-
PDF
- Split View
-
Views
-
Cite
Cite
Chao Han, Wenbo Hua, Jinge Li, Yan Qiao, Lianmei Yao, Wei Hao, Ruizi Li, Min Fan, Geert De Jaeger, Wenqiang Yang, Ming-Yi Bai, TOR promotes guard cell starch degradation by regulating the activity of β-AMYLASE1 in Arabidopsis, The Plant Cell, Volume 34, Issue 3, March 2022, Pages 1038–1053, https://doi.org/10.1093/plcell/koab307
- Share Icon Share
Abstract
Starch is the main energy storage carbohydrate in plants and serves as an essential carbon storage molecule for plant metabolism and growth under changing environmental conditions. The TARGET of RAPAMYCIN (TOR) kinase is an evolutionarily conserved master regulator that integrates energy, nutrient, hormone, and stress signaling to regulate growth in all eukaryotes. Here, we demonstrate that TOR promotes guard cell starch degradation and induces stomatal opening in Arabidopsis thaliana. Starvation caused by plants growing under short photoperiod or low light photon irradiance, as well as inactivation of TOR, impaired guard cell starch degradation and stomatal opening. Sugar and TOR induce the accumulation of β-AMYLASE1 (BAM1), which is responsible for starch degradation in guard cells. The plant steroid hormone brassinosteroid and transcription factor BRASSINAZOLE-RESISTANT1 play crucial roles in sugar-promoted expression of BAM1. Furthermore, sugar supply induced BAM1 accumulation, but TOR inactivation led to BAM1 degradation, and the effects of TOR inactivation on BAM1 degradation were abolished by the inhibition of autophagy and proteasome pathways or by phospho-mimicking mutation of BAM1 at serine-31. Such regulation of BAM1 activity by sugar–TOR signaling allows carbon availability to regulate guard cell starch metabolism and stomatal movement, ensuring optimal photosynthesis efficiency of plants.
Introduction
Starch, the insoluble polymer of glucose, amylopectin, and amylose, is the most widespread and abundant storage carbohydrate in plants (Smith et al., 2005). Starch contributes to maintaining plant fitness under changing environmental conditions as its degradation functions in buffering the availability of carbon, energy, and carbon-derived metabolites in response to several physiological demands (Zeeman et al., 2010; Thalmann and Santelia, 2017). Starch metabolism and function depend on the different cell types from which the starch is derived as well as the external environmental conditions. Starch in guard cells is present at night and degrades within 1-h light exposure to promote stomatal opening (Horrer et al., 2016; Santelia and Lunn, 2017). This transitory starch breakdown in guard cells produces glucose, but not malate, maintaining the cytoplasmic sugar pool and inducing stomatal opening (Flutsch et al., 2020). The glucan hydrolases β-AMYLASE1 (BAM1) and α-AMYLASE3 (AMY3) are preferentially expressed in guard cells and are responsible for the degradation of guard cell starch in plants (Valerio et al., 2011; Horrer et al., 2016). Arabidopsis (Arabidopsis thaliana) plants lacking BAM1 and AMY3 display the guard cell-specific starch excess phenotype and decreased stomatal apertures in response to light (Horrer et al., 2016). Recently, the expression of BAM1 was reported to be induced by the phytohormone brassinosteroid (BR) and redox signal hydrogen peroxide (H2O2) to stimulate the breakdown of starch in guard cells and promote stomatal opening (Li et al., 2020). These results suggested that the fine regulation of BAM1 activity could control the degradation of starch in guard cells, thereby modulating stomatal opening.
Target of rapamycin (TOR) is an evolutionarily conserved master regulator that modulates nutrient status and energy signaling to promote cell proliferation and growth in all eukaryotes (Laplante and Sabatini, 2012; Xiong et al., 2013; Xiong and Sheen, 2015; Dobrenel et al., 2016). In plants, TOR has essential roles in regulating cell proliferation, cell size, nitrogen mobilization, glucose utilization, protein synthesis, metabolism, and hormone coordination (Xiong et al., 2013; Xiong and Sheen, 2015). Photosynthesis-derived glucose activates TOR to phosphorylate and activate the transcription factor E2Fa for root meristem activation (Xiong et al., 2013). Continuous auxin biosynthesis in the shoot activates TOR through a physical interaction of TOR with small GTPase Rho-like GTPase 2, promoting E2Fa and E2Fb transcription factors and activating the shoot meristem (Li et al., 2017). TOR promotes cell elongation by stabilizing the transcription factor BRASSINAZOLE-RESISTANT1 (BZR1), a master regulator of the BR signaling pathway, which balances the growth-promotion hormonal programs with carbon availability in plants (Zhang et al., 2016). In Arabidopsis, TOR inactivation was shown to arrest plant growth and led to starch and triacylglycerol accumulation (Ren et al., 2012; Caldana et al., 2013), but its molecular mechanism has not been fully elucidated. Furthermore, the role of TOR in guard cell starch metabolism and stomatal movement remains unclear.
In this study, we demonstrate that TOR kinase modulates guard cell starch metabolism by regulating BAM1 activity. TOR inactivation impairs guard cell starch and degradation and stomatal opening. Exogenous sugar supply promotes guard cell starch breakdown and stomatal opening under carbon starvation conditions such as very short photoperiod or low light photon irradiance. Sugar and TOR induce BAM1 expression via the transcription factor BZR1. Furthermore, exogenous sugar promoted BAM1 accumulation, but such sugar effects were largely abolished by TOR inactivation. Moreover, the effects of TOR inactivation on BAM1 degradation could be eliminated by inhibiting autophagy and the proteasome pathway or by the phospho-mimicking mutation of BAM1 at serine-31 (Ser-31). Our findings demonstrate that sugar and TOR finely regulate BAM1 activity at both the transcriptional and posttranscriptional levels to control guard cell starch degradation and stomatal movement.
Results
TOR is required for starch degradation in guard cells
TOR reportedly plays a critical role in starch metabolism in plants (Ren et al., 2012; Caldana et al., 2013). To determine whether TOR is involved in guard cell starch metabolism, we analyzed the guard cell starch content of wild-type Arabidopsis in the presence and absence of AZD8055, a highly specific ATP-competitive inhibitor of TOR kinase (Chresta et al., 2010; Montane and Menand, 2013). In the absence of AZD8055, guard cell starch started to be synthesized 1 h after light exposure, and accumulation peaked at midnight, then slowly degraded for late night and rapidly degraded within 1 h of light exposure (Figure 1, A and B). However, in the presence of AZD8055, starch accumulated to high levels in the guard cells and did not degrade, even upon light exposure. The effects of AZD8055 on guard cell starch metabolism were increased as the treatment time increased (Figure 1, A and B; Supplemental Figure S1A). The transitory starch breakdown in guard cells is necessary for stomatal opening. To determine whether TOR is involved in stomatal opening, we examined the stomatal apertures in intact leaves of wild-type plants with or without AZD8055 treatment. We found that AZD8055 treatment significantly inhibited stomatal opening upon light exposure (Figure 1C; Supplemental Figure S1B). These results indicated that TOR inactivation by AZD8055 treatment impairs guard cell starch degradation and stomatal opening.
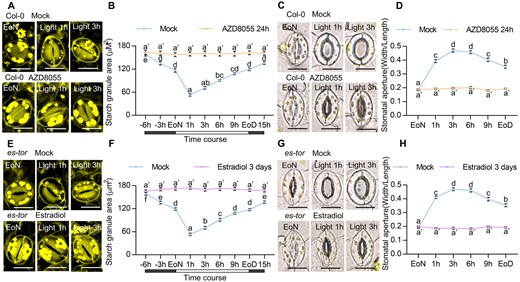
TOR promotes guard cell starch degradation. A–C, Dynamic changes of starch granules within guard cells and stomatal apertures in the cotyledon of wild-type plants with or without AZD8055 treatment. Seedlings of Col-0 plants were grown on 1/2 MS medium containing 1% sucrose under a 12-h light/12-h dark photoperiod and 100-µM m−2 s−1 light condition for 10 days, transferred to medium containing mock solution or 2-µM AZD8055 and allowed to grow for another 24 h, and then harvested at the indicated time points and immediately fixed in buffer for starch quantification (A and B) or used to directly measure the stomatal apertures (C). D and F, Dynamic changes of starch granules within guard cells and stomatal apertures in the cotyledons of es-tor plants with or without estradiol treatment. Seedlings of es-tor plants were grown on 1/2 MS medium containing 1% sucrose under a 12-h light/12-h dark photoperiod with 100- µM m−2 s−1 light condition for 7 days, transferred to medium containing mock solution or 10- µM estradiol to grow for another 3 days and then harvested at the indicated time points. Starch granules (D and E) and stomatal apertures (F) were then measured. The starch granules in guard cells or the ratio of stomatal aperture width to length from at least 100 guard cells from 8 to 15 cotyledons of 8 different plants were measured using ImageJ software. Scale bar in stomata pictures represents 20 µm. EoN means the end of light, EoD means the end of the day. Error bars indicate standard error (n = 100). Different letters above the bars indicate statistically significant differences between samples (two-way ANOVA followed by Tukey’s multiple comparisons test, P < 0.05). Supplemental Data Set S1 provides details of the statistical analyses.
To further examine the effects of TOR on the guard cell starch degradation and stomatal opening, we analyzed the guard cell starch content and stomatal apertures of an estradiol-inducible TOR RNAi line es-tor plants grown on 1/2 Murashinge & Skoog (MS) medium with or without estradiol for various days under a 12-h light/12-h dark photoperiod. Similar to the AZD8055 treatment results, estradiol supplementation for 3 days dramatically inhibited guard cell starch degradation and stomatal opening upon light exposure, confirming the critical roles of TOR in guard cell starch metabolism and stomatal opening (Figure 1, D–F; Supplemental Figure S2, A and C). With estradiol treatment for 7 days or longer, guard cell starch levels in es-tor plants were lower than those in es-tor plants with mock solution treatment at the end of night (EoN) and failed to degrade upon light exposure (Supplemental Figures S2A and S3, A–F). Besides, estradiol treatment for a longer time led to the accumulation of excess undegraded starch in whole seedlings (Supplemental Figure S2B). We also analyzed the effects of TOR on guard cell starch metabolism in the rosette leaves by spraying mock solution or 10 µM estradiol onto es-tor plants or spraying 2 µM AZD8055 onto wild-type plants, which were grown under a 12-h light/12-h dark photoperiod condition for 4 weeks. After 3 days, estradiol treatment or AZD8055 treatment increased the levels of guard cell starch at the EoN, impaired the starch breakdown and stomatal opening upon light exposure, and reduced the stomatal conductance (Supplemental Figure S4, A–I). TOR inactivation also increased the starch levels in whole leaves (Supplemental Figure S4, J and K). In addition, stomata in the es-tor plants with or without estradiol treatment opened to a similar extent in response to the fungal toxin fusicoccin (Supplemental Figure S5), which induces stomatal opening via the irreversible activation of H+-ATPase (Kinoshita and Shimazaki, 2001). Together, these results demonstrate that TOR plays an important role in the guard cell starch degradation and stomatal opening.
Sugar promotes guard cell starch metabolism under certain carbon starvation conditions
To determine whether upstream signals of TOR, such as energy and nutrient signals, regulate guard cell starch degradation and stomatal opening, we analyzed the guard cell starch content and stomatal apertures of wild-type plants that were grown under different light conditions with or without exogenous sucrose. When plants were grown under low light photon irradiance without sucrose, the starch content in guard cells was low and did not decrease after light exposure. However, guard cell starch level and degradation upon light exposure were partially recovered when exogenous sucrose was supplied (Figure 2A; Supplemental Figure S6, A–B). The plants grown under low light intensity in the absence of sucrose displayed the reduced stomatal apertures in response to light, whereas sucrose supply promoted stomatal opening (Figure 2B; Supplemental Figure S6C). Additionally, the plants grown under the 4-h light/20-h dark photoperiod without sucrose exhibited decreased guard cell starch levels and an impaired stomatal opening phenotype. Sucrose supplementation recovered the light-triggered degradation of guard cell starch and stomatal opening of plants grown under short photoperiods (Figure 2, C and D). Similarly, glucose, fructose, and maltose, but not mannitol, promoted starch accumulation in guard cells and induced starch degradation and stomatal opening upon light exposure (Supplemental Figure S7, A–D). These results indicated that sugar promotes guard cell starch metabolism and stomatal opening under certain carbon starvation conditions, such as low light quantity or short-day photoperiod conditions.
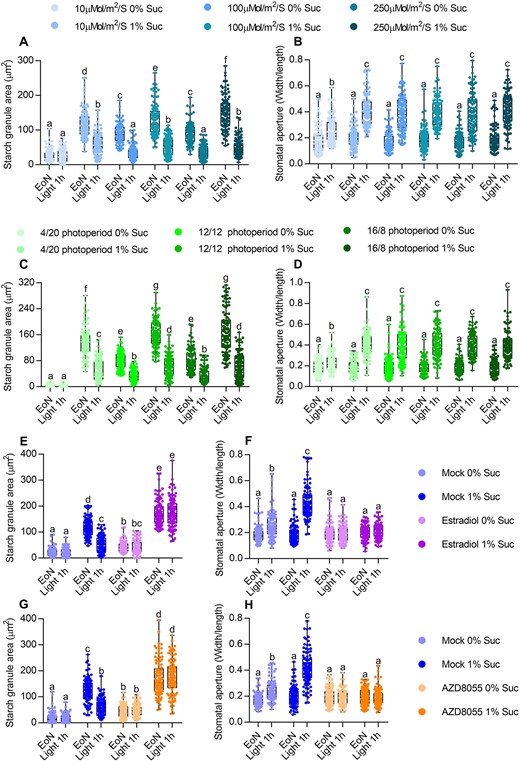
TOR is required for sucrose-promoted stomatal opening under carbon starvation conditions. A–D, Quantification of guard cell starch granules (A and C) and stomatal apertures (B and D) in the intact leaves of wild-type plants. Seedlings of wild-type plants were grown on 1/2 MS medium with or without 1% sucrose under a 12-h light/12-h photoperiod with different light intensities for 10 days (A and B) or under a different photoperiod with a 100-µM m−2s−1 light intensity for 10 days (C and D). E and F, Quantification of guard cell starch granules (E) and stomatal apertures (F) in the intact leaves of es-tor plants with or without estradiol treatment. Seedlings of es-tor plants were grown on 1/2 MS medium with or without 1% sucrose under a 12-h light/12-h dark photoperiod with 10-µM m−2 s−1 light conditions for 7 days, transferred to medium Figure 2 (Continued) containing mock solution or 10-µM estradiol to grow for another 3 days and then harvested at the indicated time points and immediately fixed in buffer for starch quantification (E) or used to directly measure the stomatal apertures (F). G and H, Quantification of guard cell starch granules (E) and stomatal apertures (F) in the intact leaves of wild-type plants with or without AZD8055 treatment. Seedlings of Col-0 plants were grown on 1/2 MS medium with or without 1% sucrose under a 12-h light/12-h dark photoperiod and 10-µM m−2 s−1 light conditions for 10 days, transferred to medium containing mock solution or 2 µM AZD8055 to grow for 24 h, and then harvested at the indicated time points. Starch granules (G) and stomatal apertures (H) were then measured. EoN means the end of light, light 1 h means the white light illumination for 1 h after the EoN. The starch granules in guard cells or the ratio of stomatal aperture width to length from at least 100 guard cells from 10 to 15 leaves of 8 different plants were measured using ImageJ software. Every measurement of stomatal starch granule area or stomatal aperture was labeled as a dot on box plot. The box extends from the 25th to 75th percentiles and plots each individual value as a point superimposed on the graph. The line in the middle of the box is plotted at the median. Different letters above the bars indicate statistically significant differences between samples (two-way analysis of variance (ANOVA) followed by Tukey’s multiple comparisons test, P < 0.05).
To investigate the functions of TOR on sugar-mediated guard cell starch degradation and stomatal opening, we analyzed the kinase activity of TOR under certain carbon starvation conditions. S6 kinase 1 (S6K1) is a substrate of TOR, and the S6K1 phosphorylation state has been used as a functional readout for sugar-dependent TOR activity (Xiong et al., 2013; Li et al., 2017; Liu et al., 2021). The total protein levels of S6K1 were similar, but the phosphorylated S6K1 of plants grown on the sugar-free medium under low light irradiance or short photoperiod conditions was substantially lower than those of plants grown under high light irradiance or long photoperiod conditions. Sucrose supply and phosphorylated S6K1 protein increased under all growth conditions (Supplemental Figure S8, A and B). These results suggested that TOR activity is low when plants are grown under carbon starvation conditions, and increases following sucrose supply. Furthermore, TOR inactivation by AZD8055 treatment or inducible RNA interference significantly attenuated the promoting effects of sucrose on guard cell starch degradation and stomatal opening under low light irradiance (Figure 2, E–H). Thus, sugar induces stomatal opening under certain carbon starvation conditions, but these effects were abolished by TOR inactivation.
Sucrose and TOR induce BAM1 expression
To determine how sucrose and TOR kinase promote the breakdown of guard cell starch under certain conditions, we examined the expression of genes involved in guard cell starch degradation in response to sucrose. The glucan hydrolases BAM1 and AMY3 are preferentially expressed in guard cells and are responsible for the degradation of guard cell starch (Horrer et al., 2016). Reverse transcription quantitative PCR (RT-qPCR) analysis showed that exogenous sucrose treatment significantly increased the expression of BAM1, but not AMY3, in plants grown under low light quantity condition (Figure 3A). Treatment with the TOR kinase inhibitor AZD8055 significantly reduced the expression of BAM1 (Figure 3B). To verify these effects, we monitored the fluorescent signals of ProBAM1:GFP transgenic plants grown on medium with or without sucrose and/or AZD8055 under a 4-h light/20-h dark photoperiod or low light quantity conditions. Sucrose treatment significantly increased, but AZD8055 treatment reduced the fluorescence intensities of ProBAM1:GFP (Figure 3, C and D; Supplemental Figure S9, A and B). These results demonstrate that sucrose and TOR induce the expression of BAM1.
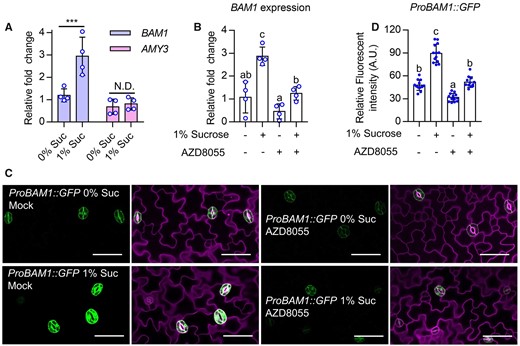
Sugar and TOR induce BAM1 expression. A and B, Quantitative RT-qPCR analysis the effects of sucrose on the expression of BAM1 and AMY3. Seedlings of wild-type plants were grown on 1/2 MS medium with or without 1% sucrose and/or 1 µM AZD8055 under 12-h light/12-h dark photoperiod with 10-µM m−2 s−1 light for 10 days. PP2A was used as an internal control. Error bars indicate standard deviation (sd) of four biological repeats. Asterisk and different letters above bars indicate statistically significant differences between the samples (two-way ANOVA followed by uncorrected Fisher’s least significant difference (LSD) multiple comparisons test, ***P < 0.001). C and D, Sucrose induced, but AZD8055 reduced the fluorescence signals intensity of ProBAM1:GFP. Seedlings of ProBAM1:GFP transgenic plants were grown on 1/2 MS medium with or without 1% sucrose and/or 1 µM AZD8055 under 12-h light/12-h dark photoperiod with 10-µM m−2 s−1 light for 10 days. GFP signal intensity from more than 10 seedlings was analyzed using ImageJ software. Error bars indicate sd (n = 10). Different letters above bars indicate statistically significant differences between samples (one-way ANOVA followed by uncorrected Fisher’s LSD multiple comparisons test, P < 0.05). green fluorescent proteins (GFP) are in green, PI-marked cell outlines are in purple. Scale bars in confocal images represent 50 μm.
BR and BZR1 play critical roles in TOR-induced expression of BAM1
Our previous study showed that the plant steroid hormone BR promotes guard cell starch degradation and stomatal opening by inducing BAM1 expression via the transcription factor BZR1 (Li et al., 2020). Sugar and TOR have been reported to stabilize BZR1 protein by inhibiting the autophagy pathway (Zhang et al., 2016). Here, we further showed that TOR inactivation led to the degradation of BZR1 in guard cells, but had weak effects on the mutant version of bzr1-1D protein, which displayed high activity due to its high binding affinity to phosphatase 2A (PP2A; Supplemental Figure S10, A–D). Thus, we wondered whether sugar and TOR regulate the expression of BAM1 through BZR1. To test this hypothesis, we analyzed BAM1 expression in wild-type, BR-deficient mutant de-etiolated 2-1 (det2-1), and BR-insensitive mutant bzr hextuple (bzr-h mutant missing of BZR1, BES1, BEH1, BEH2, BEH3, and BEH4; Chen et al., 2019) plants that were grown on 1/2 MS medium with or without sucrose under low light intensity condition. Sucrose induced the expression of BAM1 in wild-type plants, but not in det2-1 and bzr-h mutants (Figure 4A). Next, we compared BAM1 expression levels in the wild-type plants and bzr1-1D mutants with or without AZD8055 treatment. The AZD8055 treatment strongly repressed BAM1 expression in the wild-type background, but had weaker effects on BAM1 expression in the bzr1-1D background (Figure 4, C and D; Supplemental Figure S11, A–B). Together, these results indicated that BR and BZR1 play critical roles in the sugar- and TOR-promoted expression of BAM1.
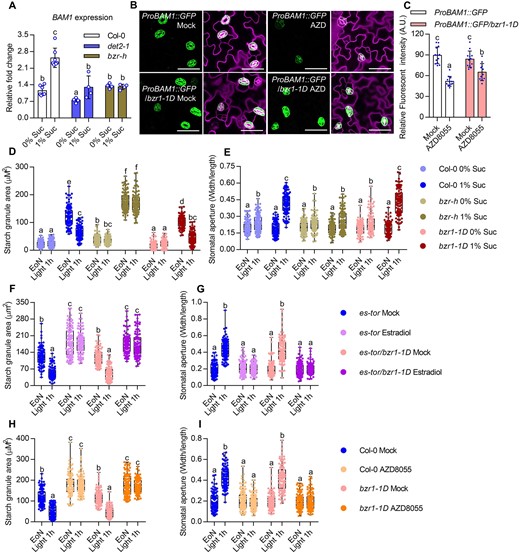
BR and BZR1 play critical roles in sugar- and TOR-induced BAM1 expression. A, Sucrose failed to induce BAM1 expression in det2-1 and bzr-h. Seedlings of Col-0, det2-1, and bzr-h were grown on 1/2 MS medium with or without 1% sucrose under a 12-h light/12-h dark photoperiod with 10-µM m−2 s−1 light for 10 days. RT-qPCR analysis BAM1 expression in wild-type and mutant plant under different conditions. PP2A was used as an internal control. Error bars indicate sd of four biological repeats. Different letters above bars indicate statistically significant differences between samples (two-way ANOVA followed by uncorrected Fisher’s LSD multiple comparisons test, P < 0.05). B and C, The gain-of-function mutant bzr1-1D partially suppressed the AZD8055-inhibited BAM1 expression. Seedlings of ProBAM1::GFP and ProBAM1::GFP/bzr1-1D transgenic plants were grown on the 1/2 MS medium with or without 1 µM AZD8055 under 12-h light/12-h dark photoperiod with 10-µM m−2 s−1 light condition for 10 days. GFP signal intensity was analyzed using ImageJ software. Error bars indicate sd (n = 10). GFP are in green, PI-marked cell outlines are in purple. Scale bars in confocal images represent 50 μm. Different letters above bars indicate statistically significant differences between samples (two-way ANOVA followed by uncorrected Fisher’s LSD multiple comparisons test, P < 0.05). D and E, Quantification of guard cell starch granules (D) and stomatal apertures (E) in the intact leaves of Col-0, bzr-h and bzr1-1D at the indicated time points. Seedlings of wild-type Col-0, bzr-h, and bzr1-1D were grown on the 1/2 MS medium with or without 1% sucrose under a 12-h light/12-h dark photoperiod with 10-µM m−2 s−1 light condition for 10 days. F and G, The bzr1-1D mutant had no significant effects on TOR-mediated guard cell starch metabolism (F) and stomatal opening (G). Seedlings of es-tor and es-tor/bzr1-1D were grown on the 1/2 MS medium containing 1% sucrose under a 12-h light/12-h dark photoperiod with 100-µM m−2 s−1 light condition for 7 days, transferred to the medium containing mock solution or 10- µM Figure 4 (Continued) estradiol to grow for another 3 days and then harvested at the indicated time points and immediately fixed in buffer for starch quantification (F) or used to directly measure the stomatal apertures (G). H and I, Quantification of guard cell starch granules (H) and stomatal apertures (I) in the intact leaves of Col-0 and bzr1-1D with or without AZD8055 treatment. Seedlings of Col-0 and bzr1-1D were grown on the 1/2 MS medium containing 1% sucrose under a 12-h light/12-h dark photoperiod with 100-µM m−2 s−1 light condition for 10 days, transferred to the medium containing mock solution or 2 µM AZD8055 to grow for 24 h, and harvested at the indicated time points. Starch granules (H) and stomatal apertures (I) were then measured. EoN means the end of light, light 1 h means the white light illumination for 1 h after the EoN. The starch granules in guard cells or the ratio of stomatal aperture width to length from at least 100 guard cells from 10 to 15 leaves of 8 different plants were measured using ImageJ software. Every measurement of stomatal starch granule area or stomatal aperture was labeled as a dot on box plot. The box extends from the 25th to 75th percentiles and plots each individual value as a point superimposed on the graph. The line in the middle of the box is plotted at the median. Different letters above the bars indicate statistically significant differences between samples (two-way ANOVA followed by Tukey’s multiple comparisons test, P < 0.05).
Considering the involvement of BZRs in sugar-induced BAM1 expression, we analyzed the guard cell starch content and stomatal apertures in wild-type plants, bzr-h and bzr1-1D mutants that were grown in 1/2 MS medium with or without sucrose under low light intensity conditions. In the absence of sucrose, guard cell starch levels were low in the wild-type, bzr-h and bzr1-1D, and the stomatal apertures failed to open fully. In the presence of sucrose, starch accumulated in the guard cells of all plants examined at the EoN, but starch levels were substantially higher in bzr-h, lower in bzr1-1D than those in wild-type plants. Upon light exposure, the guard cell starch degraded in wild-type and bzr1-1D, but not in bzr-h mutants. Correspondingly, the stomatal apertures of bzr-h were smaller than those of wild-type and bzr1-1D in the presence of sucrose after light exposure (Figure 4, D and E). Consistent with this, the BR-insensitive 1-116 (bri1-116) and det2-1 mutants displayed the accumulated guard cell starch and impaired stomatal opening phenotypes (Supplemental Figure S12, A and B). These results indicated that BR signaling is required for sugar-mediated guard cell starch metabolism and stomatal opening.
The gain-of-function mutant bzr1-1D suppresses the dwarf phenotype of the es-tor mutant (Zhang et al., 2016). To test whether bzr1-1D contributes to the TOR-mediated guard cell starch metabolism and stomatal opening, we analyzed the guard cell starch content and stomatal apertures of es-tor and bzr1-1D/es-tor plants that were grown on 1/2 MS medium containing 1% sucrose with or without 10 µM estradiol. The results showed that TOR inactivation prevented guard cell starch degradation and stomatal opening upon light exposure. The bzr1-1D/es-tor mutant displayed similar phenotypes to es-tor plants in terms of guard cell starch metabolism and stomatal movement (Figure 4, G and H). Consistent with this finding, the AZD8055 treatment significantly inhibited the guard cell starch degradation and stomatal opening in both wild-type plants and bzr1-1D mutant (Figure 4, I and J). These results suggested that TOR plays a more dominant role than BZR1 in guard cell starch metabolism and stomatal movement.
Sucrose and TOR induce BAM1 protein accumulation
Given that the bzr1-1D mutant could restore BAM1 expression, but had no strong effects on guard cell starch degradation of es-tor mutants, TOR might regulate BAM1 at the posttranscriptional level. To test this hypothesis, we investigated the effects of sucrose on BAM1 protein levels in Pro35S:BAM1-YFP transgenic plants that were grown on medium with or without sucrose under different light conditions. Sucrose treatment increased the protein levels of BAM1-YFP, especially under the 4-h light/20-h dark photoperiod or 10-μM m−2 s−1 light photon irradiance conditions (Figure 5, A and B). We also evaluated the effects of different types of sugar on the accumulation of BAM1. Sucrose, glucose, fructose, and maltose all significantly induced BAM1 accumulation (Supplemental Figure S13).
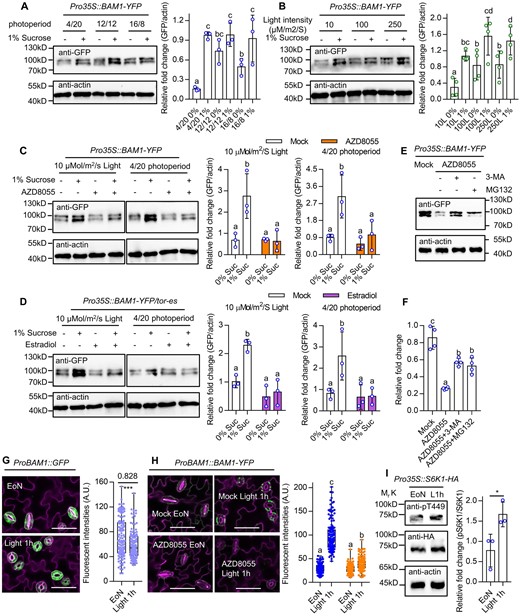
Sugar and TOR promote the accumulation of BAM1 protein. A and B, Sucrose supply increased BAM1 protein levels. Seedlings of Pro35S:BAM1-GFP were grown on 1/2 MS medium with or without 1% sucrose under different photoperiods (A) or different light intensity (B) for 10 days. C, AZD8055 treatment inhibited sucrose-induced BAM1 protein accumulation. Seedlings of Pro35S:BAM1-GFP were grown on 1/2 MS medium with or without 1% sucrose and/or 1-µM AZD8055 under a 12-h light/12-h dark photoperiod with 10 µM m−2 s−1 light or a 4-h light/20-h dark photoperiod with 100-µM m−2 s−1 light conditions for 10 days. D, TOR is required for sucrose-induced BAM1 protein accumulation. Seedlings of Pro35S:BAM1-GFP/es-tor were grown on 1/2 MS medium with or without 1% sucrose and/or 10 µM estradiol under a 12-h light/12-h dark photoperiod with 10-µM m−2 s−1 light conditions for 10 days or under 4-h light/20-h dark photoperiod with 100-µM m−2 s−1 light conditions for 10 days. E and F, Immunoblot analysis of the effects of MG132 and 3-MA on AZD8055-reduced BAM1 protein stability. Seedlings of Pro35S:BAM1-GFP were grown on 1/2 MS liquid medium with 1% sucrose under a 16-h light/8-h dark photoperiod with 100-µM m−2 s−1 light for 7 days, then treated with 2 μM AZD8055, or 2 μM AZD8055 and 5 mM 3-MA or 50-μM MG132 for 6 h. The ratio of immunoprecipitated BAM1-YFP to actin was quantified Figure 5 (Continued) by ImageJ software. Error bars indicate sd of three or four biological repeats. Actin bands were used as loading control. Different letters above bars indicate statistically significant differences between samples (one-way ANOVA followed by uncorrected Fisher’s LSD multiple comparisons test, P < 0.05). G, BAM1 gene expression slightly decreases after 1-h light exposure in the dawn. Seedlings of ProBAM1::GFP transgenic plants were grown on the 1/2 MS medium with 1% sucrose under a 12-h light/12-h dark photoperiod with 100-µM m−2 s−1 light condition for 10 days. The confocal image of ProBAM1::GFP was captured at the EoN and after 1-h light illumination. Asterisk above the bars indicate statistically significant differences between samples (Student’s t test, *P < 0.05). H, BAM1 protein abundant dramatically increase after 1-h light exposure in the dawn is dependent on TOR kinase. Seedlings of ProBAM1::BAM1-YFP transgenic plants were grown on the 1/2 MS medium with 1% sucrose under a 12-h light/12-h dark photoperiod with 100-µM m−2 s−1 light condition for 10 days, transferred to the medium containing mock solution or 2-µM AZD8055 to grow for 12 h before the EoN. The confocal image of ProBAM1::BAM1-YFP was captured at EoN and after 1-h light illumination. Different letters above bars indicate statistically significant differences between samples (two-way ANOVA followed by Tukey’s multiple comparisons test, P < 0.05). GFP signal intensity of >100 stomata from five seedlings was analyzed using ImageJ software. Error bars indicate sd (n = 100). GFP are in green, PI-marked cell outlines are in purple. Scale bars in confocal images represent 50 μm. I, TOR kinase activity increases after 1-h light exposure in the dawn. Seedlings of Pro35S::S6K1-HA transgenic plants were grown on the 1/2 MS medium with 1% sucrose under a 12-h light/12-h dark photoperiod with 100-µM m−2 s−1 light condition for 10 days. Samples were harvested at EoN and after 1-h light illumination using for immunoblot analysis. The ratio of S6K1-pT449 and S6K1-HA were analyzed by ImageJ software. Error bars indicate sd (n = 3). Asterisk above the bars indicates statistically significant differences between samples (Student’s t test, *P < 0.05). Actin bands were used as loading control.
To determine whether TOR is involved in the sugar-induced accumulation of BAM1, we analyzed BAM1-YFP protein levels in response to AZD8055 and found that AZD8055 treatment reduced protein levels (Figure 5C). Consistent with this result, sucrose failed to induce BAM1 accumulation in es-tor mutants (Figure 5D). Considering that protein degradation relies on proteasome or autophagy pathways in eukaryotic cells, we investigated the effects of the autophagy inhibitor 3-methyladenine (3-MA; Takatsuka et al., 2004) and the proteasome inhibitor MG132 on the stability of BAM1. The 3-MA and MG132 treatments prevented the AZD8055-induced degradation of BAM1 protein (Figure 5, E and F). Furthermore, the 3-MA and MG132 treatments partially suppressed the undegradable guard cell starch and impaired stomatal opening phenotypes of es-tor mutants, and such effects were enhanced in the bzr1-1D/es-tor mutants (Figure 5, G and H). These results indicated that sugar starvation and TOR inactivation caused BAM1 degradation via autophagy and proteasome pathways.
Given that the critical roles of the TOR-BAM1 module in the rapid guard cell starch degradation within 1 h of light exposure, we analyzed the dynamic changes of BAM1 expression and BAM1 protein and TOR activity during this process. After 1 h of light exposure, the expression levels of BAM1 were slightly decreased, but the protein levels of BAM1 and the phosphorylated S6K1 were significantly increased (Figure 5, G–I). Furthermore, the light-induced accumulation of BAM1 protein was significantly inhibited by AZD8055 treatment (Figure 5H). These results suggest that light exposure increases TOR activity and promotes BAM1 protein accumulation, thereby inducing guard cell starch degradation.
Phospho-mimicking mutation at Ser-31 increases BAM1 protein stability
A recent phosphoproteomic study showed that sucrose treatment weakly increased the phosphorylated modification of BAM1 at Ser-31, whereas AZD8055 treatment dramatically inhibited the Ser-31phosphorylation of BAM1 (Van Leene et al., 2019). Consistent with this, AZD8055 treatment significantly reduced the phosphorylation of BAM1 (Supplemental Figure S15, A and B). To investigate whether TOR directly phosphorylates BAM1 in plants, we examined the interaction between TOR and BAM1 using Co-immunoprecipitation experiments. Our results showed that TOR did not interact with BAM1 under our experimental conditions, suggesting that TOR promotes the phosphorylation of BAM1 by regulating the activity of other kinases (Supplemental Figure S16).
To determine the effects of TOR-regulated phosphorylation on the function of BAM1, we generated transgenic plants with the phospho-mimicking mutation BAM1S31D or phospho-null mutation BAM1S31A driven by the BAM1 native promoter in the bam1-1 mutant background (Supplemental Figure S17, A and B). The ProBAM1:BAM1-YFP/bam1-1 and ProBAM1:BAM1S31D-YFP/bam1-1 constructs rescued the stomata-opening phenotype of the bam1-1 mutant, but ProBAM1:BAM1S31A-YFP/bam1-1 displayed the similar phenotype to that of bam1-1 mutant (Supplemental Figure S17D). The guard cell starch content in the ProBAM1:BAM1S31D-YFP/bam1-1 was much lower than those in wild-type plants and the bam1-1 mutant and the guard cell starch degraded rapidly upon light exposure (Figure 6, A and B; Supplemental Figure 15C). Although Ser-31 is localized on the chloroplast transit domain of BAM1, Ser-31 mutations to Alanine or Glutamate did not change the subcellular localization of BAM1 in the chloroplast (Supplemental Figure S18, A and B). Immunoblot analysis revealed that AZD8055 treatment significantly reduced the accumulation of BAM1-YFP and BAM1S31A-YFP protein, but had weaker effects on the protein levels of BAM1S31D-YFP (Figure 6C). These results indicated that phosphorylation on Ser-31 increased the protein stability of BAM1.
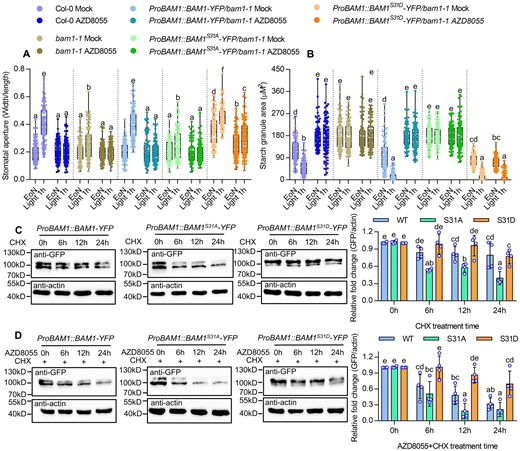
Phospho-mimicking mutation at Ser-31 increased BAM1 protein stability. A and B, Quantification of guard cell starch granules (A) and stomatal apertures (B) in the intact leaves of Col-0, bam1-1, ProBAM1::BAM1-YFP/bam1-1, ProBAM1::BAM1S31A-YFP/bam1-1, and ProBAM1::BAM1S31D-YFP/bam1-1 at the indicated time points. Seedlings of wild-type and indicated plants were grown on the 1/2 MS medium containing 1% sucrose under a 12-h light/12-h dark photoperiod and 100-µM m−2 s−1 light condition for 10 days, transferred to the medium containing mock solution or 2-µM AZD8055 to grow for 24 h, and then harvested at the indicated time points and measured the starch granules and stomatal apertures. The starch granules in guard cells (A) or the ratio of stomatal aperture width to length (B) from at least 100 guard cells from 10 to 15 leaves of 8 different plants were measured using ImageJ software. Every measurement of stomatal starch granule area or stomatal aperture was labeled as a dot on box plot. The box extends from the 25th to 75th percentiles and plots each individual value as a point superimposed on the graph. The line in the middle of the box is plotted at the median. Different letters above bars indicate statistically significant differences between samples (two-way ANOVA followed by Tukey’s multiple comparisons test, P < 0.05). C, Immunoblot analysis of the effects of cycloheximide (CHX) on the protein levels of BAM1-YFP, BAM1S31A-YFP, and BAM1S31D-YFP using anti-GFP antibody. Seedlings of ProBAM1::BAM1-YFP, ProBAM1::BAM1S31A-YFP/bam1-1, and ProBAM1::BAM1S31D-YFP/bam1-1 were grown on the 1/2 liquid MS medium containing 1% sucrose under a 16-h light/8-h dark photoperiod with 100-µM m−2 s−1 light condition for 7 days, and then 50-µm CHX for different times. D, Immunoblot analysis of the effects of AZD8055 on the protein levels of BAM1-YFP, BAM1S31A-YFP, and BAM1S31D-YFP using anti-GFP antibody. Seedlings of ProBAM1::BAM1-YFP, ProBAM1::BAM1S31A-YFP/bam1-1, and ProBAM1::BAM1S31D-YFP/bam1-1 were grown on the 1/2 liquid MS medium containing 1% sucrose under a 16-h /8-h dark photoperiod with 100-µM m−2 s−1 light condition for 7 days, and then 50-µm CHX and 2-µm AZD8055 for different times. The ratio of immunoprecipitated BAM1-YFP to actin was quantified by ImageJ software. Error bars indicate sd of four biological repeats. Actin bands were used as loading control. Different letters above bars indicate statistically significant differences between samples (two-way ANOVA followed by uncorrected Fisher’s LSD multiple comparisons test, P < 0.05).
Discussion
TOR promotes guard cell starch degradation by activating BAM1 at both the transcriptional and posttranscriptional levels
The timing and extent of starch degradation are regulated by diverse developmental and environmental signals (Zeeman et al., 2010; Streb and Zeeman, 2012). In this study, our genetic and biochemical analysis elucidated the critical roles of sugar and TOR in regulating guard cell starch metabolism and stomatal movement. Sugar treatment significantly induced the starch accumulation in guard cells and promoted its breakdown upon light exposure, inducing the stomatal opening under certain carbon starvation conditions caused by the short photoperiod or low light intensity. The promotion of stomatal opening by sugar is modulated by the conserved nutrient sensor TOR kinase. TOR inactivation via RNA interference or AZD8055 treatment resulted in failures to degrade guard cell starch and open stomata after light exposure.
BAM1 is preferentially expressed in guard cells, where it catalyzes the degradation of guard cell starch. Mutation of BAM1 leads to guard cell starch undegradable and an impaired stomatal opening phenotype upon light exposure (Horrer et al., 2016). Here, we demonstrated that sugar and TOR regulate BAM1 activity at the transcriptional and posttranscriptional levels. Sugar and TOR substantially induced BAM1 expression, whereas the TOR kinase inhibitor AZD8055 treatment reduced BAM1 expression. The gain-of-function mutant bzr1-1D partially suppressed the inhibiting effects of AZD8055 on the expression of BAM1, indicating that BZR1 plays an important role in the sugar- and TOR-induced expression of BAM1. At the posttranscriptional level, sugar and TOR induced BAM1 protein accumulation, and AZD8055 treatment promoted BAM1 degradation via autophagy and proteasome pathways. BAM1 is phosphorylated at Ser-31 by the TOR kinase pathway (Van Leene et al., 2019). We conducted a phospho-mutant activity assay, revealing that the phospho-null mutation BAM1S31A failed to rescue the guard cell-specific starch excess phenotype of the bam1-1 mutant due to instability of BAM1S31A protein. These results suggest that sugar and TOR increase BAM1 protein stability by promoting its phosphorylation. Together, these findings demonstrate that sugar and TOR promote guard cell starch degradation by increasing BAM1 activity, and the sugar produced by starch degradation can activate TOR, thus forming a positive feedback loop that regulates guard cell metabolism and stomatal movement (Figure 7).
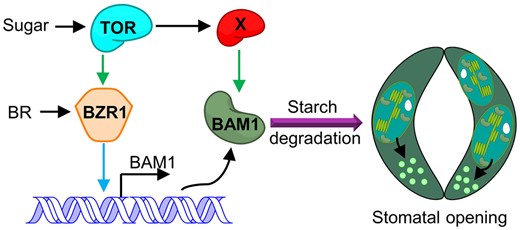
Working model for TOR-regulated guard cell starch degradation in plants. The glucan hydrolase encoded by BAM1 is preferentially expressed in guard cells to degrade guard cell starch. Sugar and TOR promote the expression of BAM1. BR and BZR1 play critical roles in the sugar-promoted expression of BAM1. Sugar supply induces BAM1 protein accumulation, but TOR inactivation leads to BAM1 degradation, and these effects are abolished by the inhibition of autophagy and proteasome pathways. TOR stabilizes BAM1 protein by promoting phosphorylation of BAM1 at Ser-31through an unknown kinase. Multiple mechanisms allow for fine-tuning the activity of BAM1 by hormonal and nutrient signals for accurate regulation of guard cell starch breakdown and stomatal movement. Blue line indicates regulation at the transcriptional level; green line indicates regulation at the posttranscriptional level.
Guard cell starch degradation mediated by the TOR-BAM1 module may be evolutionarily conserved in different plant species
The phenomenon by which starch degradation in guard cells promotes stomatal opening upon light exposure in the dawn widely exists in different species (Outlaw and Manchester, 1979; Vavasseur and Raghavendra, 2005). A recent study in Arabidopsis showed that BAM1 and AMY3, which are preferentially expressed in guard cells, promote stomatal opening by inducing guard cell starch degradation (Horrer et al., 2016). The steroid hormone BR and redox signal H2O2 induce the expression of BAM1 to promote guard cell starch degradation and stomatal opening through the transcription factors BZR1 and GBF2 (Li et al., 2020). Here, we showed that BAM1 protein quickly accumulated in guard cells upon light exposure in the dawn, which is dependent on TOR kinase activity. Activated TOR by the glucose derived from guard cell starch degradation induces the phosphorylation of BAM1 at Ser-31, which enhances BAM1 protein stability, thereby promoting guard cell starch degradation. In the Arabidopsis genome, there are nine BAM isoforms. At least four of them (BAM1–BAM4) are localized in the chloroplast (Fulton et al., 2008). Only BAM1 and BAM3 are believed to be catalytically active and catalyze glucan substrates in vitro (Monroe et al., 2014). BAM1 and BAM3 display high levels of amino acid sequence identity, but BAM3 does not contain the TOR-dependent phosphosite, suggesting that BAM1 and BAM3 have different responses to sugar-TOR-mediated signals. In addition, we found that BAM1 is highly conserved in plants. Remarkably, the Ser-31 residue is present in flowering plants, including soybean, maize, and rice. These results suggest that the TOR-BAM1 module may have the conserved function of promoting guard cell starch degradation and inducing stomatal opening in the higher plant species.
Glucose promotes stomatal opening at low contents, but induces stomatal closure at high contents
A recent study showed that guard cell starch degradation produces glucose for rapid stomatal opening (Flutsch et al., 2020), but whether glucose promotes stomatal opening and the underlying mechanism were not reported. In this study, we found that sugar supply significantly promoted the stomatal opening under certain carbon starvation conditions, such as low light quantity or very short photoperiod. Under normal light conditions or photoperiods with >12-h light, exogenous sugar supplementation did not affect stomatal opening, suggesting that the sugar produced by photosynthesis of plants is sufficient for promoting stomatal opening. We also found that TOR inactivation under all growth conditions prevented stomatal opening upon light exposure. These results indicated that glucose produced by guard cell starch degradation not only provides energy for ion transport across the plasma membrane, but may also activate TOR to promote stomatal opening.
Glucose promotes stomatal closure in a dose-dependent manner (Li et al., 2016, 2018). Stomatal closure was induced by 1-mM glucose, and the maximum promoting effect was achieved with 100-mM glucose (Li et al., 2018). Hexokinase 1 (HXK1) is a sugar-phosphorylating enzyme that acts as a glucose sensor, coordinating light, nutrient, and hormone signaling networks to regulate plant growth and development (Moore et al., 2003). The loss-of-function HXK1 mutant gin2-1 exhibited impaired stomatal closure in response to glucose treatment, indicating that sugar-induced stomatal closure is independent of its osmotic effect, but dependent on the HXK1-mediated signal transduction pathway (Li et al., 2018). However, in this study, we found that sugar and TOR play important roles in stomatal opening. The reason for these opposing effects of sugar on stomatal movement remains unclear. One possibility is that sugar regulates the stomatal movement in a dose-dependent manner. According to published results, wild-type plants produced ∼0.3-mM glucose via guard cell starch degradation to promote stomatal opening after light exposure, whereas bam1-1amy3-1 mutants produced ∼0.05-mM glucose and failed to promote stomatal opening, suggesting that the optimal glucose concentration for stomatal opening is ∼0.3 mM (Flutsch et al., 2020). However, the optimal glucose concentration for stomatal closure is ∼100 mM. Thus, sugar might promote stomatal opening via the TOR signaling pathway at low concentrations and induce stomatal closure via HXK1 signal transduction at high concentrations. The contrasting effects of sugar on stomatal movement enable plants to fine-tune stomatal apertures and photosynthesis in response to different nutritional conditions.
Photosynthetic sugar optimizes stomatal movement
Plant photosynthesis rates are closely correlated to stomatal conductance, which is coordinated with stomatal apertures over a range of CO2 concentrations and light intensities (Buckley and Matt, 2013; Santelia and Lawson, 2016). In this study, we found that plants grown under high photon irradiance had larger stomatal apertures than those grown under low photon irradiance. Plants grown under a 16-h light/8-h dark photoperiod also displayed increased stomatal apertures compared to plants grown under a 4-h light/20-h dark photoperiod. TOR inactivation inhibited stomatal opening and reduced stomatal conductance. Exogenous sucrose supply significantly increased the stomatal apertures of plants grown under low photon irradiance or short photoperiod conditions, suggesting that the decreased stomatal apertures observed under those conditions may be attributed to insufficient sugar production via photosynthesis. However, an excessive sugar concentration induced stomatal closure, reducing stomatal conductance, and inhibiting photosynthesis (Li et al., 2018). In conclusion, when plants encounter sunshine after consistent cloudy weather, photosynthesis-derived sugar activates TOR to promote stomatal opening, which in turn enhances photosynthesis; whereas under continuous sunny weather, sugar accumulated to high levels by photosynthesis and induced stomatal closure through the HXK1 pathway. Photosynthesis, sugar, and stomatal movement form a feedback loop that finely regulates plant photosynthesis in response to changing environmental conditions.
Material and methods
Plant materials and growth conditions
The Arabidopsis Col-0 ecotype was used as the wild-type plant for all chemical treatment or comparisons with transgenic plants and mutants. The T-DNA insertion knockout mutants bam1-1 (Salk_039895) were obtained from the Arabidopsis Biological Resource Center. es-tor, es-tor/bzr1-1D, det2-1, bzr1-1D, bri1-116, ProBAM1:GFP, and ProBAM1:GFP/bzr1-1D plant used in this study were described previously (Li et al., 1996; Li and Chory, 1997; Wang et al., 2002; Zhang et al., 2016; Li et al., 2020). Arabidopsis plants were grown in a growth chamber with light-emitting diode light (Ningbo Jiangnan Instruments, RXZ, light wavelength: 410–780 nm) for different photoperiod and light intensity conditions with a relative humidity of 50%.
Plasmid constructs and transgenic plants
Full-length cDNA of BAM1 without a stop codon was amplified by polymerase chain reaction (PCR) and cloned into pENTR/SD/D-TOPO vectors (Thermo Fisher Scientific, Waltham, MA, USA), and then recombined with destination vector pX-YFP (Pro35S:X-YFP). The promoters and genomic DNA of BAM1 were amplified by PCR and cloned into pENTR/SD/D-TOPO vectors (Thermo Fisher), and then recombined with destination vector pEG-TW1 (Native promoter:YFP) to generate ProBAM1:BAM1-YFP. The constructs of BAM1S31A and BAM1S31D were generated using the quick-change site-directed mutagenesis kit (Stratagene, San Diego, CA, USA). Oligo primers used for cloning are listed in Supplemental Table S1. All binary vector constructs were introduced into Agrobacterium tumefaciens (strain GV3101) and transformed into Col-0 plants or the bam1-1 mutant by the floral dipping method (Clough and Bent, 1998).
Guard cell starch quantification
The quantification of starch in guard cells followed a previously described method (Horrer et al., 2016; Li et al., 2020). Briefly, epidermal peels of the fifth or sixth rosette leaf or cotyledon of wild-type plants grown in different conditions were harvested at the indicated time points and immediately soaked in fixation solution (50% v/v methanol and 10% v/v acetic acid) for at least 24 h, at 4°C. Samples were first de-stained by incubation in 80% ethanol (v/v) at 65°C for 5 min. After removing the ethanol, the leaf or cotyledon was washed with water. Starch granules were stained with a modified pseudo-Schiff propidium iodide (PI) staining method (Truernit et al., 2008). The stained leaf or cotyledon was fixed in Hoyers solution (30 g gum Arabic, 200 g chloral hydrate, 20 g glycerol, and 50 mL water) after incubation in chloral hydrate solution (4 g chloral hydrate, 1 mL glycerol, and 2 mL water) for 1 h. The samples were observed using an LSM700 laser scanning confocal microscope system (Zeiss, Oberkochen, Germany). The excitation wavelength was set at 488 nm and the emission was collected between 610 nm and 640 nm.
Stomatal aperture measurement
Stomatal apertures were measured as described in our previous study (Li et al., 2020). Intact leaves were used for stomatal aperture measurement, detached leaves were used for Fusicoccin treatment experiment. For determining stomatal aperture in intact leaves, the “Tape-Arabidopsis Sandwich” method (Ibata et al., 2013; Lawrence et al., 2018) was applied. In this method, the leaves of the wild-type and different mutants were harvested at the indicated time points and partitioned between two portions of transparent Scotch tape, with one piece adhering to the abaxial side and the other piece adhering to the adaxial side of the leaves. The abaxial side with pavement and guard cells was gently peeled apart and placed on a glass microscope slide. Images of multiple stomata were acquired at 40× magnification. At least 100 guard cells from 10 to 15 leaves of eight different plants were measured using ImageJ software.
RT-qPCR analysis
Total RNA was extracted from 10-day-old Arabidopsis seedlings of wild-type, det2-1, and transgenic plants under different conditions using the Trizaol RNA extraction kit (Transgene). The first-strand cDNAs were synthesized using RevertAid reverse transcriptase (Abcam Cambridge, UK) and used as RT-qPCR templates. RT-qPCR analyses were performed on a CFX connect real-time PCR detection system (Bio-Rad, Hercules, CA, USA) using an SYBR green reagent (Roche, Basel, Switzerland) with gene-specific primers (see Supplemental Table S1).
Microscopy
Confocal microscopy was performed using an LSM-880 laser scanning confocal microscope (Zeiss). GFP fluorescent intensities in different genetic backgrounds transgenically expressing ProBAM1:GFP were quantified using ImageJ software (Han et al., 2020). Images were captured at 488 and 561 nm laser excitation and 500–530 nm and 580–660 nm for GFP and PI, respectively. Laser intensity setting was restricted to ˂5%. The master gain value was not more than 800.
Immunoblot analysis of protein abundance
Protein was extracted from 10-day-old Arabidopsis seedlings transgenically expressing Pro35S:BAM1-YFP or other BAM1-YFP constructs under different growth conditions using protein extraction buffer (25-mM Tris–HCl pH 7.6, 15-mM MgCl2, 150-mM NaCl, 60-mM β-glycerophosphate, 0.1-mM Na3VO4, 1-mM NaF, 1-mM PMSF, 1% Triton X-100, and 1 100-mL−1 protease inhibitor cocktail). After quantifying the protein concentration using Bradford solution, 60-μg total protein extract was separated by SDS–polyacrylamide gel electrophoresis. Proteins were transferred by immunoblotting, blots were blocked for 1 h in 5% skimmed milk in TBS-T, and bands were detected with mouse anti-GFP (1/5,000 dilution) (Transgene Biotech, Beijing, China; cat: HT801) or mouse anti-actin (1/5,000 dilution) (Sigma; cat: A0480) as the primary antibody and anti-mouse-HRP (1/10,000, dilution) (Beyotime Biotechnology, Shanghai, China; Cat: A0216) as the secondary antibody. BAM1-YFP protein abundance was analyzed using ImageJ software and normalized by actin relative abundance.
Accession numbers
Sequence data from this article can be found in the Arabidopsis Genome Initiative or GenBank/EMBL databases under the following accession numbers: AT1G50030 (TOR), AT5G65700 (BAM1), and AT1G75080 (BZR1).
Supplemental data
The following materials are available in the online version of this article.
Supplemental Figure S1. AZD8055 treatment inhibited guard cell starch degradation.
Supplemental Figure S2. TOR plays an important role on guard cell starch degradation.
Supplemental Figure S3. Dynamic analysis guard cell starch granules and stomatal apertures of es-tor mutants.
Supplemental Figure S4. TOR promotes guard cell starch degradation in the rosette leaves of plants.
Supplemental Figure S5. FC treatment promotes stomatal opening in the es-tor plants.
Supplemental Figure S6. Sucrose regulates guard cell starch content and stomatal apertures of 5-week-old plants grown under carbon starvation conditions.
Supplemental Figure S7. Quantitative analysis of the effects of different types of sugar on guard cell starch content and stomatal apertures.
Supplemental Figure S8. Immunoblot analysis of TOR activity in different photoperiod or light intensity.
Supplemental Figure S9. Sucrose induced, but AZD8055 reduced the fluorescence intensity of pBAM1::GFP under carbon starvation conditions.
Supplemental Figure S10. AZD8055 treatment promoted BZR1 protein degradation.
Supplemental Figure S11. The gain-of-function mutant bzr1-1D partially suppressed the AZD8055-inhibited BAM1 expression.
Supplemental Figure S12. BR signaling plays critical roles in sugar- and TOR-induced BAM1 expression
Supplemental Figure S13. Immunoblot analysis of the effects of different types of sugar on BAM1 protein.
Supplemental Figure S14. 3-MA and MG132 treatment partially suppressed the guard cell starch degradation and stomatal opening phenotypes of es-tor and es-tor/bzr1-1D mutants.
Supplemental Figure S15. AZD8055 treatment reduced the phosphorylation of BAM1.
Supplemental Figure S16. TOR does not interact with BAM1 in plants.
Supplemental Figure S17. The growth phenotypes of pBAM1::BAM1-YFP/bam1-1, pBAM1::BAM1S31A-YFP/bam1-1, and pBAM1::BAM1S31D-YFP/bam1-1 transgenic plants
Supplemental Figure S18. Mutations of Ser-31 to alanine or glutamate did not change the subcellular localization of BAM1 in plants.
Supplemental Table S1. Oligonucleotide sequences used in this study.
Supplemental Data Set S1. Statistical analysis in this study.
C.H. and M.B. designed experiments; C.H. performed stomata aperture measurement, starch quantification in guard cells, confocal analysis of GFP intensity, qRT-PCR, immunoblot analysis of protein abundant, and all other rest experiments. W.H., R.L., and J.L. provided suggestion on stomata bioassay protocol and generated Pro35S:BAM1-YFP, ProBAM1:GFP, ProBAM1:GFP/bzr1-1D, ProBAM1:BAM1-YFP, ProBAM1:BAM1S31A-YFP, and ProBAM1:BAM1S31D-YFP transgenic plants. L.Y., Y.Q., and W.H. crossed the transgenic lines to bam1-1 mutant and prepared plants for experiment. L.Y. helped to perform starch content measurement. M.F. W.Y., and G.J. provided the critical discussion on the work. C.H. and M.B. wrote the manuscript.
The author responsible for distribution of materials integral to the findings presented in this article in accordance with the policy described in the Instructions for Authors (https://dbpia.nl.go.kr/plcell) is: Ming-Yi Bai ([email protected]).
Acknowledgments
We thank Prof. Zhiyong Wang for providing es-tor and es-tor/bzr1-1D seeds. We thank Haiyan Yu, Yuyu Guo, and Xiaomin Zhao from the Analysis and Testing Center of SKLMT (State Key Laboratory of Microbial Technology, Shandong University) for assistance with the laser scanning confocal microscopy.
Funding
This work was supported by grants from the National Natural Science Foundation of China (31600199, 31670284, 31970306, and 31870262) and grants from Science and Technology Department of Shandong Province (2019LZGC015 and ZR2019ZD16).
Conflict of interest statement. The authors declare that they have no conflicts of interest.
References
Author notes
Senior author