-
PDF
- Split View
-
Views
-
Cite
Cite
He Zhao, Biao Ma, Kai-Xuan Duan, Xin-Kai Li, Xiang Lu, Cui-Cui Yin, Jian-Jun Tao, Wei Wei, Wan-Ke Zhang, Pei-Yong Xin, Sin Man Lam, Jin-Fang Chu, Guang-Hou Shui, Shou-Yi Chen, Jin-Song Zhang, The GDSL Lipase MHZ11 Modulates Ethylene Signaling in Rice Roots, The Plant Cell, Volume 32, Issue 5, May 2020, Pages 1626–1643, https://doi.org/10.1105/tpc.19.00840
- Share Icon Share
Abstract
Ethylene plays important roles in plant growth and development, but the regulation of ethylene signaling is largely unclear, especially in crops such as rice (Oryza sativa). Here, by analysis of the ethylene-insensitive mutant mao huzi 11 (mhz11), we identified the GDSL lipase MHZ11, which modulates ethylene signaling in rice roots. MHZ11 localized to the endoplasmic reticulum membrane and has acyl-hydrolyzing activity. This activity affects the homeostasis of sterols in rice roots and is required for root ethylene response. MHZ11 overexpression caused constitutive ethylene response in roots. Genetically, MHZ11 acts with the ethylene receptor ETHYLENE RESPONSE SENSOR2 (OsERS2) upstream of CONSTITUTIVE TRIPLE RESPONSE2 (OsCTR2) and ETHYLENE INSENSITIVE2 (OsEIN2). The mhz11 mutant maintains more OsCTR2 in the phosphorylated form whereas MHZ11 overexpression promotes ethylene-mediated inhibition of OsCTR2 phosphorylation. MHZ11 colocalized with the ethylene receptor OsERS2, and its effect on OsCTR2 phosphorylation requires ethylene perception and initiation of ethylene signaling. The mhz11 mutant overaccumulated sterols and blocking sterol biosynthesis partially rescued the mhz11 ethylene response, likely by reducing receptor-OsCTR2 interaction and OsCTR2 phosphorylation. We propose that MHZ11 reduces sterol levels to impair receptor-OsCTR2 interactions and OsCTR2 phosphorylation for triggering ethylene signaling. Our study reveals a mechanism by which MHZ11 participates in ethylene signaling for regulation of root growth in rice.
INTRODUCTION
Ethylene regulates many aspects of plant growth, development, and stress responses. By identification of the key components of ethylene signaling and uncovering the biochemical mechanisms, a linear ethylene signaling pathway has been established in the model plant Arabidopsis (Arabidopsis thaliana; Ju and Chang, 2015). In this pathway, the ethylene signal is perceived by a family of endoplasmic reticulum (ER) membrane-localized ethylene receptors (Chang et al., 1993; Hua et al., 1995; Chen et al., 2002) and transduced from the negative regulator CONSTITUTIVE TRIPLE RESPONSE1 (CTR1; Kieber et al., 1993) to the positive regulator ETHYLENE INSENSITIVE2 (EIN2; Alonso et al., 1999). In the absence of ethylene, the receptor proteins activate the downstream Raf-like Ser/Thr protein kinase CTR1 by interacting with the N-terminal region of CTR1, which subsequently phosphorylates the C-terminal of EIN2 to suppress ethylene signal transduction (Hua and Meyerowitz, 1998; Qiao et al., 2009; Ju et al., 2012). In the presence of ethylene, the receptors are inactivated upon ethylene binding and fail to activate the kinase activity of CTR1, resulting in the dephosphorylation of EIN2. The EIN2 C-terminal domain is further cleaved off from the ER membrane and translocated into the nucleus (Ju et al., 2012; Qiao et al., 2012; Wen et al., 2012) to activate the EIN3 (ETHYLENE INSENSITIVE3)/EIN3-LIKE1 (EIL1)-mediated transcriptional regulation cascade (Chao et al., 1997; Solano et al., 1998) by stabilizing EIN3 and EIL1 proteins (Guo and Ecker, 2003; Potuschak et al., 2003; An et al., 2010) and regulating histone acetylation (Zhang et al., 2016, 2017b). Meanwhile, the EIN2 C-terminal end can also be transferred into the cytoplasmic processing-body to bind to the 3′-UTR of the EBF1/2 mRNA for translational repression of EIN3-BINDING F-BOX PROTEIN1 (EBF1) and EBF2 to release EIN3/EIL1 functions (Li et al., 2015; Merchante et al., 2015). Although great progress has been made in understanding the biochemical function of EIN2, the regulatory mechanism upstream of EIN2, especially how CTR1 is regulated in vivo, remains largely unclear.
Semiaquatic rice (Oryza sativa) is an important monocotyledonous crop worldwide. Ethylene plays essential roles in the adaptive responses to submergence-induced hypoxia in rice and functions in the regulation of multiple agronomic traits. Using an efficient system, we have screened a set of rice ethylene response mutants that were named mao huzi (mhz, meaning “cat whiskers” based on the appearance of their roots), and identified conserved and novel components of ethylene signaling, i.e., MHZ7/OsEIN2 (Ma et al., 2013); MHZ6/OsEIL1 (Yang et al., 2015); and the previously unidentified component MHZ3, a stabilizer of OsEIN2 (Ma et al., 2018).
The GDSL-family esterases/lipases are hydrolytic enzymes widely found in bacteria and plants. This family of lipase is defined by a distinct GDSL motif GxSxxxxG with the active site Ser located near the N terminus (Upton and Buckley, 1995). With a flexible active site, this family of lipases may have broad substrate specificity (Mølgaard et al., 2000). Numerous GDSL lipases have been characterized in plants and members are involved in hormone signaling (Oh et al., 2005; Kwon et al., 2009; Lee et al., 2009; Kim et al., 2013, 2014), cuticle formation (Park et al., 2010), xylan deacetylation (Zhang et al., 2017a), and secondary metabolism (Clauss et al., 2008; Kikuta et al., 2012). The secreted GDSL family lipase AtGLIP1 is involved in ethylene signaling in regulating Arabidopsis resistance to Alternaria brassicicola (Oh et al., 2005; Kwon et al., 2009; Kim et al., 2013, 2014).
In this article, we characterized the root ethylene-insensitive mhz11 mutant. MHZ11 encodes a previously uncharacterized ER membrane GDSL-family lipase with acyl-hydrolyzing activity. MHZ11 facilitates ethylene signaling through modulating the ethylene receptor-mediated OsCTR2 phosphorylation. This function is likely achieved partially by affecting the membrane sterol homeostasis. Our findings reveal a potential mechanism by which the GDSL-type lipase MHZ11 modulates ethylene signaling through its acyl-hydrolyzing activity.
RESULTS
Phenotypic Analysis and Gene Identification of the mhz11 Mutant
Rice mhz11 was identified in a genetic screen for rice ethylene-response mutants from our ethyl methanesulfonate (EMS) mutant populations (Zhou et al., 2019). In air, dark-grown seedlings of wild type and mhz11 were similar in coleoptile and root growth. In 10 µL L-1 of ethylene treatment, root growth of wild type was drastically repressed compared with that in air, while mhz11 root growth was barely inhibited, indicating a complete ethylene-insensitive phenotype in primary roots of the mutant (Figure 1A). Coleoptile growth of mhz11 exhibits similar ethylene response with that of wild type (Figure 1A). RiceETHYLENE-RESPONSE-FACTOR002 (OsERF002), RELATED-TO-AP28 (OsRAP2.8), and ARABIDOPSIS-RESPONSE-REGULATOR5 (OsRRA5) were previously described as ethylene-responsive genes (ERGs; Ma et al., 2013; Yin et al., 2015). Ethylene-induced expression of these genes in mhz11 roots was largely abolished or hampered as compared with those of the wild type, further confirming the ethylene abnormality of mhz11 roots (Figure 1B).
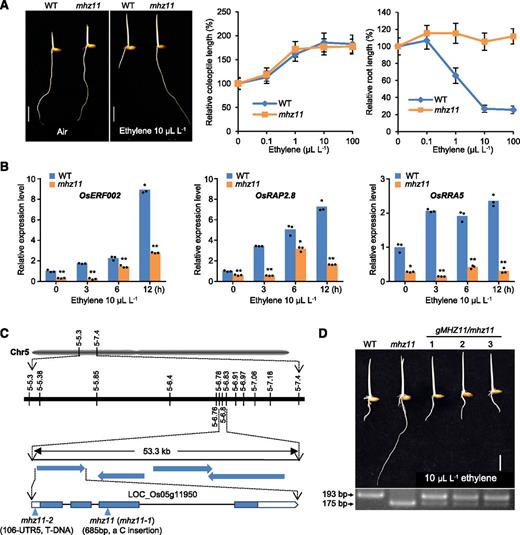
Ethylene Response Analysis and Gene Identification of mhz11.
(A) Ethylene response phenotype of mhz11 mutant. Dark-grown seedlings of the wild type (WT) and mhz11 were treated with varied concentrations of ethylene for 2.5 d. Representative seedlings grown with or without 10 µL L-1 of ethylene are shown (left). Relative coleoptile (middle) and root (right) lengths are means ± sd (n > 25) calculated from at least 25 seedlings.
(B) Ethylene-induced gene expression of OsERF002, OsRAP2.8, and OsRRA5 is abolished or hampered in mhz11 roots. Two-d–old etiolated seedlings were treated with or without 10 µL L-1 of ethylene. Data are means ± sd, n = 3 (three biological replicates, six seedlings per replicate; *P < 0.05, **P < 0.01, Student’s t test; compared with each corresponding wild-type [WT] control).
(C) Fine mapping of the MHZ11 gene. The mutation sites are indicated in schematic diagrams. mhz11 (mhz11-1) was obtained by screening our EMS-mutagenized lines. mhz11-2 is a T-DNA insertion mutant requested from the POSTECH Biotech Center (http://www.postech.ac.kr/eng/).
(D) Functional complementation of mhz11 with MHZ11 genomic DNA. Vector carrying the wild-type MHZ11 genomic DNA was transformed into the mhz11 mutant, rescuing the ethylene response of mhz11-etiolated seedlings in transgenic lines (top). The mhz11 background in the transgenic lines was confirmed by dCAPS analysis (lower representation). The fragment of mhz11 mutant was 18-bp shorter than that of wild type (WT).
Scale bars = 10 mm.
By map-based cloning and sequencing of the candidate genes within a 53.3-kb region on chromosome 5, a single C insertion was revealed in the third exon of LOC_Os05g11950 (Figure 1C), resulting in a frame-shift mutation of the gene. A construct carrying the wild-type genomic sequence of the gene (3,016-bp sequence upstream of ATG codon, 2,756-bp genomic coding sequence, and 1,578-bp sequence downstream of stop codon) was transformed into mhz11 and rescued the ethylene-insensitive phenotype of mhz11, demonstrating that MHZ11 is LOC_Os05g11950. The mhz11 background of the transgenic lines was confirmed by derived cleaved amplified polymorphic sequence analysis (dCAPS; Figure 1D).
A rice mutant PFG_1A-21225.R from the POSTECH Biotech Center (Yi and An, 2013) was further identified and named mhz11-2, following the original mutant mhz11-1 (mhz11 for simplicity). This mhz11-2 had a T-DNA insertion 106-bp upstream of the ATG codon of LOC_Os05g11950 and showed hardly detectable LOC_Os05g11950 transcripts compared with wild type. mhz11-2 exhibited similar ethylene responses as mhz11 (Supplemental Figure 1A). This further confirmed that alteration of LOC_Os05g11950 is responsible for the mhz11 phenotype.
MHZ11 Encodes a Putative ER Membrane-Integrated GDSL Lipase
Examination of sequence alignment suggested that MHZ11 encodes a putative GDSL lipase (Supplemental Figure 1B). Conserved residues Ser, Gly, Asn, and His in four conserved blocks I, II, III, and V were found within MHZ11 (Supplemental Figure 1C), suggesting it belongs to the SGNH subgroup hydrolases, which usually have broad substrate specificity due to their flexible active sites (Mølgaard et al., 2000). MHZ11 is predicted to be a type-II membrane protein by the server program TMHMM 2.0 (http://www.cbs.dtu.dk/services/TMHMM-2.0/; Supplemental Figure 1D). To determine whether MHZ11 is membrane-localized, MHZ11-FLAG/mhz11 transgenic lines were generated by transforming the ProMHZ11:MHZ11-FLAG construct into mhz11. These transgenic lines exhibited similar ethylene responses as the wild type, indicating that the expressed MHZ11-FLAG proteins are functional (Figure 2A). Total proteins of roots were subsequently extracted and separated into soluble and microsomal fractions by ultracentrifugation. A small amount of MHZ11-FLAG protein was detected in the cytosol (the supernatant), but MHZ11-FLAG protein was mainly detected in the microsomal fraction, indicating that MHZ11 is a membrane-associated protein (Figure 2B). To further determine the nature of the membrane association, microsomes extracted from MHZ11-FLAG/mhz11 transgenic lines were further subjected to various extractions. Results showed that compared with the Triton X-100 or SDS treatments extracting the membrane protein MHZ11 (Figure 2C, lanes 5 to 8), neither high salt nor alkali treatments, which are effective in extracting most peripherally associated proteins, were able to remove MHZ11-FLAG from the membranes (Figure 2C, lanes 1 to 4), indicating that MHZ11 is integrated into the membrane rather than peripherally associated with it.
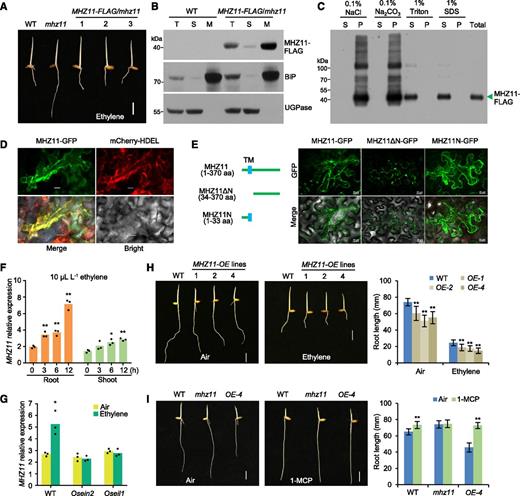
MHZ11 Is Localized to the ER Membrane and Positively Regulates Ethylene Signaling.
(A) MHZ11-FLAG protein rescued the ethylene-insensitive phenotype of mhz11 roots. Two-kb promoter of MHZ11 was cloned to drive the MHZ11-FLAG. Vector carrying ProMHZ11:MHZ11-FLAG was transformed into mhz11 and ethylene responses of the homozygous lines, and checked. Dark-grown seedlings were treated with 10 µL L-1 of ethylene. Scale bar = 10 mm.
(B) Membrane association of MHZ11. Equal amounts of total protein (T), soluble protein (S), and microsomal membranes (M) were immunoblotted for MHZ11-FLAG, BiP (ER membrane marker), and UGPase (cytoplasm marker).
(C) MHZ11 is an integral membrane protein. Microsomes were prepared from roots of MHZ11-FLAG/mhz11 transgenic plants. The membrane pellet was resuspended in 0.1 M of NaCl, 0.1 of M Na2CO3 at pH 11, and 1% (v/v) Triton X-100 or 1% (w/v) SDS. Samples were separated into supernatant (S) and pellet (P) fractions by centrifugation at 125,000g for 1 h. Equal amounts of proteins were immunoblotted for MHZ11-FLAG.
(D) ER membrane localization of MHZ11 protein as revealed by transient expression of MHZ11-GFP in N. benthamiana leaf epidermal cells. mCherry-HDEL served as ER marker. Scale bars = 20 μm. Five randomly chosen regions of interest of infiltrated leaves were observed with similar results.
(E) Localization of different truncated versions of MHZ11 protein. TM, transmembrane motif of MHZ11. Scale bars = 20 μm. Five randomly chosen regions of interest of infiltrated leaves in each group were observed with similar results.
(F) Gene expression of MHZ11 is induced by ethylene as revealed by qPCR. Data are means ± sd, n = 3, six seedlings per replicate (*P < 0.05, **P < 0.01, Student’s t test; each compared with the corresponding 0-h control). Two-d–old dark-grown seedlings were treated with 10 µL L-1 of ethylene for various time lengths before being collected for RNA extraction.
(G) Ethylene induction of MHZ11 is abolished in Osein2 and Oseil1 mutants as revealed by qPCR. Two-d–old dark-grown seedlings were treated with 10 µL L-1 of ethylene for 6 h. Data are means ± sd, n = 3, six seedlings per replicate (*P < 0.05, Student’s t test; compared with the corresponding air control).
(H) Constitutive short root phenotype of MHZ11-OE lines. Vector carrying Pro35S:MHZ11-FLAG was transformed into the mhz11 mutant and three transgenic lines overexpressing MHZ11 were used for phenotype analysis. Dark-grown seedlings were treated with 10 µL L-1 of ethylene for 2.5 d. Root lengths are means ± sd (n > 30) calculated from at least 30 seedlings (**P < 0.01, Student’s t test; compared with the wild type [WT]).
(I) 1-MCP suppressed the short root phenotype of MHZ11-OE. Dark-grown seedlings were treated with or without 10 µL L-1 of 1-MCP for 2 d. Scale bars = 10 mm. Root lengths are means ± sd (n > 30) calculated from at least 30 seedlings (**P < 0.01, Student’s t test; each compared with the corresponding air control).
MHZ11-GFP protein was further transiently expressed in epidermal cells of Nicotiana benthamiana leaves and GFP fluorescence was mainly detected in a reticular network-like structure labeled by the ER marker protein mCherry-HDEL, suggesting that MHZ11-GFP is mainly localized to the ER membrane (Figure 2D). The localization of different truncated versions of MHZ11 fused with GFP revealed that MHZ11 is bound to the ER membrane through its N-terminal transmembrane domain (Figure 2E). Because the N-terminal hydrophobic sequences in some GDSL family members may function as a signal peptide for secretion of the protein (Oh et al., 2005; Lee et al., 2009), the possibility that MHZ11 may be secreted to the intercellular space was further tested. N. benthamiana leaf cells expressing MHZ11-YFP were treated with 30% Suc for plasmolysis, and the yellow fluorescent protein (YFP) fluorescence was barely detected in the intercellular space, suggesting that MHZ11 is an intracellular protein (Supplemental Figure 2A). All these results support the prediction that MHZ11 is an integral ER membrane protein.
MHZ11 Expression Is Induced by Ethylene, and Its Overexpression Confers Constitutive Ethylene Response
MHZ11 transcripts were induced by ethylene in both roots and shoots of wild-type–etiolated seedlings, and the induction in roots was stronger than in shoots (Figure 2F). Promoter-GUS analysis further confirmed the ethylene induction of MHZ11 (Supplemental Figure 2B) in roots and coleoptiles of etiolated seedlings. The induction is abolished in the ethylene signaling mutants mhz7/Osein2 and mhz6/Oseil1 (Figure 2G), suggesting that ethylene induced MHZ11 expression through the canonical ethylene signaling pathway.
MHZ11 fused with the FLAG tag driven by the 35S promoter was constructed in plant expression vector and transformed into mhz11 to study gene function. Three lines with high MHZ11 expression (MHZ11-OE lines) were selected for phenotype analysis (Figure 2H, Supplemental Figure 2C). Compared with wild type, the OE lines exhibited significantly shorter roots when grown in the air or upon ethylene treatment (Figure 2H). Treatment with the ethylene perception inhibitor 1-methylcyclopropene (1-MCP) suppressed the short root phenotype of MHZ11-OE lines (Figure 2I), suggesting that the constitutive short root phenotype was caused by enhanced ethylene response and MHZ11 function requires ethylene signal perception. Because the ethylene production in the OE lines was not enhanced compared with wild type (Supplemental Figure 2D), it is likely that the short root phenotype in the MHZ11-OE lines was due to an enhanced ethylene signaling. These results suggest that MHZ11 facilitates ethylene signal transduction in rice roots.
MHZ11 Functions through Its Acyl-Hydrolyzing Activity to Modulate Lipid Metabolism and Ethylene Response
As MHZ11 encodes a putative SGNH subfamily hydrolase that may have broad substrate specificity, a structure-based prediction of its specific substrates would be difficult. Therefore, we applied a lipidomics approach to study the possible substrates for MHZ11 in vivo. By analyzing the lipidomics data of the wild type, mhz11, and the overexpression line OE-4, a variety of lipids related to MHZ11 were identified and divided into three groups: (1) Phospholipids including phosphatidylcholines (PCs), phosphatidylethanolamines (PEs), phosphatidylserines (PSs), and phosphatidylglycerols (PGs) etc.; (2) Triacylglycerols (TAGs) and diacylglycerols (DAGs); and (3) Free phytosterols and phytosteryl esters (Figures 3A to 3C; Supplemental Data Set 1). Under air treatment, the PS, DAG, and free phytosterol levels in mhz11 were significantly higher than those in the wild type. PC, PE, and PG contents in mhz11 were also slightly higher than the wild type (Figures 3A and 3C). Meanwhile, TAG and phytosteryl ester contents in mhz11 were lower than those in wild type (Figures 3B and 3C). In the OE-4 line, PC, PE, PS, PG, and free sitosterol contents were lower than those in the wild type (Figures 3A and 3C), while TAG, sitosteryl ester, and campesteryl ester levels were higher than the wild type (Figures 3B and 3C). Under ethylene treatment, levels of PC, PE, TAG, and steryl esters in all the wild type, mhz11, and OE-4 lines were enhanced compared with the corresponding values in air (Figures 3A to 3C). The pattern changes of lipid species in mhz11 and OE-4 compared with the wild type after ethylene treatment are similar to that in these plants without treatment. In ethylene-treated mhz11, contents of PC, PE, PS, PG, DAG, and free sterols are higher than those in the wild type, while contents of steryl esters are lower (Figures 3A to 3C). In ethylene-treated OE-4 line, PC, PE, PS and DAG levels are lower than those in the wild type, while TAG and campesteryl ester levels are higher (Figures 3A to 3C). From these results, we propose that MHZ11 may participate in the hydrolysis of phospholipids like PC, PE, PS, and PG, etc. to produce free fatty acids (FFAs), which were subsequently supplied for the biosynthesis of TAG and phytosteryl esters using DAG and free phytosterols, respectively, as substrates, possibly with the help of other relevant enzymes.
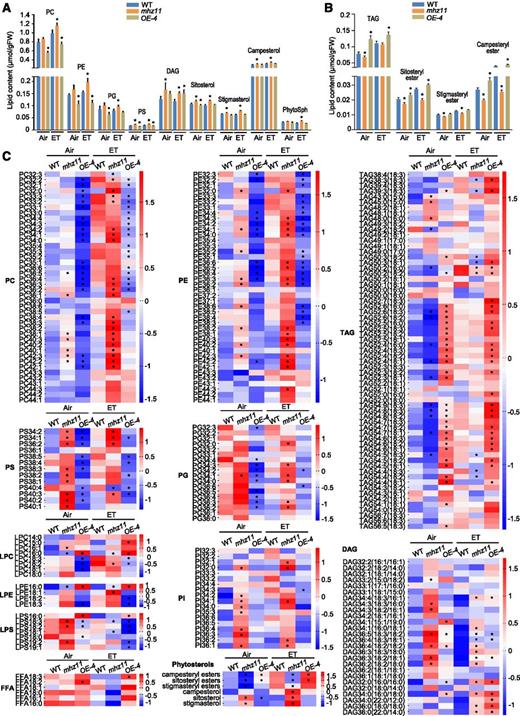
MHZ11 Modulates Lipid Metabolism in Rice Roots.
(A) and (B) MHZ11-related lipids in roots of the wild type (WT), mhz11 mutant, and MHZ11OE-4 transgenic line. Roots of 3-d–old etiolated seedlings treated with or without 10 µL L-1 of ethylene for 6 h were collected and weighed precisely. Polar lipids were analyzed using a model no. 1260 HPLC system (Agilent Technologies) coupled with a triple quadrupole/ion trap mass spectrometer (QTRAP 5500). Data are means ± sd from four biological replicates (10 seedlings per replicate). Student’s t test indicates a significant difference compared with the corresponding WT control (*P < 0.05).
(C) Heatmaps of MHZ11-related lipids in the wild-type (WT), mhz11 mutant, and MHZ11 transgenic overexpression line OE-4 according to the lipid profile analysis. Bars indicate the Z-score. ET, ethylene; PI, phosphatidylinositols; LPS, lyso-PS; LPC, lyso-PC; LPE, lyso-PE. Student’s t test indicates a significant difference compared with the corresponding WT control (*P < 0.05).
FFAs and lyso-phospholipids are the hydrolysis products of phospholipids (Ryu, 2004). In our lipid profile data, several FFA species (FFA18:3, FFA18:1, and FFA16:1) showed increases in MHZ11-OE plants but decreases in mhz11 in air and/or ethylene (Figure 3C). Regarding lyso-phospholipid species, while several (LPC16:0, LPE16:0, and LPS16:0) are roughly positively associated with MHZ11, some other species exhibit opposite trends or are not closely associated with MHZ11 (Figure 3C).
The ability of MHZ11 to hydrolyze PC was tested. MHZ11-FLAG protein from roots of the Pro35S:MHZ11-FLAG/mhz11 transgenic plants were purified and tested for phospholipase A (PLA) activity. The MHZ11 protein exhibits strong PLA2 activity in the in vitro assay (Figure 4A). By contrast, the purified MHZ11(S39A)-FLAG protein, with a mutation at the active site Ser, barely had any enzyme activity (Figure 4A). These results indicate that MHZ11 may function as a hydrolase with PLA2 activity and hydrolyze phospholipids to release FFAs for subsequent ester biosynthesis. Considering that a variety of lipid species were altered by the MHZ11 mutation and overexpression (Figures 3A to 3C; Supplemental Data Set 1), it is also possible that MHZ11 may have an acyl-hydrolyzing activity with broad substrate specificity.
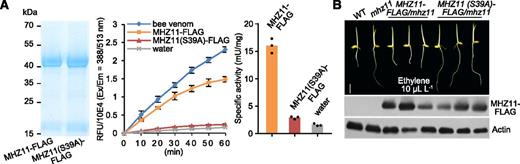
MHZ11 has PLA2 Activity and the Enzyme Activity Is Essential for Its Function.
(A) PLA2 activity of MHZ11. (Left) MHZ11-FLAG and MHZ11(S39A)-FLAG proteins were purified from transgenic plants expressing MHZ11-FLAG and MHZ11(S39A)-FLAG, respectively. (Middle) PLA2 activity of MHZ11-FLAG was tested. Proteins were incubated with a synthetic thiophospholipid at 37°C, producing a lysothiophospholipid that reacts with a fluorogenic probe to produce a fluorescent product detectable in the visible range (Excitation = 388 nm; Emission = 513 nm). Bee venom (provided in the PLA2 activity assay kit, BioVision) and water served as positive and negative controls, respectively. Data are means ± sd calculated from three replicates. (Right) The specific activity of MHZ11-FLAG and MHZ11(S39A)-FLAG was calculated. One unit of PLA2 is the amount of enzyme that generates 1 μmole of lysothiophospholipid metabolite per min at pH7.5 at 37°C. RFU, relative fluorescence units.
(B) Ethylene responses of MHZ11(S39A)-FLAG/mhz11 transgenic lines. Seedlings were grown in dark with 10 µL L-1 of ethylene for 2.5 d. Expression of MHZ11-FLAG and MHZ11(S39A)-FLAG protein in the transgenic lines were tested using anti-FLAG antibody. Actin served as internal reference. Scale bar = 10 mm. WT, wild type.
We further investigated the effect of the MHZ11(S39A) mutation on ethylene response, and found that the MHZ11(S39A)-FLAG protein could not rescue the ethylene-insensitive phenotype of mhz11 in ProMHZ11:MHZ11(S39A)-FLAG/mhz11 lines (Figure 4B). By contrast, normal MHZ11-FLAG protein can rescue the mhz11 ethylene response phenotype in ProMHZ11:MHZ11-FLAG/mhz11 lines. These results demonstrate that MHZ11 participates in ethylene responses through its acyl-hydrolyzing activity.
MHZ11 Functions Upstream of OsCTR2 in the Ethylene Signaling Pathway
To study the genetic relationship of MHZ11 with the known ethylene signaling components, genetic analyses were performed. In the wild type, overexpression of OsEIN2 and OsEIL1 resulted in strong constitutive and enhanced ethylene responses (Figures 5A and 5B). By crossing the overexpression lines of OsEIN2 and OsEIL1 with mhz11, lines overexpressing OsEIN2 or OsEIL1 in mhz11 background were generated. These lines exhibited a constitutive response phenotype in air and enhanced ethylene responses in roots after ethylene treatment (Figures 5A and 5B), suggesting that MHZ11 may function upstream of OsEIN2 and OsEIL1. We further generated lines overexpressing MHZ11 in the Osein2 and Oseil1 backgrounds by crossing. The constitutive short roots and root ethylene response of MHZ11OE-4 were completely abolished by Osein2 or Oseil1 mutation, further confirming that MHZ11 acts upstream of OsEIN2 and OsEIL1 in ethylene-regulated root growth (Figures 5C and 5D). In rice, the ethylene-receptor loss-of-function mutant Osers2 had mildly shorter roots compared with the wild type either in air or under ethylene treatment (Figure 5E). Double mutant analysis revealed that the Osers2 mhz11 exhibited long roots and ethylene insensitivity similar to mhz11, indicating that MHZ11 may function at or downstream of ethylene receptors (Figure 5E).
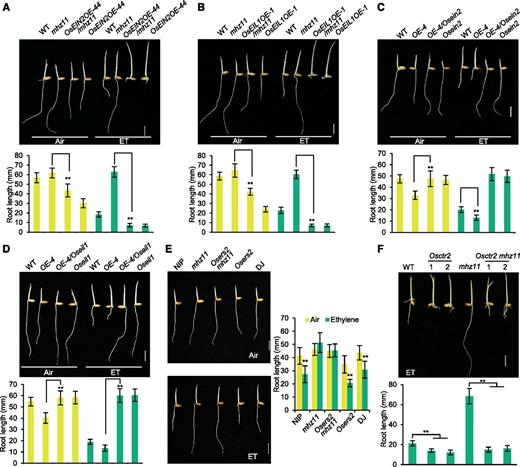
Genetic Relationship between MHZ11 and Ethylene Signaling Genes.
(A) and (B) Ethylene responses of OsEIN2-OE/mhz11 (A) and OsEIL1-OE/mhz11 (B) lines. The OsEIN2-OE/mhz11 and OsEIL1OE-1/mhz11 lines were generated by crossing mhz11 with OsEIN2OE-44 (an OsEIN2 overexpression transgenic line) and OsEIL1OE-1 (an OsEIL1 overexpression transgenic line), respectively. Seedlings of each line were grown in darkness with 10 µL L-1 of ethylene for 2.5 d. Scale bars = 10 mm. Data are means are means ± sd (n > 30) calculated from at least 30 seedlings (**P < 0.01, Student’s t test).
(C) and (D) Ethylene responses of MHZ11OE-4/Osein2 (C) and MHZ11OE-4/Oseil1 (D) lines. The MHZ11OE-4/Osein2 and MHZ11OE-4/Oseil1 lines were generated by crossing MHZ11OE-4 transgenic line with Osein2 and Oseil1 mutants, respectively. Seedlings were grown under darkness with 10 µL L-1 of ethylene treatment for 2.5 d. Scale bars = 10 mm. Data are means ± sd (n > 30) calculated from at least 30 seedlings (**P < 0.01, Student’s t test).
(E) Ethylene response of Osers2 mhz11 double mutant (left) and root length quantification (right). Osers2 mutant has a Dongjin (DJ) background. Seedlings of wild type (Nipponbare [NIP] and DJ) and mutants were grown in darkness with or without 1 µL L-1 of ethylene for 2 d. Scale bars = 10 mm. Root lengths are means ± sd (n > 20) calculated from at least 20 seedlings (**P < 0.01, Student’s t test; compared with corresponding air control).
(F) Osctr2 suppressed the ethylene-insensitive roots of mhz11. Osctr2 single and Osctr2 mhz11 double mutants were generated by mutating OsCTR2 in the wild type (WT) or mhz11 background using CRISPR/Cas9. Two Osctr2 lines and two Osctr2 mhz11 lines bearing the same mutation site within OsCTR2 (1039T deletion) were used for phenotype observation. Seedlings of each line were grown in darkness with 10 µL L-1 of ethylene for 2.5 d. Scale bars = 10 mm. Data are means ± sd (n > 25) calculated from at least 25 seedlings. One-way ANOVA was performed followed by a Tukey’s multiple comparisons test. **P < 0.01, Student’s t test.
Rice OsCTR2 is the functional homolog of Arabidopsis CTR1 (Wang et al., 2013). To study the genetic relationship between MHZ11 and OsCTR2, Osctr2 single and Osctr2 mhz11 double mutants were generated by mutating OsCTR2 in wild-type or mhz11 background using clustered regularly interspaced short palindromic repeats (CRISPR)/Cas9. Two Osctr2 lines and two Osctr2 mhz11 lines bearing the same mutation site within OsCTR2 (1039T deletion) were used for phenotype observation. The Osctr2 mutant showed enhanced short root phenotype and Osctr2 mutation suppressed the long root phenotype of mhz11 under ethylene treatment, suggesting that MHZ11 may function upstream of OsCTR2 (Figure 5F). All the genetic analyses suggest that MHZ11 acts at or downstream of ethylene receptors, but upstream of OsCTR2, OsEIN2, and OsEIL1 to regulate ethylene response of rice roots.
MHZ11 Is Essential for Ethylene-Induced Inhibition of OsCTR2 Phosphorylation
Given that MHZ11 may act upstream of OsCTR2, whether and how MHZ11 would affect OsCTR2 to regulate the ethylene response was further explored. A specific anti-OsCTR2 polyclonal antibody was generated by immunizing mice with polypeptides of OsCTR2 N terminus-specific sequence DKGGDPADRPAGSSGGGG. When testing the specificity of the antibody, an additional band above the original OsCTR2 band was detected, suggesting that the OsCTR2 protein is likely subject to post-translational modification (Figure 6A). The upper band vanished after 30 min of λ-protein phosphatase treatment, suggesting that the upper band represents a phosphorylated version of OsCTR2 (OsCTR2-P; Figure 6B).
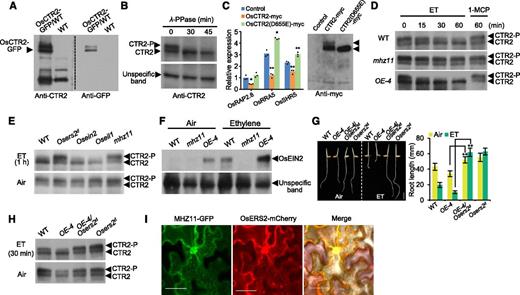
MHZ11 Modulates the Phosphorylation Status of OsCTR2.
(A) Specificity test of OsCTR2 antibody. The wild type (WT) indicates protoplast of wild-type rice. OsCTR2-GFP/WT indicates WT protoplast expressing OsCTR2-GFP protein.
(B) Detection of OsCTR2 in roots of the wild type. Protein extracts were treated with λ-PPase for different time lengths before being boiled with SDS loading buffer.
(C) The phosphorylation state of OsCTR2 is dependent on its kinase activity. OsCTR2(D655E) mimicked the Arabidopsis CTR1-1 mutant version CTR1(D694E), which is catalytically inactive. OsCTR2-myc and OsCTR2(D655E)-myc were transiently expressed in protoplasts of wild-type rice and biological activity of OsCTR2-myc and OsCTR2(D655E)-myc were compared by examining the inhibition effect they have on the expression of ERGs (left). The expressed proteins were detected with anti-myc antibody (right). Data are means ± sd, n = 3 (*P < 0.05, **P < 0.01, Student’s t test; compared with control).
(D) Phosphorylation time-course of OsCTR2 in roots of the wild type (WT), mhz11, and MHZ11OE-4 (OE-4) in response to ethylene. Dark-grown seedlings of wild-type, mhz11, and OE-4 were treated with ethylene (ET) and 1-MCP for different time lengths and total proteins of roots were extracted for immunoblot analysis.
(E) OsCTR2 phosphorylation status in roots of different ethylene signaling mutants. Roots of 2-d–old dark-grown seedlings were treated with 10 µL L-1 of ethylene for 1 h before collected for protein extraction. ET, ethylene; WT, wild type.
(F) Abundance of OsEIN2 protein in roots of the wild type (WT), mhz11, and MHZ11OE-4 (OE-4) etiolated seedlings. Two-d–old dark-grown seedlings were treated with or without 10 µL L-1 of ethylene (ET) for 6 h. The unspecific band served as an internal reference.
(G) Osers2d suppressed the short root phenotype of MHZ11-OE. Dark-grown seedlings were treated with 10 µL L-1 of ethylene (ET) for 2 d. Scale bar = 10 mm. Data are means ± sd (n > 30) calculated from at least 30 seedlings (**P < 0.01, Student’s t test). WT, wild type.
(H) Osers2d blocked the phosphorylation status change of OsCTR2 in OE-4 in response to ethylene. Roots of 2-d–old dark-grown seedlings treated with 10 µL L-1 of ethylene (ET) for 30 min were collected for protein extraction. WT, wild type.
(I) MHZ11 colocalized with OsERS2 on the ER membrane. Scale bars = 50 μm.
Furthermore, we tested whether the phosphorylated OsCTR2 resulted from its autophosphorylation. We generated a catalytically inactive OsCTR2(D655E), which mimicked the Arabidopsis CTR1-1 mutant version CTR1(D694E; Huang et al., 2003). In protoplasts of wild-type rice, while the OsCTR2 suppressed expression of the three ethylene responsive genes compared with control, the OsCTR2(D655E) protein lost the ability to suppress the expression of these genes (Figure 6C), indicating that OsCTR2(D655E) is biologically inactive. Also, OsCTR2(D655E) failed to suppress OsEIN2 accumulation (Supplemental Figure 3), further indicating that OsCTR2(D655E) has lost the ability to suppress ethylene signaling. The mutant protein OsCTR2(D655E) did not produce any phosphorylated form (Figure 6C), suggesting that the phosphorylated OsCTR2 is a consequence of autophosphorylation and OsCTR2 phosphorylation represents its kinase activity.
We subsequently examined the effect of MHZ11 on OsCTR2 phosphorylation status in roots in response to ethylene. In wild-type roots, phosphorylation levels of OsCTR2 decreased over time upon ethylene treatment within 1 h (Figure 6D, top). Meanwhile, the phosphorylation status of OsCTR2 stayed largely unchanged at high level in mhz11 (Figure 6D, middle). In MHZ11-overexpressing line OE-4, an apparent decline of OsCTR2 phosphorylation was observed in 15 min after ethylene treatment, and the phosphorylated OsCTR2 completely disappeared in 30 min after ethylene treatment (Figure 6D, bottom). After 1-MCP treatment blocking ethylene perception, all plants showed similar OsCTR2 phosphorylation levels, with a higher level of OsCTR2-P compared by OsCTR2. These results indicate that MHZ11 promotes ethylene inhibition of OsCTR2 phosphorylation/activity.
Because mhz11- and 1-MCP–treated samples had more OsCTR2-P than OsCTR2, we examined whether similar status was present in other ethylene-insensitive mutants identified in our previous studies. In air, the wild type and different mutants had similar higher level of OsCTR2-P than OsCTR2, indicating that more OsCTR2 proteins are in active forms under normal condition (Figure 6E, bottom). After ethylene treatment (10 µL L-1, 1 h), phosphorylation levels of OsCTR2 decreased apparently in wild type, indicating that ethylene inhibited OsCTR2 activity (Figure 6E, top). While the Osein2 and Oseil1 mutants had similar decreases of OsCTR2 phosphorylation as wild type in ethylene, the phosphorylation status of OsCTR2 in mhz11 and Osers2d (a dominant OsERS2 gain-of-function mutant) remained largely unaffected by ethylene (Figure 6E, top). These results indicate that MHZ11 mutation and OsERS2 gain-of-function mutation may lock OsCTR2 in a phosphorylation state, whereas mutations in downstream components OsEIN2 and OsEIL1 do not affect ethylene inhibition of OsCTR2 phosphorylation. This finding suggests that both MHZ11 and ethylene receptors are required for ethylene inhibition of OsCTR2 phosphorylation, and MHZ11 may act at ethylene receptor level for this function.
Because MHZ11 affects OsCTR2 phosphorylation/activity, we further examined whether the downstream OsEIN2 protein levels were altered. In air, no or little OsEIN2 protein was detected in the wild type and mhz11, while OE-4 had OsEIN2 protein (Figure 6F). This is consistent with the observation that the majority of OsCTR2 was in the active phosphorylation state in the wild type and mhz11 in the air, while only half or less of the OsCTR2 in OE-4 was phosphorylated (Figures 6D and 6E). The more phosphorylated OsCTR2 correlates with less OsEIN2 and vice versa. In ethylene, OsEIN2 levels were elevated in both the wild type and OE-4 but not in mhz11 (Figure 6F). These results support that MHZ11 mediates ethylene-induced OsEIN2 accumulation, a likely downstream event after OsCTR2 dephosphorylation/inactivation by MHZ11 function.
Taken together, our data indicate that MHZ11 is essential for ethylene-induced OsCTR2 dephosphorylation/inactivation.
MHZ11 Functions at the Ethylene Receptor Level
Given that both MHZ11 and the ethylene receptor OsERS2 are required for ethylene-induced inhibition of OsCTR2 phosphorylation, epistatic analysis was further performed to identify their relationship. MHZ11OE-4 line was crossed with the OsERS2 gain-of-function mutation (Osers2d) and the ethylene response of OE-4/Osers2d was examined. The constitutive short root and root ethylene response of OE-4 were completely abolished by Osers2d (Figure 6G), indicating that Osers2d is epistatic to MHZ11 in ethylene response. This was further confirmed by the presence of more phosphorylated OsCTR2 in OE-4/Osers2d after ethylene treatment (Figure 6H). These findings are consistent with the result that 1-MCP treatment suppressed the short root phenotype of OE-4 line (Figure 2I), suggesting that MHZ11’s positive role in modulating ethylene response requires ethylene perception and signal transduction initiation. While OsERS2 also requires MHZ11 for ethylene signaling (Figure 5E), and given the fact that MHZ11 is colocalized with OsERS2 on the ER membrane (Figure 6I), it may be concluded that MHZ11 functions at the ethylene receptor level to modulate ethylene response.
Sterol Is Partially Responsible for the Ethylene-Insensitive Phenotype of mhz11
We demonstrated that the acyl-hydrolyzing activity of MHZ11 is required for ethylene responses in rice roots, and MHZ11 functions through affecting ethylene receptor-dependent OsCTR2 phosphorylation to modulate ethylene signaling. However, it is not clear what specific lipid species is actually involved in the process. Free sterols are primary bilayer components regulating membrane fluidity and flexibility and affecting the functions of membrane-associated components (Lindsey et al., 2003; Schaller, 2004). Sterol acylation plays crucial roles in maintaining the free sterol content of cell membranes (Lewis et al., 1987; Dyas and Goad, 1993; Sturley, 1997; Schaller, 2004). Given that MHZ11 and other ethylene signaling components such as receptors and EIN2 are all ER membrane-integrated, it is possible that accumulation of free sterols in mhz11 impaired the proper functions of ethylene signaling components and caused the ethylene-insensitive phenotype of mhz11.
To test the hypothesis, free sterol contents were further measured and compared among wild-type, mhz11, and the OE-4 lines using the sterol detection kit (cat. no. K603-100; BioVision). Consistent with the lipidomics data, the level of free sterols was higher in mhz11 but lower in OE-4 compared with that of the wild type (Figure 7A). To test whether the accumulation of free sterols is responsible for the ethylene-insensitive phenotype of mhz11, we used the sterol biosynthesis inhibitor fenpropimorph (FEN; He et al., 2003) to treat the mhz11-etiolated seedlings. In the presence of FEN, ethylene significantly suppressed the root growth of mhz11, while the root growth of mhz11 under DMSO treatment remained insensitive to ethylene (Figure 7B). The results indicate that FEN treatment could partially rescue the ethylene-insensitive phenotype of mhz11, implying that reduction of sterol level may facilitate ethylene responses.
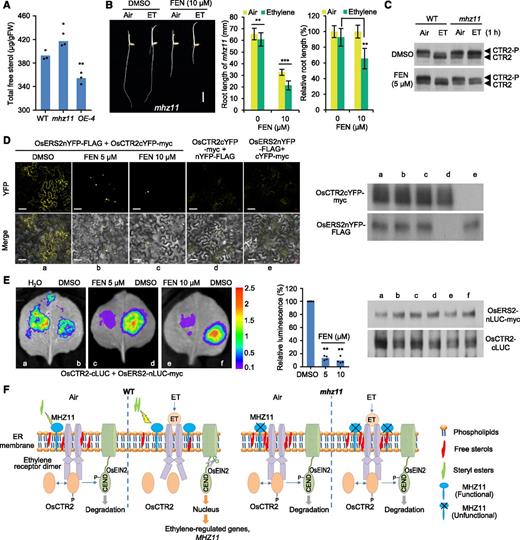
Overaccumulated Sterol in mhz11 Is At Least Partially Responsible for the Ethylene-Insensitive Phenotype of mhz11 Roots.
(A) Free sterol contents in roots of the wild-type (WT), mhz11 mutant, and overexpression line OE-4. Sterols were extracted from roots of 2-d–old dark-grown seedlings with CHCl3/IPA/NP-40 (7:11:0.1) and measured using a colorimetric method. Data are means ± sd (n = 3), 20 seedlings per biological replicate (*P < 0.05, **P < 0.01, Student’s t test).
(B) Sterol biosynthesis inhibitor FEN could partially restore the ethylene-insensitive phenotype of mhz11 roots. Representative seedlings grown in 10 µL L-1 of ethylene (ET) with DMSO or 10 μM of FEN are shown (left). Lengths (middle) and relative lengths (right) of roots are means ± sd (n > 20) calculated from at least 20 seedlings (**P < 0.01, ***P < 0.0001, Student’s t test; compared with the corresponding control).
(C) FEN treatment partially restored the ethylene inhibition effect on OsCTR2 phosphorylation in mhz11. Roots of 2-d–old etiolated seedlings grown in DMSO or 5 μM of FEN were treated with air or 10 µL L-1 of ethylene for 1 h before being collected for protein extraction. WT, wild type.
(D) FEN treatment suppressed the interaction between OsERS2 and OsCTR2 in an N. benthamiana epidermal cell BiFC system. Different concentrations of FEN were injected into N. benthamiana epidermal cells expressing OsERS2nYFP-FLAG and OsCTR2cYFP-myc. Confocal microscopy imaging was performed 12 h after FEN treatment (left). OsCTR2cYFP-myc + nYFP-FLAG and OsERS2nYFP-FLAG + cYFP-myc served as negative control. Scale bars = 50 μm. Immunoblot showed similar expression of OsCTR2cYFP-myc and OsERS2nYFP-FLAG proteins in each experimental group (right). Five randomly chosen regions of interest of infiltrated leaves in each group were observed with similar results.
(E) FEN treatment suppressed the interaction between OsERS2 and OsCTR2 in N. benthamiana epidermal cell split LUC complementation system. Different concentrations of FEN were injected into N. benthamiana epidermal cells expressing OsERS2-nLUC-myc and OsCTR2-cLUC. (Left) Color bar indicates relative fold changes. Relative luminescences are means ± sd from four leaves (**P < 0.01, Student’s t test; compared with DMSO; middle). Immunoblot showed similar expression of OsERS2-nLUC-myc and OsCTR2-cLUC proteins in each experimental group (right).
(F) A proposed working model for MHZ11. On the ER membrane, MHZ11 hydrolyzes phospholipids to release FFAs, which are used for sterol acylation, affecting sterol homeostasis. By maintaining a low sterol level on ER membrane, MHZ11 facilitates ethylene-triggered– and receptor-off-state–related OsCTR2 inactivation for downstream signaling. MHZ11 is transcriptionally activated by ethylene signaling, which may serve as a feedback regulation. In mhz11 mutant, overaccumulated free sterols likely hampered the ethylene-induced conformation change of ethylene (ET) receptors, leaving OsCTR2 constitutively active. OsEIN2 protein is thus constitutively phosphorylated and sent to degradation, and ethylene signal transduction is blocked. WT, wild type.
As mhz11 accumulates excess sterol (Figures 3A, 3C, and 7A) and the sterol biosynthesis inhibitor FEN partially restored the ethylene response of mhz11 (Figure 7B), we tested the effect of FEN on OsCTR2 phosphorylation. Under FEN treatment, ethylene apparently inhibited the phosphorylation of OsCTR2 in mhz11 roots (Figure 7C), indicating that sterol accumulation in mhz11 may at least partially impair the ethylene-induced OsCTR2 dephosphorylation. Because MHZ11 functions at the ethylene receptor level to modulate OsCTR2 phosphorylation, the possibility that FEN impairs the interaction between ethylene receptors and OsCTR2 was tested using the bimolecular fluorescence complementation (BiFC) assay. The results showed that FEN treatment obviously suppressed the interaction between OsERS2 and OsCTR2 in N. benthamiana epidermal cells using a BiFC system (Figure 7D), suggesting that low sterol levels could affect OsERS2 and OsCTR2 interaction. The split luciferase complementation assay in N. benthamiana epidermal cells also demonstrated that FEN treatment suppressed the interaction between OsERS2 and OsCTR2 without altering the protein abundance (Figure 7E). Taken together, our results imply that MHZ11-derived low level of membrane sterol could disrupt interaction of ethylene receptors with OsCTR2 and inhibit OsCTR2 phosphorylation for downstream signaling on ER membrane (Figure 7F).
DISCUSSION
In this study, we identified an ER membrane-integrated GDSL lipase MHZ11 with acyl-hydrolyzing activity that facilitates ethylene signaling in rice roots. MHZ11 hydrolyzes phospholipids to release fatty acids, which is likely further transferred to sterol to produce sterol esters, affecting sterol homeostasis. During this process, MHZ11 mediates the ethylene-inhibited OsCTR2 phosphorylation and the subsequent OsEIN2-related signal transduction. This process requires ethylene signal perception and signaling initiation. Blocking sterol biosynthesis can reduce OsERS2-OsCTR2 interaction, and suppress OsCTR2 phosphorylation in mhz11 in response to ethylene for restoration of ethylene response. Our study reveals a potential mechanism by which MHZ11 helps to maintain a low sterol level on ER membrane, and then facilitates the ethylene-triggered and receptor-off-state–related OsCTR2 inactivation for downstream signaling (Figure 7F).
Our study discovered that MHZ11 affects the phosphorylation of rice OsCTR2, which is homologous to CTR1 in Arabidopsis (Wang et al., 2013). In air, OsCTR2 has two forms, i.e., the phosphorylated and nonphosphorylated, and the phosphorylated form appeared to be the majority (Figure 6). Upon ethylene treatment, the level of phosphorylated form (OsCTR2-P) is reduced and the nonphosphorylated form seems to be enhanced, implying that the two states may be easily changeable at least at very early stages of the treatment. This process is regulated by MHZ11. MHZ11 overexpression caused faster decrease of OsCTR2-P level whereas MHZ11 mutation locked OsCTR2-P at a constantly higher level than nonphosphorylated OsCTR2. The OsCTR2-P increases with increasing OsCTR2 kinase activity (Figure 6C) and can be regarded as a default state for a no-ethylene situation or an ethylene receptor-on situation. By contrast, loss of OsCTR2-P indicates the initiation of ethylene signaling, and the lower ratio of OsCTR2-P/OsCTR2 may reflect the progress of signaling or intensity/strength of the later ethylene response. Consistently, the OsCTR2-P level is negatively correlated with the OsEIN2 level (Figure 6), which is positively correlated with the ethylene response (Qiao et al., 2009). This fact provides in vivo evidence for the connection between OsCTR2 dephosphorylation/inactivation and ethylene signaling activation, consistent with the idea that Arabidopsis CTR1 functions through its kinase activity to phosphorylate EIN2 and thus control ethylene signaling (Ju et al., 2012). Also, the findings that OsERS2 gain-of-function mutation or 1-MCP treatment keeps more OsCTR2 in the phosphorylated state provides the in vivo biochemical evidence for the relationship between ethylene receptors and OsCTR2 activity, which is only speculated in the canonical Arabidopsis model (Hua and Meyerowitz, 1998).
MHZ11 has demonstrated acyl-hydrolyzing activity and causes changes of lipid profiles in rice roots. Among the MHZ11-related lipid species, we show that sterol plays a role in OsCTR2 regulation. Inhibition of sterol biosynthesis by the inhibitor FEN led to partial rescue of the mhz11 ethylene response, and this function appears to be due to blocking of the OsERS2-OsCTR2 interaction and suppression of OsCTR2 phosphorylation (Figure 7). Sterols are primary components of bilayers where they regulate membrane fluidity, properties, and functions. Previous studies suggested that changes in sterol contents affect the functions of membrane-associated receptors or components of signal transduction pathways (Lindsey et al., 2003; Schaller, 2004). As a kind of sterol modification, sterol acylation is essential in maintaining the free sterol content of cell membranes at their physiological levels as steryl esters cannot participate in the bilayer of the membranes (Lewis et al., 1987; Dyas and Goad, 1993; Sturley, 1997; Schaller, 2004). Phospholipids could serve as acyl donors for sterol esterification (Zimowski and Wojciechowski, 1981; Dyas and Goad, 1993). We propose that MHZ11 helps maintain a proper free sterol level on the ER membrane through affecting sterol esterification. It is most likely that the low sterol levels caused by MHZ11 function may increase membrane fluidity and flexibility of the microdomains hosting the ethylene receptors and CTRs, facilitating conformational change and thus dissociation of the protein complex in response to ethylene for downstream signaling. Consistent with this, Arabidopsis sterol biosynthesis mutants hydra1 and hydra2 exhibited abnormal root hair patterning that could be rescued by ETHYLENE RESPONSE1 (ETR1) mutant etr1-1 or silver ion treatment (Souter et al., 2002; Lindsey et al., 2003), indicating that sterols may play roles in ethylene signal transduction. It should be mentioned that the differences among wild type, mhz11, and OE-4 are visually small but statistically significant, leading to the corresponding change of ethylene response (Figures 3 and 7). Phospholipids and free sterols are the structural lipid components of cellular membranes and mild alterations of their abundance may result in strong phenotype changes. Previous studies showed that mutations of the genes for patatin-related phospholipase OspPLAIIIα in rice and pPLAIIIβ in Arabidopsis, both with PLA activities, caused similar slight changes of phospholipids but have a series of growth defects (Li et al., 2011; Liu et al., 2015).
In addition to the structural role, campesterol also served as the precursor for the biosynthesis of brassinosteroids (Yokota, 1997). While the brassinosteroids were detected at higher levels in mhz11 compared with those in wild type (Supplemental Figure 4A), brassinazole (Brz, a brassinosteroid biosynthesis inhibitor) treatment did not restore the ethylene response of mhz11 (Supplemental Figure 4B), suggesting that mhz11 phenotype is not due to the overaccumulation of brassinosteroids.
It should be noted that while we find a potential role for MHZ11-derived low sterol level in OsCTR2 inactivation in rice roots, the possibility that other lipid species participate in this process cannot be excluded. The effect of PA on mhz11 was tested as a previous study reported that PA binds to CTR1 and inhibits its kinase activity in vitro in Arabidopsis (Testerink et al., 2007). Dioctanoylphosphatidic acid (a water-soluble PA) treatment could not rescue the ethylene response of mhz11 roots, suggesting that PA may not be responsible for the mhz11 phenotype (Supplemental Figure 5A). This discrepancy may be because different plant species may adopt different mechanisms for regulation. The effects of FFAs and lyso-phospholipids on mhz11 phenotype were also tested as they are the direct hydrolysis products of phospholipids by MHZ11. LPC16:0 and FFA18:1, the potential hydrolyzing products of MHZ11 whose contents are higher in MHZ11 OE-4 plants compared with the wild type (Figure 3C), were selected for the rescue. Neither rescued the mhz11 phenotype (Supplemental Figures 5B and 5C), suggesting that these lipids are unlikely to be directly responsible for the mhz11 phenotype.
It should be mentioned that the secreted GDSL lipase AtGLIP1 (GDSL LIPASE-LIKE 1), which is different from our present ER-membrane localized MHZ11, has been found to be involved in resistance to A. brassicicola and ethylene responses in Arabidopsis. This gene may contribute to the production of a systemic signal(s) that function at the downstream of the canonical ethylene signaling pathway (Oh et al., 2005; Kwon et al., 2009; Kim et al., 2013, 2014).
While our mhz11 mutant is insensitive to ethylene in root growth inhibition, it seems that the aerial parts are not affected. We examined the OsCTR2 phosphorylation status in shoots of the wild type, mhz11, and OE-4. In response to ethylene, the OE-4 line had similar decrease trend of OsCTR2 phosphorylation compared with that of the wild type, while the OsCTR2 phosphorylation was largely maintained in mhz11 shoots (Supplemental Figure 6A). The fact that the mhz11 shoots maintained OsCTR2 phosphorylation but showed normal ethylene response may be due to the shoot/root differential responses to the change of endogenous OsCTR2 phosphorylation. The OsCTR2 phosphorylation level in mhz11 shoots may not be sufficient to suppress the ethylene response of coleoptile/shoot. In our RNA sequencing (RNA-seq) data, the ethylene responsiveness of 34% of the total ERGs in shoots is impaired in mhz11 mutant (Supplemental Figure 6B; Supplemental Data Set 2), suggesting that ethylene signaling may be partially impaired in mhz11 shoots. q-PCR analysis also reveals that several ERGs are differentially affected in mhz11 shoots (Supplemental Figures 6C and 6D).
Collectively, we identified a novel ER-localized GDSL lipase MHZ11 with acyl-hydrolyzing activity, which participates in ethylene signaling by modulating OsCTR2 phosphorylation. This function is likely achieved by affecting sterol homeostasis. Our study discovers a previously unidentified mechanism and provides insights into the understanding of how OsCTR2 is regulated during ethylene signal transduction.
METHODS
Plant Materials and Growth Conditions
mhz11 and Osers2d mutants were identified in our genetic screen for rice (Oryza sativa) ethylene-response mutants from our EMS-induced mutant pools of Nipponbare rice (Zhou et al., 2019). Osers2d harbors a dominant gain-of-function version of OsERS2(A32V) which is equivalent to Arabidopsis (Arabidopsis thaliana) etr1-3 (Hall et al., 1999). mhz7/Osein2 and mhz6/Oseil1 are previously identified ethylene-response mutants in our lab (Ma et al., 2013; Yang et al., 2015). mhz11-2 and Osers2 are T-DNA insertion mutants obtained from the POSTECH Biotech Center (Yi and An, 2013). The OsEIN2-OE/mhz11, OsEIL1OE-1/mhz11, and Osers2 mhz11 lines are generated by crossing mhz11 with OsEIN2OE-44 (an OsEIN2 overexpression transgenic line), OsEIL1OE-1 (an OsEIL1 overexpression transgenic line), and Osers2, respectively. The MHZ11OE-4/Osein2, MHZ11OE-4/Oseil1, and MHZ11OE-4/Osers2d lines were generated by crossing MHZ11OE-4 transgenic line with Osein2, Oseil1, and Osers2d, respectively. Osctr2 single and Osctr2 mhz11 double mutants were generated by mutating OsCTR2 in wild-type or mhz11 background using CRISPR/Cas9. Two Osctr2 lines and two Osctr2 mhz11 lines from different transformation events bearing the same mutation site within OsCTR2 (1039T deletion) were used for phenotype observation.
All rice plants used in this study were grown in the experimental fields of the Institute of Genetics and Developmental Biology in Beijing from May to October and in Hainan from November to next April each year between 2014 and 2019. Ethylene treatment of rice seedlings was performed as previously described by Ma et al. (2013, 2018). For FEN and Brz treatment, stocks solution of FEN (Cayman, 20,875) and Brz (Cayman, 16,804) were prepared in DMSO and diluted into solutions of different concentrations with water. Equivalent volumes of DMSO were added to the control.
Map-Based Cloning and Complementation Assay
F2 mapping populations used for map-based cloning was generated by crossing mhz11 with an indica rice, MH63. The MHZ11 locus was mapped to the short arm of chromosome 5, within a 53.3-kb genomic region. Genes and the relevant sequences in this region were amplified for sequencing. For genetic complementary assay, wild-type genomic sequence of MHZ11 (3,016-bp sequence upstream of ATG codon, 2,756-bp genomic coding sequence, and 1,578-bp sequence downstream of stop codon) was cloned into the pCAMBIA2300 vector and transformed into mhz11. The mhz11 background in the transgenic lines was confirmed by dCAPS analysis. The fragment of mhz11 mutant was 18-bp shorter than that of the wild type. The primers are listed in Supplemental Table.
Gene Expression Analysis by Real-Time PCR
For gene expression analysis under ethylene treatment, 2-d–old etiolated seedlings of wild type and mhz11 were treated with or without 10 µL L-1 of ethylene. Roots and shoots of the seedlings were harvested for total RNA extraction using TRIZOL reagent (Invitrogen). RNA was treated with TURBO DNase (Invitrogen) for genomic DNA digestion. cDNAs were synthesized using a Maxima First Strand cDNA Synthesis Kit (Thermo Fisher Scientific) and real-time PCR was conducted according to the instructions of TransStart Green qPCR SuperMix (TransGen Biotech). OsActin2 was used as internal control. The primers are listed in the Supplemental Table. Each data point has three biological replicates.
GUS Staining
Etiolated seedlings treated with air or ethylene were fixed in 90% (v/v) acetone on ice for 15 min and washed with staining buffer (100 mM of Na3PO4 buffer at pH 7.0, 10 mM of EDTA, 5 mM of potassium ferricyanide, 5 mM of potassium ferrocyanide, and 0.1% [v/v] Triton X-100) for two times. Samples were then soaked in staining solution, which is a staining buffer containing 0.5 mg/mL of X-Gluc (cat. no. B8049; Sigma-Aldrich) for 10 min in a vacuum system before being incubated at 37°C in the dark for 1 h. The samples were observed using a stereo microscope (model no. M165 FC; Leica; Ma et al., 2014).
Phylogenetic Analysis
Alignment of MHZ11 and the GDSL-family members in Arabidopsis and rice were analyzed according to the sequences from Chepyshko et al. (2012) and Lai et al. (2017). The phylogenetic tree of plant GDSL lipases/esterases was built using maximum likelihood (ML) with the program MEGA6 (Tamura et al., 2013). One-thousand bootstrap replicates were each used in nearest-neighbor interchange searches for the best ML tree. The ML tree was visualized online with the program iTOL (http://itol.embl.de/; Letunic and Bork, 2019). Sequence alignments and the tree file are provided as Supplemental Files 1 and 2, respectively.
Subcellular Localization
Microsomal membrane proteins were isolated according to Ma et al. (2018). In brief, 3 g of seedling roots were ground in liquid nitrogen to a fine powder and dissolved in 3 mL of extraction buffer (100 mM of Tris-HCl at pH 8.0, 150 mM of KCl, 5 mM of EDTA, 10% Glycerol [v/v], 3.3 of mM dithiothreitol, 0.6% [w/v] polyvinylpyrrolidone, and 2% [v/v] protease inhibitor cocktail; Sigma-Aldrich). Homogenates were centrifuged at 16,000g for 10 min at 4°C for three times to remove debris. Supernatants were further centrifuged at 100,000g for 60 min at 4°C and the microsomal membrane were washed three times with extraction buffer before dissolved in 100 μL of extraction buffer containing 1% (v/v) Triton X-100 and 0.1% (w/v) SDS. Samples were heated at 65°C for 10 min with SDS loading buffer before loaded for SDS-PAGE and immunoblot analysis.
For analyses of MHZ11 subcellular localization, MHZ11 and different truncated versions were fused to GFP or YFP and transiently expressed in Nicotiana benthamiana leaf cells before fluorescence was observed in the epidermal cells. HDEL-mCherry was used as an ER marker. For plasmolysis analysis, N. benthamiana leaf cells expressing MHZ11-YFP were treated with 30% (w/v) Suc for 5 min.
Ethylene Production Measurement
Ethylene production measurement was performed according to Yin et al. (2015). In brief, seedlings of wild-type, mhz11, and OE lines were grown in 40-mL uncapped vials for 7 d in dark at 28°C. Vials were then sealed with rubber syringe caps for 17 h before 1 mL of headspace of each vial was measured using a gas chromatograph (model no. GC-2014; Shimadzu).
Lipidomics Analysis
The lipidomics analysis of rice seedlings was performed as described by Xiong et al. (2017). In brief, roots of 3-d–old etiolated rice seedlings were collected for lipid extraction, which was performed using a modified version of the Bligh and Dyer’s protocol as described by Lam et al. (2014b). Polar lipids were analyzed using a model no. 1260 HPLC system (Agilent Technologies) coupled with a triple quadrupole/ion trap mass spectrometer (QTRAP 5500; SCIEX) as described by Lam et al. (2014a). Four biological repeats were performed. Individual lipid species were quantified by referencing to spiked internal standards.
Enzyme Activity Assay
The PLA2 activity of MHZ11 was tested using a PLA2 Activity Assay Kit (cat. no. K400-100; BioVision). MHZ11-FLAG and MHZ11(S39A)-FLAG proteins were purified from transgenic plants using the anti-FLAG M2 Magnetic Beads (Sigma-Aldrich) and concentrated. For PLA2 activity measurement, proteins were incubated with a synthetic thiophospholipid at 37°C, producing a lysothiophospholipid that reacts with a fluorogenic probe to produce a fluorescent product detectable in the visible range (Excitation = 388 nm/Emission = 513 nm), and measured in relative fluorescence units. Bee venom provided in the PLA2 activity kit and water served as positive and negative controls, respectively.
Measurement of Brassinosteroids
Measurements of brassinosteroids in rice seedlings were performed as previously described by Xin et al. (2016). Roots of 3-d–old etiolated rice seedlings were collected and ground in liquid nitrogen to a fine powder before brassinosteroid extraction. Brassinosteroid contents were analyzed based on ultra-performance liquid chromatography-mass spectrometry.
OsCTR2 Antibody Generation and Immunoblot Analysis
The specific anti-OsCTR2 and anti-OsEIN2 polyclonal antibodies were generated by immunizing mice with synthetic KLH-conjugated peptides of OsCTR2 N terminus-specific sequence DKGGDPADRPAGSSGGGG and OsEIN2-specific sequence PNILESDNKPLGGNNPS, respectively. For immunoblot analysis, proteins were heated at 65°C for 5 min with SDS-PAGE loading buffer and separated using SDS-PAGE. Primary antibody dilutions were in PBS containing 3% (w/v) milk. The anti-OsCTR2 and anti-OsEIN2 antibodies were diluted in Immunoreaction Enhancer Solution I (Toyobo). The primary antibodies used were: anti-FLAG (FLA-1, 1:3,000, cat. no. M185-3; MBL), anti-BiP (1:5,000, ER marker, cat. no. AS09 481; Agrisera), anti-UGPase (1:5,000, cytoplasm marker, cat. no. AS05 086; Agrisera), anti-GFP (7G9, 1:5,000, cat. no. M20004H; Abmart), anti-c-Myc (19C2, 1:2,000, HRP-conjugated, cat. no. M20019L; Abmart), anti-OsCTR2 (1:10,000), and anti-OsEIN2 (1:10,000). Secondary goat anti-mouse-IgG-horseradish peroxidase (cat. no. M210021; Abmart) antibody was used at 1:10,000 dilutions in PBS containing 3% (w/v) milk to detect FLAG-tagged proteins, while also being used at 1:10,000 to detect OsCTR2 and OsEIN2. Secondary goat anti-rabbit-IgG-horseradish peroxidase (cat. no. M210011; Abmart) antibody was used at 1:10,000 dilutions in PBS containing 3% (w/v) milk. The signals were detected by chemiluminescence method using WesternBright ECL Detection Kit (cat. no. K-12045-D50; Advansta) while the WesternBright Sirius Detection Kit (cat. no. K-12043-D10; Advansta) was used to detect OsCTR2 and OsEIN2.
BiFC Assays
For interaction of OsERS2 and OsCTR2, OsERS2 and OsCTR2 coding sequences were fused to the N-terminal half of YFP (nYFP) and C-terminal half (cYFP), respectively. The nYFP and cYFP were tagged with flag and myc, respectively. Plasmids were transformed into N. benthamiana leaf cells through Agrobacterium tumefaciens strain GV3101. The combination of OsCTR2cYFP-myc and nYFP-FLAG, OsERS2nYFP-FLAG, and cYFP-myc served as negative controls. Transformed N. benthamiana plants were grown at 23°C for 48 h before being infiltrated with different concentrations of FEN or DMSO. YFP fluorescence was detected 12 h later using a confocal microscope.
Split Luciferase Complementation Assay
OsERS2 and OsCTR2 coding sequences were fused to the N-terminal half of Luciferase (LUC) and C-terminal half, respectively. The N-terminal half of LUC was tagged with myc tag. Plasmids were transformed into N. benthamiana leaf cells through A. tumefaciens strain GV3101. Transformed N. benthamiana plants were grown at 23°C for 48 h before being infiltrated with different concentrations of FEN or DMSO. LUC activity was observed with a low-light cooled charge-coupled device imaging apparatus (iXon; Andor Technology).
Statistical Analysis
The relative root or coleoptile length of each mutant is analyzed relative to the length in untreated conditions. All of the data were analyzed using ANOVA or Student’s t test. Detailed descriptions of statistical analyses are presented in Supplemental Data Set 3.
RNA-Seq Analysis
For RNA-seq analysis, 2-d–old etiolated seedlings of wild type and mhz11 were treated with air or 10 µL L-1 of ethylene for 8 h. Roots and shoots of the seedlings were collected separately and subjected to RNA-seq analysis with three biological replicates. Genes with at least 2-fold changes of expression in ethylene treatment compared with those in the air (false discovery rate ≤ 0.05) were identified as ERGs. ERGs no longer induced by ethylene in mhz11 were identified as MHZ11-dependent ERGs.
Data Availability
The raw sequencing data reported in this article have been deposited in the Sequence Read Archive (https://www.ncbi.nlm.nih.gov/sra) under a National Center for Biotechnology Information BioProject accession number (PRJNA600591) and National Center for Biotechnology Information BioSample accession numbers (SAMN13826478 to SAMN13826513).
Accession Numbers
Sequence data from this article can be found in the GenBank library under the following accession numbers: Os05g11950 (MHZ11), Os06g08340 (OsERF002), Os11g05740 (OsRAP2.8), Os07g26720 (OsRRA5), Os05g06320 (OsERS2), Os07g06130 (OsEIN2), Os03g20790 (OsEIL1), and Os02g32610 (OsCTR2).
Supplemental Data
Supplemental Figure 1. MHZ11 is a GDSL lipase that positively modulates ethylene response supports Figures 1 and 2).
Supplemental Figure 2. MHZ11 is localized to the ER membrane and is induced by ethylene (supports Figure 2).
Supplemental Figure 3. OsCTR2 kinase activity is required for its function in ethylene signaling (supports Figure 6).
Supplemental Figure 4. MHZ11 affects the brassinosteroids levels in rice roots (supports Figure 7).
Supplemental Figure 5. PA, LPC, and FFA and treatment failed to rescue the ethylene insensitivity of mhz11 roots (supports Figure 7).
Supplemental Figure 6. OsCTR2 phosphorylation status in shoots and expression of ERGs in roots/shoots of rice (supports Figures 6 and 7).
Supplemental Table. Primers used in this study.
Supplemental Data Set 1. Lipid profile analysis of wild-type, mhz11, and OE-4 roots in air and ethylene.
Supplemental Data Set 2. RNA-seq analysis of ERGs in wild type and mhz11 mutant.
Supplemental Data Set 3. Summary of statistical tests.
Supplemental File 1. Alignment of GDSL family lipases from rice and Arabidopsis.
Supplemental File 2. Phylogenetic relationships among GDSL family lipases from rice and Arabidopsis.
DIVE Curated Terms
The following phenotypic, genotypic, and functional terms are of significance to the work described in this paper:
Acknowledgments
This work is supported by the National Natural Science Foundation of China (31530004, 31670274, and 31600980), 973 Projects (2015CB755702, and the State Key Lab of Plant Genomics.
AUTHOR CONTRIBUTIONS
J.-S.Z., H.Z., B.M., and S.-Y.C. designed the research; H.Z. performed most of the research; B.M. and K.-X.D. isolated the mutants; X.-K.L. performed the rice protoplast isolation and transformation; X.L. performed RNA-seq analysis; C.-C.Y., J.-J.T., W.W. and W.-K.Z. helped performed the genetic analyses; G.-H.S. and S.M.L. performed the lipid measurement and analysis; J.-F.C. and P.-Y.X. performed the brassinosteroid measurements; all authors contributed to material preparation and data analysis; H.Z. and J.-S.Z. wrote the article; all authors read and approved the article.
References
Author notes
These authors contributed equally to this work.
The author responsible for distribution of materials integral to the findings presented in this article in accordance with the policy described in the Instructions for Authors (www.plantcell.org) is: Jin-Song Zhang ([email protected]).
Articles can be viewed without a subscription.