. None declared.