-
PDF
- Split View
-
Views
-
Cite
Cite
Qi Li, Jiechen Wang, Jianwei Ye, Xixi Zheng, Xiaoli Xiang, Changsheng Li, Miaomiao Fu, Qiong Wang, Zhiyong Zhang, Yongrui Wu, The Maize Imprinted Gene Floury3 Encodes a PLATZ Protein Required for tRNA and 5S rRNA Transcription through Interaction with RNA Polymerase III, The Plant Cell, Volume 29, Issue 10, October 2017, Pages 2661–2675, https://doi.org/10.1105/tpc.17.00576
- Share Icon Share
Abstract
Maize (Zea mays) floury3 (fl3) is a classic semidominant negative mutant that exhibits severe defects in the endosperm but fl3 plants otherwise appear normal. We cloned the fl3 gene and determined that it encodes a PLATZ (plant AT-rich sequence and zinc binding) protein. The mutation in fl3 resulted in an Asn-to-His replacement in the conserved PLATZ domain, creating a dominant allele. Fl3 is specifically expressed in starchy endosperm cells and regulated by genomic imprinting, which leads to the suppressed expression of fl3 when transmitted through the male, perhaps as a consequence the semidominant behavior. Yeast two-hybrid screening and bimolecular luciferase complementation experiments revealed that FL3 interacts with the RNA polymerase III subunit 53 (RPC53) and transcription factor class C 1 (TFC1), two critical factors of the RNA polymerase III (RNAPIII) transcription complex. In the fl3 endosperm, the levels of many tRNAs and 5S rRNA that are transcribed by RNAPIII are significantly reduced, suggesting that the incorrectly folded fl3 protein may impair the function of RNAPIII. The transcriptome is dramatically altered in fl3 mutants, in which the downregulated genes are primarily enriched in pathways related to translation, ribosome, misfolded protein responses, and nutrient reservoir activity. Collectively, these changes may lead to defects in endosperm development and storage reserve filling in fl3 seeds.
INTRODUCTION
Maize (Zea mays) is one of the most important feed and food crops in the world. With the earth's fast-growing population, the demand for sustainable production of maize seeds with high nutritional quality is increasing (Wheeler and von Braun, 2013; Zhang et al., 2016). In maize, the endosperm accounts for 90% of the dry seed weight and serves as the main organ of nutrient storage. The endosperm provides abundant starch and proteins for food, biofuels, and chemical production. Therefore, a better understanding of maize endosperm development and filling is critical for its genetic improvement (Sabelli and Larkins, 2009; Olsen and Becraft, 2013).
The endosperm is a highly differentiated organ that contains four cell types: the aleurone cell layer, starchy endosperm cells, basal endosperm transfer layers (BETL), and embryo surrounding cells; these cell types perform specific functions during endosperm development. Aleurone is a single cell layer that functions in mineral storage and later by acting as digestive tissue that responds to gibberellic acid upon germination to produce a variety of catabolic enzymes. Starchy endosperm cells, the main portion of the endosperm that is directly underneath the aleurone, are specialized for the synthesis and storage of starch and proteins. The BETL transports nutrient solutes from the maternal placenta to the developing endosperm. Embryo surrounding cells are hypothesized to function in communication and nutrient transfer between the endosperm and embryo (Sabelli and Larkins, 2009; Olsen and Becraft, 2013). As the starchy endosperm is the largest in size and fulfills a simple biological function, many mutations in the endosperm cause easily recognized but viable phenotypes, with the most well known being opaque and floury. The opaque and floury mutants bear a chalky and soft endosperm that does not allow light to transmit through the seed. However, they differ in genetic behavior, with the floury mutants being semidominant or dominant (i.e., fl1, fl2, and fl3) and the opaque being recessive (i.e., o1 and o2) (Hays and East, 1915; Lindstrom, 1923; Emerson et al., 1935; Maize Genetics Corporation, 1939; Ma and Nelson, 1975; Coleman et al., 1997; Wu and Messing, 2010a). The most abundant storage proteins in the maize endosperm are alcohol-soluble proteins called zeins. Thus far, 11 naturally occurring opaque and floury mutants have been cloned; all but o5 are related to zein synthesis or organization in protein bodies (Myers et al., 2011). The o2 mutation affects the transcription of most zein genes (Schmidt et al., 1992; Zhang et al., 2015; Yang et al., 2016); fl2, fl4, De-B30*, and Mucronate1 affect the zein protein structure (Coleman et al., 1997; Kim et al., 2004, 2006; Wang et al., 2014a); fl1, o1, and o10 affect zein protein deposition and protein body formation (Holding et al., 2007; Wang et al., 2012; Yao et al., 2016); and o6 (also called pro1) and o7 are related to metabolic processes that affect zein protein accumulation (Miclaus et al., 2011; Wang et al., 2011, 2014b). The o5 mutation is not involved in the above processes; it results from a defective monogalactosyl diacylglyerol synthase and affects the phospholipid composition of amyloplast membranes (Myers et al., 2011). Cloning and functional analyses of these mutants have greatly promoted our understanding of endosperm development, storage reserve synthesis, and vitreous and soft endosperm formation (Gibbon and Larkins, 2005; Wu and Messing, 2017). However, there is more to learn about the control of the mid and latter stages of endosperm development.
Because zeins are devoid of the essential amino acids lysine and tryptophan, their abundance results in poor-quality grain proteins (Osborne et al., 1914). In the 1960s, o2 was found to cause a reduction in zeins, a compensatory increase in non-zein proteins, and as a consequence, enhanced lysine levels (Mertz et al., 1964). Other nonvitreous mutants were also collected by Oliver Nelson for amino acid analysis, among which fl3 was obtained from Bear Seed Company in 1968 (Ma and Nelson, 1975). This mutant has been designated fl3 because it was observed that two genetic doses of the mutant gene in the endosperm cause the floury phenotype as do fl1 and fl2. Despite the increased lysine content in the fl3 endosperm, it cannot be applied for quality protein maize breeding because the fl3 mutation causes severe defects in endosperm development and a dramatic reduction in seed weight. However, the fl3 plant appears identical to the wild type in terms of plant height, leaves, flowering time, and seed setting, indicating that fl3 is an endosperm-specific mutant and that the mutation affects endosperm development and storage reserve filling.
The mRNA transcripts encoding storage proteins are predominant in total mRNA species in starchy endosperm cells during the filling stage (Chen et al., 2014). Moreover, highly abundant ribosomal proteins, tRNAs and rRNAs, are also required to achieve high-efficiency functioning of the protein translation machinery. The RNA polymerase III (RNAPIII) complex is specialized for the transcription of tRNAs and other small noncoding RNAs including 5S rRNA, U6 spliceosomal RNA, and 7SL RNA (Nikitina et al., 2011; Nielsen et al., 2013; Turowski and Tollervey, 2016). In yeast, the RNAPIII transcription complex involves RNAPIII, a 17-subunit enzyme, and three transcription factors (TFIIIA, TFIIIB, and TFIIIC) (Acker et al., 2013). TFIIIA is specific for the transcription of 5S rRNA. TFIIIB is common to all eukaryotic RNAPs. TFIIIC binds to the internal promoter elements and subsequently recruits TFIIIB upstream of the transcription start site. TFIIIB and TFIIIC form the preinitiation complex, binding to the promoters and intragenic regions of tRNAs and 5S rRNA for the recruitment of RNAPIII (Dieci et al., 2013; Male et al., 2015). tRNA biogenesis also demands the fine modulation of RNAPIII transcription. One key regulator that interacts with RNAPIII transcription machinery, MAF1, suppresses RNAPIII transcription to adapt to nutritional stress (Boguta, 2013). Further studies revealed that MAF1 weakens the interaction between the RNAPIII subunit RPC37 and the TFIIIB member BRF1 (Boguta, 2013). Despite these findings in yeast, the components and mechanisms that modulate RNAPIII transcription in plants, especially in the endosperm, which is characteristic of highly efficient storage reserve synthesis, have not been determined.
Here, we cloned the classic semidominant mutant fl3 by map-based cloning. Fl3 encodes a novel protein belonging to the PLATZ (plant AT-rich sequence and zinc binding) family, which is specifically expressed in starchy endosperm cells. The fl3 mutation results from a dominant mutation in the conserved PLATZ domain. However, Fl3 is a maternally expressed imprinted gene, leading to the suppression of fl3 when it is transmitted from the male. Consequently, this combinatory effect causes the dominant mutation to exhibit semidominant behavior. FL3 interacts with two critical components of the RNAPIII transcription machinery, RPC53 and TFC1. The fl3 mutation causes a reduction in tRNA and 5S rRNA expression and dramatically alters the transcriptome. Our work identified FL3 as a novel factor in maize that modulates the biogenesis of tRNAs and 5S rRNA through protein-protein interactions with RNAPIII transcription machinery, which may underlie endosperm development and storage reserve filling.
RESULTS
Semidominant Behavior of fl3
The reference fl3 mutation causes a semidominant negative effect on endosperm development, but develops a normal plant (Ma and Nelson, 1975). To fully characterize fl3, we obtained the mutant from the Maize Genetics Cooperation Stock Center (accession number 805A). Some mutants were not homozygous and therefore segregated out normal seeds, which were propagated for one more generation. Normal seeds from a nonsegregating ear were used as the wild type (WT) in the following experiments. Self-pollination and reciprocal crosses of fl3 and the wild type were performed to study the genetic inheritance and action of fl3. Because the endosperm is triploid (two sets of chromosomes from the female parent and one from the male), the resulting four progenies had three (fl3), two (fl3 × WT), one (WT × fl3), and zero (WT) doses of fl3. Indeed, the mutant phenotype in the endosperm only occurs when fl3 is transmitted through the female (Figures 1A and 1B). All progeny of fl3 × WT that contained two doses of the mutant gene displayed the mutant phenotype, as observed in the homozygous fl3 with three doses, while those from WT × fl3 that harbors only one dose of the mutant gene exhibited a normal phenotype. Consistent with their phenotypes, the starch granules in the outer region of the endosperm were tightly embedded in a proteinaceous matrix in WT and WT × fl3 but were naked in fl3 × WT and fl3 (Figure 1C). When fl3 × WT seeds were planted and crossed to the wild-type inbred line B73 as females, the vitreous and floury seeds on the cobs were equally segregated (Supplemental Figures 1A to 1D), confirming that fl3 is a single-gene mutation. When either type of F1 (fl3 × WT and WT × fl3) was self-pollinated, the resulting F2 progeny segregated vitreous and floury seeds at a 1:1 ratio (Supplemental Figure 1E). Mutant seeds with fl3fl3/fl3 and fl3fl3/+ genotypes and those with ++/fl3 and ++/+ (normal) were phenotypically indistinguishable. Based on these detailed genetic analyses, it appears that the mutant phenotype caused by the fl3 reference allele is not equally contributed by the two parents.
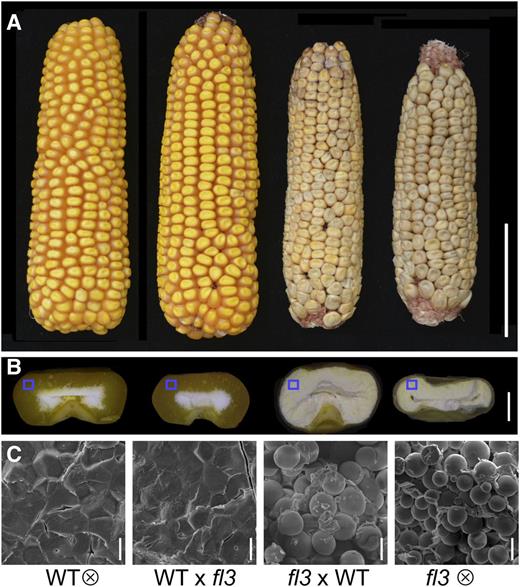
Kernel Phenotypes and Scanning Electron Microscopy of Starch Granules in WT, WT × fl3, fl3 × WT, and fl3.
(A) Ear phenotypes of WT, WT × fl3, fl3 × WT, and fl3. Bar = 5 cm.
(B) Kernel phenotypes of WT, WT × fl3, fl3 × WT, and fl3. Bar = 2 mm.
(C) Scanning electron microscopy of starch granules in the areas, as indicated in (B). Bars = 10 µm.
Endosperm-Filling Defects in fl3
As fl3 seeds are much lighter than wild-type seeds, we measured their kernel weight, storage carbohydrates, and proteins. The 100-kernel weight of fl3 was only 9.30 g, which is 58.9% lower than that of the wild type (22.65 g) (Figure 2A). Although the starch content based on dry seed flour was not significantly different in fl3 (Figure 2B), the dry weight of the seed is drastically reduced and therefore the starch content per seed is substantially reduced. The content of its soluble sugars was increased (Figure 2C). The levels of the main storage protein zeins were clearly decreased compared with those of the wild type during the course of endosperm development (Figures 2D and 2E), but the level of non-zein proteins was compensatorily increased. Proteome rebalancing resulted in the total protein content of fl3 being only 10% lower than that of the wild type (Figure 2D).
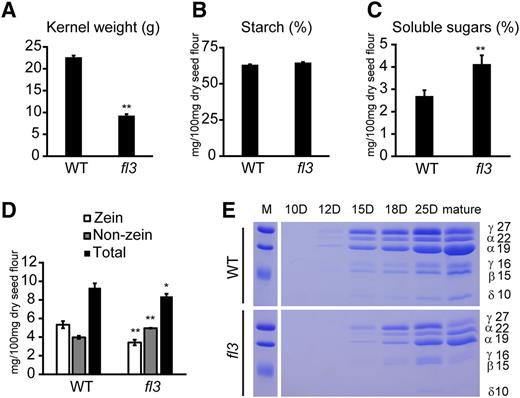
Storage Carbohydrates and Proteins in the Wild Type and fl3.
(A) The 100-kernel weight of wild type and fl3.
(B) The content of starch in wild type and fl3.
(C) The content of soluble sugars in wild type and fl3.
(D) The contents of proteins in wild type and fl3. Error bars in (A) to (D) represent the sd of five independent replicates. *P < 0.05 and **P < 0.01.
(E) SDS-PAGE analysis of zein in developing endosperms of wild type and fl3. D, days after pollination. The size of each band is indicated by numbers besides it. M, protein markers from top to bottom correspond to 25, 20, and 15 kD.
To further examine the reduction in kernel weight, we investigated endosperm development (Figure 3). Six days after pollination (DAP) when endosperm cells are undergoing cell differentiation and division, the fl3 and wild-type endosperms are both surrounded by the nucellus and their embryos remain attached to the suspensor. The endosperm and embryo in fl3 are morphologically normal but smaller compared with the wild type (Figures 3A and 3F). At 10 DAP when endosperm filling begins, both the fl3 endosperm and embryo are smaller compared with the wild type (Figures 3B and 3G), indicating that the fl3 seed develops more slowly than that of the wild type. At 18 DAP, when the storage products are actively synthesized in the endosperm, light microscopy observations clearly demonstrated that the fl3 cells were filled with dramatically fewer and smaller starch granules compared with the wild type (Figures 3C and 3H). Furthermore, transmission electron microscopy revealed that the number and size of protein bodies, organelles for the storage of zein proteins, were drastically reduced in the fl3 endosperm (Figures 3D and 3I). We also observed that the structure of the mitochondria was altered in fl3. Wild-type mitochondria had dense inner membranes, while mutant fl3 mitochondria showed a poorly developed membrane system with large internal spaces and abnormal cristae structures (Figures 3E and 3J).

Light and Transmission Electron Microscopy of Immature Endosperms at Different Developmental Stages the Wild Type and fl3.
(A) to (E) The wild type.
(F) to (J) fl3.
(A) and (F) Light microscopy of 6-DAP endosperms of the wild type (A) and fl3 (F). Bars = 100 µm.
(B) and (G) Light microscopy of 10-DAP endosperms of the wild type (B) and fl3 (G). Bars = 2 mm.
(C) and (H) Light microscopy of 18-DAP endosperms of the wild type (C) and fl3 (H). Bars = 50 µm.
(D) and (I) Transmission electron microscopy of 18-DAP endosperms of the wild type (D) and fl3 (I). Bars = 1 µm.
(E) and (J) Transmission electron microscopy of the magnified mitochondria in the wild type (E) and fl3 (J). Bars = 200 nm. En, endosperm; asterisk, embryo; SG, starch granule; PB, protein body; CW, cell wall; RER, rough endoplasmic reticulum; M, mitochondria.
Fl3 Encodes a PLATZ Family Protein
Fl3 was determined to be located on chromosome 8 by Oliver Nelson (http://mnl.maizegdb.org/mnl/50/89Nelson.pdf). The effect of the fl3 mutation on endosperm development is semidominant: Two doses of the mutant gene cause a full mutant phenotype, while one dose has no effect. To determine a correlation between the phenotype and genotype, we created two BC1F1 populations of (B73 × fl3) × B73 and (Mo17 × fl3) × Mo17 to fine-map this gene. Using 8800 individual kernels, the Fl3 gene was narrowed down to a region between the single nucleotide polymorphism (SNP) markers SNP5194 and SNP7666 (Figure 4A). Based on the B73 genome, these two markers span 2.9 Mb, where 19 genes have been annotated (Supplemental Data Set 1). Based on published RNA-seq data (Chen et al., 2014; Li et al., 2014), 14 genes could be excluded as candidates because their expression was not detected in the endosperm or embryo. Among the five expressed genes, GRMZM2G006585 is the only gene that is specifically and highly expressed in the endosperm. Because the striking mutant phenotype of fl3 is only observed in the endosperm not in other plant organs, it was thought that GRMZM2G006585 may be the candidate gene.
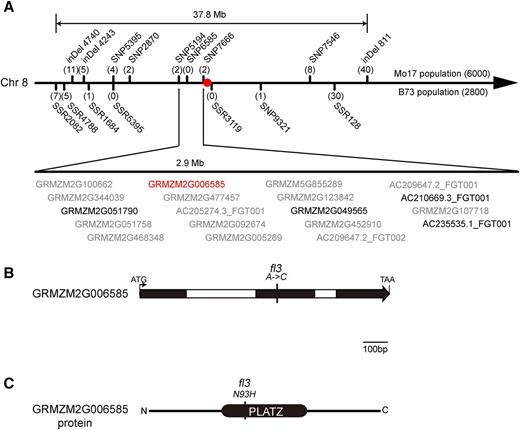
Map-Based Cloning of fl3.
(A) Fine-mapping of fl3 using the BC1F1 populations of (B73 × fl3) × B73 and (Mo17 × fl3) × Mo17 with 2800 and 6000 individuals, respectively. The numbers under each bar indicate the number of recombinants between fl3 and the molecular marker. The red dot indicates the centromere. Between two markers SNP5194 and SNP7666, 19 genes were annotated. Gene IDs in gray indicate that they are not expressed in the endosperm. Gene IDs in black indicate that they are expressed in the endosperm. The gene ID in red indicates that it is specifically expressed in the endosperm.
(B) A diagram showing the GRMZM2G006585 gene structure and the A/C substitution in the second exon in fl3.
(C) A diagram showing the GRMZM2G006585 protein structure and the Asn to His replacement in the PLATZ domain in fl3.
GRMZM2G006585 is composed of three exons and two introns. It is predicted to encode a 214-amino acid protein belonging to the PLATZ family. Therefore, the GRMZM2G006585 gene including its promoter region (662 bp upstream from the ATG start codon), three exons, and two introns from the wild type and mutant fl3, were amplified and sequenced. The wild type and mutant fl3 share an identical GRMZM2G006585 gene sequence except for an A/C substitution in the second exon (designated SNP6585; Figure 4A), which results in the replacement of Asn with His in the PLATZ domain (Figures 4B and 4C). We then amplified and sequenced the gene from 136 maize inbred lines and identified nine SNPs and one deletion in total, including four SNPs and the deletion in the promoter, one SNP in the second intron, and four SNPs in the third exon (Supplemental Figure 2A). Based on these polymorphisms, the GRMZM2G006585 gene can be classified into eight haplotypes in the analyzed population (Supplemental Figure 2B and Supplemental Data Set 2). The DNA sequence from −350 bp in the promoter to the entire second exon where the mutation occurs in fl3 was identical in all inbred lines. To further examine the GRMZM2G006585 protein, we performed a phylogenetic analysis. Public databases (National Center for Biotechnology Information [NCBI]) were searched using BLASTP with the GRMZM2G006585 amino acid sequence as a query and 19 closely related homologous sequences (identity >50%) were obtained. Sequence alignment showed that all 20 proteins contain a highly similar PLATZ domain (Supplemental Figure 3). Subsequently, we constructed a neighbor-joining phylogenetic tree of the 20 proteins (Supplemental File 1 and Supplemental Figure 4), which were grouped into two clades. Clade 1 contained 10 proteins from monocots, which shared >50% sequence identity with maize FL3, while Clade 2 included two proteins from Poaceae and eight proteins from dicots, which shared 40 to 50% sequence identity with FL3. Clade 1 contained FL3 and its homologs from other cereal species, suggesting that they play a conserved role in cereal endosperm development.
To confirm that this mutation is responsible for the floury endosperm phenotype, the high II B × A lines (see Methods) were transformed with the fl3 mutant gene driven by the 27-kD γ-zein gene promoter. Five transgenic events were recovered, and all self-pollinated ears segregated mutant seeds with a similar fl3 phenotype at a 3:1 ratio. Two representative self-pollinated ears (fl3-OE1 and fl3-OE6) are shown in Figure 5A, and two floury and normal seeds were indicated by arrows and asterisks, respectively. PCR amplification with a primer pair that flanks the 27-kD γ-zein gene promoter and the fl3 gene demonstrated that all floury seeds displayed the expected band, while the normal seeds did not (Figure 5A). Both crosses of WT × fl3-OE6/+ and fl3-OE6/+ × WT segregated floury seeds at a 1:1 ratio (Figure 5B), confirming that the A/C substitution in GRMZM2G006585 is a dominant rather than loss of physiological function mutation. The transgenic fl3 mutation caused the mutant phenotype in a dominant rather than a semidominant fashion, suggesting that the replacement of the fl3 promoter may affect its genetic behavior. The fl3 and Fl3 genes were expressed in rice (Oryza sativa) driven by a rice glutelin gene promoter, and only the mutant gene resulted in a similar floury phenotype in the transgenic seeds (Supplemental Figure 5), indicating that the function of Fl3 is conserved in cereal endosperm development.
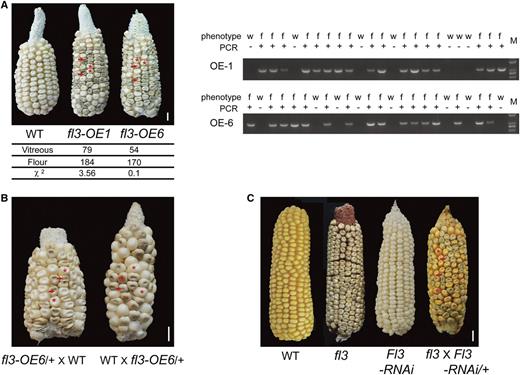
Transgenic Confirmation of fl3.
(A) Ear phenotypes of the transgenic maize expressing fl3. Bar = 5 cm. Upper left, ear phenotypes. The floury and normal seeds on the self-pollinated cobs are indicated by arrows and asterisks; lower left, statistical analysis of the kernel phenotype from two independent transgenic maize lines; right, PCR analysis of transgenic maize kernels. Forty-eight randomly selected kernels from two transgenic T0 lines were phenotyped and analyzed by PCR. The upper line indicates the phenotype of each kernel and the lower line indicates the PCR amplification of the corresponding kernel. w, the wild type; f, the floury phenotype.
(B) Reciprocal crosses of the wild type and fl3-OE6/+. The floury and normal seeds are indicated by arrows and asterisks.
(C) Rescue of fl3 with the Fl3-RNAi. In the cross of fl3 × Fl3-RNAi/+, the progeny that inherited the RNAi were restored to a normal phenotype as indicated by asterisks, whereas those without the RNAi remained floury as indicated by arrows.
To further confirm that the correct fl3 gene was cloned, we used the clustered regularly interspaced short palindromic repeats (CRISPR)/Cas9 technology to knock out this gene. Two independent events were recovered, both bearing a single nucleotide (T) insertion in the second exon located +588 bp from the ATG start codon, leading to a frame shift in the coding region (Supplemental Figure 6A). However, neither of the two null fl3 mutants exhibited a floury phenotype (Supplemental Figure 6A). This could be explained by functional redundancy of FL3 and some other PLATZ members in the maize endosperm, which are able to complement the null but not the dominant mutant allele of the Fl3 gene. We then asked if the floury phenotype in fl3 is caused by the dominant mutation in GRMZM2G006585, suppression of its expression should overcome the mutant phenotype. Rescue of a dominant mutant with RNAi has been successfully applied to Mucronate1, another maize floury mutant (Wu and Messing, 2010a). Seven Fl3-RNAi events were generated, and all exhibited silenced expression of Fl3. Three representative events were shown in Supplemental Figure 6B. Consistent with the above observation in the null mutants (Supplemental Figure 6A), none of these Fl3-RNAi mutants exhibited the floury phenotype (Supplemental Figure 6B). However, when a homozygous fl3 was crossed with a heterozygous Fl3-RNAi (Fl3-RNAi/+), half of the progeny inherited the RNAi and were restored to a normal phenotype, whereas the other half without the RNAi remained floury (Figure 5C). Theses genetic data clearly show that GRMZM2G006585 is the Fl3 gene.
Fl3 Is Specifically Expressed in Starchy Endosperm Cells
According to the maize eFP browser (http://www.maizegdb.org/gene_center/gene/GRMZM2G006585), the spatial expression pattern of Fl3 was restricted to the endosperm. RNA-seq analysis of the maize seed transcriptome revealed that Fl3 is highly expressed in the endosperm but is remarkably low or not expressed in other tissues (Chen et al., 2014; Li et al., 2014). Indeed, real-time PCR confirmed its endosperm specificity. The expression of Fl3 began at 6 DAP, reached a peak at 12 DAP, and declined thereafter (Figure 6A).
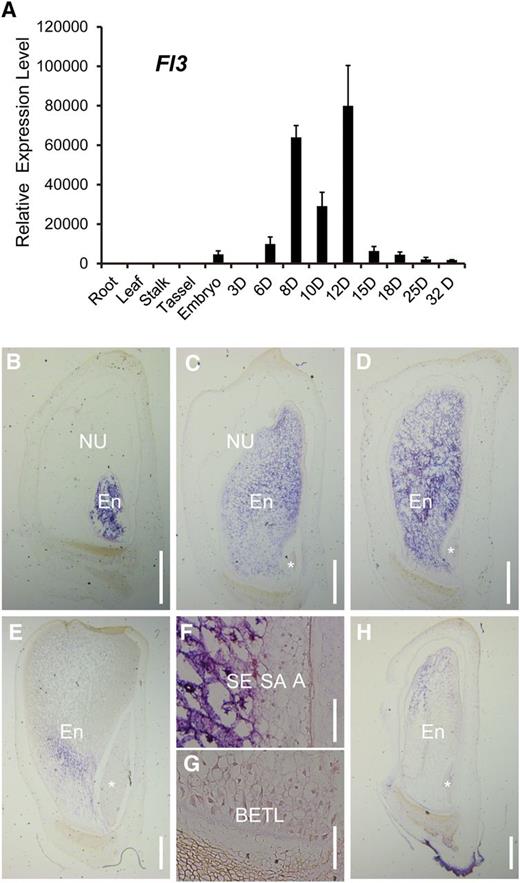
The Expression Pattern of Fl3.
(A) Quantitative RT-PCR analysis of Fl3 in root, leaf, stalk, tassel, embryo, and developing endosperms from 3 to 32 DAP. All expression levels are normalized to Actin. Three replicates for each sample were made and are illustrated as ±sd.
(B) to (E) RNA in situ hybridization of Fl3 at 6 (B), 8 (C), 12 (D), and 16 DAP (E). Longitudinal sections of wild-type kernels from 6 to 16 DAP were hybridized with an antisense RNA probe of Fl3. The positive signals (blue violet) of Fl3 were only observed in the starchy endosperm.
(F) and (G) RNA in situ hybridization of Fl3 at 12 DAP showing no expression in the aleurone and a few outmost starchy endosperm cell layers (F) and BETL (G).
(H) No signal was seen when a 12-DAP kernel section was hybridized with a sense probe.
NU, nucellus; En, endosperm; asterisk, embryo; SE, starchy endosperm; SA, subaleurone ; A, aleurone. Bars = 1 mm in (B) to (E) and (H) and 100 μm in (F) and (G).
Because the endosperm is composed of four cell types, we performed RNA in situ hybridization with an Fl3 antisense probe to investigate the spatial distribution of Fl3 in the endosperm. Consistent with the real-time PCR result, the hybridization signal was first detected in 6-DAP endosperm (Figure 6B). The signal increased thereafter and reached a maximum at 12 DAP (Figures 6C and 6D). In contrast, no signal was observed when a 12-DAP kernel section was hybridized with the Fl3 sense probe (Figure 6H). At 16 DAP, the Fl3 mRNA signal decreased remarkably (Figure 6E), but some expression in the more basal area of the endosperm could still be detected. Fl3 was not detected in the pericarp, nucellus, or embryo during seed development. Furthermore, our results revealed that Fl3 appears to be exclusively expressed in starchy endosperm cells (Figure 6D). Besides the aleurone cell layer and BETL, we also didn't detect the signal in the few outmost layers of starchy endosperm cells adjacent to the aleurone (Figures 6F and 6G).
Fl3 Is Regulated by Genomic Imprinting
The transgenic and endogenous fl3 genes deviated in genetic behavior, the former being dominant (causing the floury phenotype whether inherited from the female or male parent; Figure 5A) and the latter being semidominant (Figure 1A). This discrepancy in the penetrance of the fl3 phenotype may result from the replacement of the fl3 promoter that alters its native genetic regulation and therefore expression. The endosperm is triploid: two doses of the nuclear genome come from the female and one from the male. If two allelic genes from the female and male are equally expressed in the endosperm, their transcript ratio is expected to be 2:1. Taking advantage of SNP6585 (Figures 4A and 4B), we were able to discriminate the wild-type (Fl3) and mutant (fl3) transcripts when they were amplified from the WT × fl3 cross. However, when calculating the number of cDNA sequences, we found that the fl3 transcript was dramatically underrepresented (only 3% of the total sequences) (Figure 7A). It is possible that the fl3 mutation affects mRNA stability. However, when the wild type was used as the male, the expression of Fl3 was strikingly lower than expected (only 18.8% of the total sequence lower than the expected 33.3%). These data indicate that the allelic expression of Fl3 or fl3 depends on the parent of origin.
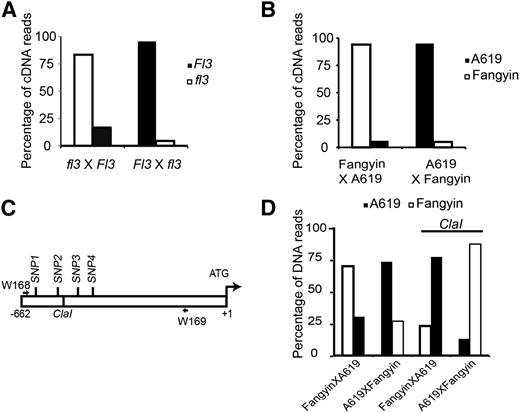
Gene Imprinting of Fl3.
(A) Allelic expression analysis of Fl3 and fl3 in their reciprocal crosses.
(B) Allelic expression analysis of Fl3-A619 and Fl3-Fangyin in their reciprocal crosses.
(C) Diagram showing the polymorphisms between the Fl3-A619 and Fl3-Fangyin promoters. W168 and W169 are a pair of primers designed to amplify the allelic promoters of Fl3-A619 and Fl3-Fangyin that comprise four SNPs and a ClaI site.
(D) Differential DNA methylation in the Fl3 promoters of two parents in the endosperm. ClaI is a DNA methylation-sensitive restriction enzyme. Without ClaI digestion, the Fl3 promoter sequences amplified from the female and male alleles were ∼2:1, consistent with their gene dosage contribution in the endosperm. With ClaI digestion, the sequences were more amplified from the male allele, which has a higher DNA methylation in its promoter.
To investigate whether the parent-of-origin regulation of Fl3 is allele or gene specific, we examined the transcript ratios of Fl3-A619 and Fl3-Fangyin in their reciprocal crosses, which belong to haplotypes 1 and 3 (Supplemental Figure 2B and Supplemental Data Set 2), respectively. Indeed, regardless of what allele was used, the expression of the allele that came from the male parent was dramatically suppressed (Figure 7B), indicating that the parent-of-origin regulation of Fl3 is gene specific.
As Fl3 is specifically expressed in the endosperm and is regulated by the parent of origin, it may be an imprinted gene. Differential DNA demethylation in the promoters of two parent genes plays an important role in genomic imprinting (Gutiérrez-Marcos et al., 2006; Feil and Berger, 2007; Haun et al., 2007; Villar et al., 2009; Costa et al., 2012). The Fl3 promoter contains a ClaI restriction endonuclease site (ATCGAT) located –564 bp from the ATG start codon (Figure 7C). Because this enzyme is DNA methylation-sensitive, if the male allele is methylated and female allele is unmethylated, the male allele will remain intact following the ClaI digestion. The Fl3-A619 and Fl3-Fangyin alleles also contain polymorphisms in their promoters, which enabled us to distinguish between the two (Figure 7C). Genomic DNAs were extracted from the endosperms of Fangyin × A619 and A619 × Fangyin. A primer pair (W168 and W169), shown in Figure 7C, was able to specifically amplify the Fl3 promoter fragment comprising the ClaI recognition site and the polymorphic sites. This fragment was amplified from both undigested and digested (by ClaI) endosperm genomic DNAs. When the fragments amplified from the undigested DNAs were cloned and sequenced, the ratio of sequences from the female and male was ∼2:1, consistent with their dosage contribution in the endosperm (Figure 7D). However, when the genomic DNAs were digested with ClaI, the sequences were primarily amplified from the male allele, indicating that the Fl3 promoter from the male has a higher methylation rate than that from the female in the endosperm. These results indicate that Fl3 is an imprinted gene.
FL3 Binds Nonspecifically to A/T-Rich Sequences
To determine its subcellular localization, FL3 and fl3 proteins were fused to the enhanced green fluorescent protein (eGFP) and transiently expressed in Nicotiana benthamiana leaves. Both fused proteins were localized in the nucleus, consistent with FL3 being a nuclear protein (Supplemental Figure 7). The Asn-to-His replacement in fl3 did not influence its localization to the nucleus.
PLATZ family proteins are a novel class of plant-specific zinc-dependent DNA binding proteins and are classified as transcription factors (TFs) in plant TF databases (Nagano et al., 2001); however, none have yet been genetically characterized to regulate specific genes in plants. PLATZ1 isolated from pea (Pisum sativum) binds nonspecifically to A/T-rich sequences and is believed to function in transcriptional repression (Nagano et al., 2001). To investigate the DNA element recognized by FL3, we performed a random binding site selection experiment. Using the His-FL3 protein bound to Ni-NTA magnetic agarose beads, the FL3 binding DNA element was screened from a pool of DNA fragments, each bearing a 32-base random sequence in the middle. After five rounds of binding and amplification, 22 sequences were found to contain the GAA(A/T/G)(A/G) sequence in all 72 sequences recognized by FL3. This finding indicates that the FL3 protein preferentially targets any sequence that is rich in A/T bases, consistent with previous findings in PLATZ1.
Using the yeast GAL4 system, we determined that FL3 has no transcriptional activation ability (Supplemental Figure 8), suggesting that FL3 may not act as a typical transcription factor as do O2 and PBF, which activate the expression of zein genes through recognizing specific DNA motifs (Li et al., 2015a; Zhang et al., 2015; Yang et al., 2016).
FL3 Interacts with RPC53 and TFC1 in the RNA Polymerase III Machinery
As Fl3 is highly expressed between 8 and 12 DAP in the endosperm, we constructed a yeast two-hybrid library using 10-DAP endosperms; 11 candidate genes were obtained (Supplemental Table 1). All proteins encoded by these genes were tested individually and only the RNAPIII subunit RPC53 encoded by GRMZM2G025294 was confirmed to participate in a protein-protein interaction with FL3 (Figure 8A). A bimolecular luciferase complementation (BiLC) experiment was also performed, in which RPC53 and FL3 were fused to the C- and N-terminal domains of luciferase (CLUC and NLUC, respectively). Cotransfection of FL3-NLUC and RPC53-CLUC produced strong luciferase activity, while FL3-NLUC and the corresponding empty construct CLUC failed to produce a visible signal (Figure 8B). These results demonstrate that FL3 interacts with RPC53. Deletion analysis revealed that the PLATZ domain is required for this interaction (Figures 8C and 8D).
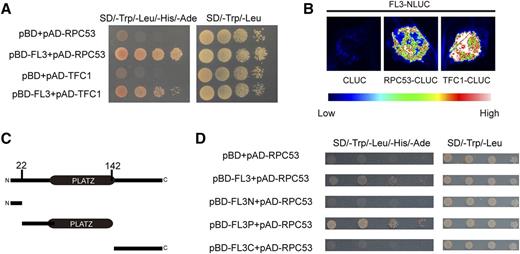
FL3 Interacts with RPC53 and TFC1.
(A) Yeast two-hybrid assay showing that FL3 and RPC53/TFC1 interacts.
(B) BiLC showing that FL3 and RPC53/TFC1 interacts. Fluorescence signal intensities represent their interaction activities.
(C) A diagram showing different deletions in FL3 designed for protein-protein interaction analysis.
(D) Yeast two-hybrid assay showing that the PLATZ domain is required for protein-protein interaction with RPC53. The full-length, N terminus, PLATZ domain region, and C terminus of FL3 were tested for their interaction with RPC53.
In (A) and (D), the yeast cultures with four different dilutions (100, 10−1, 10−2, and 10−3) were grown on the SD medium lacking Trp, Leu, His, and adenine (left panel) and lacking Trp and Leu (right panel, as control), respectively. AD, activating domain; BD, binding domain.
RNAPIII is responsible for the transcription of short noncoding RNAs, such as tRNAs and 5S rRNA (Turowski and Tollervey, 2016). In addition to RNAPIII, TFIIIA, TFIIIB, and TFIIIC are also part of the RNAPIII transcription machinery, forming the preinitiation complex and recruiting RNAPIII to the promoter for transcription initiation (Dieci et al., 2013; Male et al., 2015; Turowski and Tollervey, 2016). Therefore, the maize homolog of the TFIIIC subunit TFC1 (GRMZM2G053008) was cloned. Yeast two-hybrid and BiLC experiments revealed that FL3 also interacts with TFC1 as well, indicating that FL3 plays an important role in the RNAPIII transcription machinery (Figures 8A and 8B).
The Expression of tRNAs and the 5S rRNA Is Reduced in the fl3 Endosperm
If our speculation regarding the function of FL3 in RNAPIII transcription machinery is correct, the biogenesis of tRNAs and 5S rRNA would be affected in the fl3 endosperm. Indeed, the transcript level of 5S rRNA was decreased by more than 1-fold (Figure 9). In contrast, the levels of 18S and 25S rRNAs that are transcribed by RNAPI were not affected, indicating that the function of RNAPIII is specifically compromised by the fl3 mutation. We then determined the levels of 17 tRNAs and found that 12 had a significant reduction in their expression. Remarkably, levels of six tRNAs (tRNA-Gly, tRNA-Lys, tRNA-Asn, tRNA-Ala, tRNA-Glu, and tRNA-Trp) were reduced by more than 2-fold (Figure 9 ). However, we also observed that the expression of three tRNAs was not affected and two were elevated (Figure 9), suggesting that the accumulation of tRNAs may be regulated by multiple steps.
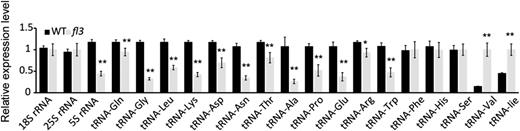
Quantitative RT-PCR Analysis of 17 tRNAs and 5S rRNA Genes in 12-DAP Endosperms of the Wild Type and fl3.
All expression levels are normalized to Actin. Three replicates for each sample were made and are illustrated as ±sd.
Dramatic Transcriptomic Alterations in the fl3 Endosperm
The fl3 mutation caused severe defects in endosperm development and a loss in kernel weight, likely through interrupting the function of RNAPIII. To investigate the downstream gene network affected by fl3, the endosperms of wild-type and fl3 seeds were harvested at 10 DAP, the time point that represents the high expression of Fl3 and the initiation of storage product synthesis, for transcriptome analysis by RNA-seq. Three biological replicates of the wild type and fl3 were sequenced and determined to be clustered into one group (Figure 10A). Approximately 87% of raw reads from each sample were mapped to the annotated gene-coding regions. On average, 30,226 expressed genes were expressed for each sample; 7662 upregulated and 7516 downregulated genes were detected in the mutant fl3 compared with the wild type (Figure 10B; Supplemental Data Set 3). Gene Ontology (GO) terms associated with pathways such as translation, the ribosome, misfolded protein responses, and nutrient reservoir activity were preferentially enriched in the downregulated genes (Figure 10C; Supplemental Data Set 4). For example, the storage protein zein genes and starch biosynthetic pathway genes related to nutrient reservoir activity were significantly enriched in the downregulated genes. The expression of 22- and 19-kD α-zein genes was reduced by 95%, while that of GBSSI, Sh1, Bt2, and Sh2 was reduced by 79, 58, 52, and 40%, respectively (Supplemental Table 2). We also performed quantitative RT-PCR to validate the expression of these individual genes in the wild type and fl3 and found the two data sets correlated very well (Supplemental Figure 9). In general, these results demonstrate that the endosperm transcriptome is dramatically altered by the fl3 mutation, suggesting a critical role for the FL3-mediated modulation of RNAPIII in endosperm development and filling.

Global Transcriptome Analysis Based on RNA-Seq Data in 10-DAP Endosperms of the Wild Type and fl3.
(A) Principal component analysis.
(B) The numbers of differentially expressed genes in the fl3 endosperm compared with the wild type.
(C) GO classification for genes with downregulated expression in the fl3 endosperm compared with the wild type.
DISCUSSION
A Dominant Mutation in the Maternally Expressed Imprinted Gene fl3
A dominant mutation causes a mutant phenotype in heterozygotes. Because the endosperm is triploid, the differential contribution of gene dosage by the female and male may lead to a dominant mutation behaving as semidominant. The fl1 and fl2 phenotypes are dosage-dependent (Coleman et al., 1997; Holding et al., 2007). However, the dosage effect is not likely the reason that leads to the semidominant behavior of fl3. Two doses of the mutation resulted in a nearly identical fl3 phenotype, while one dose produced a completely normal phenotype (Figure 1). When heterozygous plants (Fl3/fl3) were self-pollinated or pollinated by a wild-type plant, the resulting progeny segregated the mutant and normal seeds at an equal ratio (Supplemental Figure 1). Allelic expression analysis revealed that the maternal and paternal alleles of fl3 are not equivalent in expression. When fl3 was inherited from pollen, its expression was dramatically suppressed (Figure 7A). It is unlikely that this differential expression resulted from the instability of fl3 mRNA, as the wild-type Fl3 allele was also suppressed, as long as it underwent male transmission. A619 and Fangyin are two inbred lines from the US and China, respectively. Taking advantage of SNPs in Fl3 coding regions, we were able to discriminate the two Fl3 alleles in the reciprocal crosses of A619 and Fangyin (Supplemental Figure 2 and Supplemental Data Set 2). Regardless of what Fl3 allele came from the male, its expression was suppressed (Figure 7B), indicating that the parent-of-origin-biased regulation of Fl3 expression is gene specific. In the triploid endosperm, the maternal alleles of imprinted genes such as Meg1, Fie1, Fie2, and Mez1 are preferentially expressed relative to the paternal alleles (Gutiérrez-Marcos et al., 2004, 2006; Haun et al., 2007; Costa et al., 2012). DNA methylation and demethylation affect allelic expression, resulting in gene imprinting (Feil and Berger, 2007; Haun et al., 2007; Köhler et al., 2012). Hence, we used a DNA methylation-sensitive restriction enzyme, ClaI, to determine the DNA methylation status in the Fl3 promoter. The paternal allele had a higher level of methylation in the promoter than the maternal allele (Figures 7C and 7D), indicating that transcriptional suppression of the paternal Fl3 allele is regulated by DNA methylation-mediated epigenetic control; thus, Fl3 is a maternally expressed imprinted gene.
Imprinting or genomic imprinting refers to an epigenetic modification of maternally or paternally inherited alleles that lead to their differential expression in a parent-of-origin-dependent manner (Kermicle, 1970). Most characterized examples of imprinted gene expression in plants have been documented in the triploid endosperm (Köhler et al., 2012). The genome-wide discovery of imprinted genes in the maize endosperm through RNA-seq analysis was based on allelic polymorphisms between inbreds B73 and Mo17, which identified 100 to 200 protein-coding genes that were regulated by genomic imprinting (Waters et al., 2011; Zhang et al., 2011). Fl3 has not been identified as an imprinting gene, because this gene has no allelic polymorphism between the two examined inbred lines (Supplemental Data Set 2), suggesting that the number of imprinted genes may be underestimated in the maize genome.
FL3 as a New Regulator in RNAPIII Transcription Machinery
In eukaryotes, three major RNAPs are employed to accomplish the transcription of different classes of RNA. RNAPI is responsible for the transcription of large rRNAs (in plants being 18 and 25S rRNAs); RNAPII synthesizes mRNAs of protein-coding genes; and RNAPIII transcribes many short noncoding RNAs, such as tRNAs, 5S rRNA, and 7SL RNA (Turowski and Tollervey, 2016). Additionally, plant-specific RNAP IV and V transcribe small interfering RNAs for RNA-mediated DNA methylation (Zhou and Law, 2015). Transcriptional regulation of protein-coding genes by RNAPII has been extensively studied in yeast, animals, and plants. In contrast, the regulation of other RNA species expression is considerably less understood.
RNAPIII is a multisubunit enzyme that functions with three transcription factors (TFIIIA, TFIIIB, and TFIIIC) (Acker et al., 2013). A large number of studies have focused on the genetic and functional characterization of RNAPIII components in various organisms. In zebra fish, a mutation resulting from a premature stop codon at Rpc9 locus impairs hematopoietic stem and progenitor cell maintenance (Wei et al., 2016). The nrpc7 mutation occurs in a gene encoding the Arabidopsis thaliana ortholog of the yeast RNAPIII subunit RPC25, which causes altered expression of a number of RNA molecules and a pleiotropic development abnormality in plant morphogenesis (Johnson et al., 2016). Meanwhile, several reports have demonstrated that the fine modulation of RNAPIII transcription mediated by key factors is also essential for normal organism development. Trypanosoma brucei cell growth is arrested due to ablation of the TFIIIB subunit TbBRF1 by RNAi (Vélez-Ramírez et al., 2015). The suppression and CRISPR-mediated deletion of Brf1 cause several neurodevelopmental phenotypes in zebra fish (Borck et al., 2015). Additionally, the mediator complex member MED4 was genetically characterized in Arabidopsis with aborted seed development in its homozygous mutant. This protein was identified as a common interactor with RNAPII component TFIIF2-2 and RNAPIII component RPC53 in rice (Li et al., 2015b). Despite these findings, the factors and mechanisms that underlie the modulation of RNAPIII transcription in plants remain obscure.
Here, we found that FL3 plays an important role in the modulation of RNAPIII transcription machinery in the maize endosperm. Our results show that FL3 interacts with the RNAPIII subunit RPC53 and the TFIIIC subunit TFC1 in vitro and in planta (Figure 8). The biogenesis of many tRNAs and 5S rRNA was significantly reduced by the dominant fl3 mutation (Figure 9). These experiments revealed that FL3 is a new factor of the RNAPIII transcription machinery and is involved in the modulation of RNAPIII transcription (Figure 11). The 5S rRNA is required for ribosome assembly and tRNAs function in the transfer of amino acids to the protein translation machinery. The levels of their RNA transcripts are critical to maintain efficient running of the protein synthesis system. One could envision that impaired biogenesis of tRNAs and 5S rRNA would negatively affect storage reserve accumulation and other processes related to protein synthesis in the endosperm (Figure 11). Indeed, transcript levels of the storage protein zein genes and many starch synthetic pathway genes were remarkably decreased in fl3 compared with the wild type (Supplemental Table 2 and Supplemental Figure 9). The protein bodies and starch granules, which are the organelles for storage of zeins and starch, were dramatically fewer and smaller in fl3 (Figures 3C, 3D, 3H, and 3I), consistent with its much lighter kernel weight compared with the wild type (Figure 2A). Apparently, the mechanism by which FL3 regulates the endosperm development and storage reserve filling is different from any of what have discovered in other opaque and floury mutants (Wu and Messing, 2017).
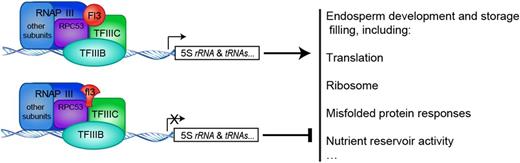
A Proposed Model for FL3/fl3 Controlling the Storage Filling in the Maize Endosperm.
The PLATZ Family in Plants
In this study, the fl3 gene was cloned and determined to encode a PLATZ family protein (Figure 4C; Supplemental Figure 3). PLATZ1 was first identified as a novel zinc-dependent DNA binding protein in peas (Nagano et al., 2001). According to a previous report, no paralogous sequence to pea PLATZ1 has been found outside of the plant kingdom. Similarly, homologs of FL3 have not yet been retrieved in yeast or animals using BLAST (Supplemental Figure 3), consistent with the PLATZ proteins being plant specific. It is likely that the FL3 homologs have diverged between dicots and monocots (Supplemental Figure 4). Expression of the maize fl3 mutant gene in rice results in a similar floury phenotype in the transgenic seeds (Supplemental Figure 5), indicating the functional conservation of FL3 homologs in cereals.
Fl3 is specifically expressed in maize endosperm starchy cells (Figure 6), whereas components of RNAPIII and its associated transcription factors are ubiquitously expressed. Their expression patterns are paradoxical and therefore raise the following question: If FL3 is involved in the modulation of the RNAPIII transcription complex through protein-protein interactions with RPC53 and TFC1 in the endosperm, why is it not required in other tissues? One explanation may be that the highly abundant synthesis of tRNAs and 5S rRNA is metabolically demanding in starchy endosperm cells, where starch and proteins are actively produced and stored. The FL3-mediated modulation of RNAPIII transcription may be required for the efficient production of these small RNAs. As tRNAs and 5S rRNA are much more stable than mRNAs, Fl3 may not need to continuously be expressed during the filling stage. The plateau of its expression (between 8 and 12 DAP) coincides with period of the endosperm filling initiation. Another explanation may be functional redundancy of FL3 with other members in the PLATZ family, which has been predicted to contain 15 members (http://grassius.org/family.php?family=PLATZ&species=Maize). This speculation is supported by the observation that the knockout and knockdown mutations of Fl3 didn't cause the floury phenotype (Supplemental Figure 6). To test this hypothesis, we selected six additional PLATZ genes that are not specifically expressed in the endosperm and found that three (PLATZ3/GRMZM2G094168, PLATZ5/GRMZM2G131280, and PLATZ11/GRMZM2G004548) interact with RPC53 (Supplemental Figure 10). This finding indicates that many other PLATZ proteins modulate RNAPIII transcription in different tissues including the endosperm.
The Fl3 function can be explored due to the dominant mutation, an effective genetic approach to study a gene family with functional redundancy. Although the detailed mechanisms have not been known yet, the cloning of fl3 may contribute to our understanding of the functions of the newly identified PLATZ protein family and mechanisms of the RNAPIII transcription modulation in plants. More information regarding FL3 and other PLATZ members will be discovered by creating combinational mutations by CRISPR/Cas9 in the future.
METHODS
Genetic Materials
The fl3 mutant was obtained from Maize Genetics Cooperation Stock Center under the accession number 805A. The homozygous normal seeds segregated from the heterozygous mutant were used as the wild type in this study. The 136 maize (Zea mays) inbred lines used for the Fl3 polymorphism study were provided by Jinsheng Lai at China Agricultural University. For maize transformation of fl3, the fl3 coding sequence was amplified from 10-DAP old endosperms and driven by the 27-kD γ-zein gene promoter, generating the construct P27-fl3. To silence the expression of the Fl3 gene, a 227-bp Fl3 cDNA fragment was amplified for Fl3-RNAi construction. To knock out Fl3 by CRISPR/Cas9, the guide RNA was synthesized by Sangon Biotech and cloned into the PsiI and XbaI sites between the maize U6 promoter and U6 terminator. The constructs were delivered into maize by Agrobacterium tumefaciens-mediated transformation. Hi-II F1 (B × A) (for P27-fl3 and fl3-RNAi constructs) and the inbred line C01 (for the fl3 CRISPR/Cas9 construct) immature embryos (1.5–2.0 mm) were dissected from the ears growing in the field at 11 DAP. Five, seven, and two positive events for the three constructs were recovered, respectively, following the standard transformation protocol (Frame et al., 2002). For rice (Oryza sativa) transformation, the fl3 and Fl3 coding sequences were cloned into pCAMBIA1300 plasmid and the promoter was replaced with a rice endosperm-specific glutelin1 promoter. The primers for making the constructs and for amplifying the target region by CRISPR/Cas9 are listed in Supplemental Table 3.
Measurement of Proteins, Soluble Sugars, and Starch
At least 20 mature kernels of the wild type and fl3 were ground into fine flour using a coffee grinder. One hundred milligrams of flour for each sample was used for measurement of proteins, soluble sugars, and starch. Protocols for these measurements have been described (Liu et al., 2016; Zhang et al., 2016). SDS-PAGE was performed to analyze the accumulation patterns of zein and non-zein proteins in the wild type and fl3.
Light, Transmission, and Scanning Electron Microscopy
To prepare semithin sections, immature seeds were soaked in 50% FAA and then vacuumed overnight in a low-temperature (4°C) circumstance. Later, seeds were treated with gradient concentrations of ethanol and embedded in epoxide resin for producing 2- to 3-μm sections. After 42°C treatment and toluidine blue staining, sections were sealed for observation under Leica DM2500. We chose mature seeds and 18-DAP immature seeds to perform scanning electron microscopy and transmission electron microscopy, respectively. Protocols have been described (Wu and Messing, 2010b; Zhang et al., 2016).
Map-Based Cloning of fl3
Because fl3 behaves as a semidominant allele, we used BC1F1 populations to fine map the mutant gene. Two BC1F1 populations were generated, (B73 × fl3) × B73 and (Mo17 × fl3) × Mo17, which segregated mutant and normal seeds in an equal ratio, clearly correlated to their genotypes. After phenotyping, all mutant and normal seeds were germinated for exaction of DNA. Molecular markers were developed for fine mapping as shown in Figure 4A. The primers are listed in Supplemental Table 3.
Phylogenetic Analysis
Multiple sequences were aligned using ClustalX software with default parameters. Homologs of FL3 in various species were retrieved from NCBI BLAST. The phylogenetic tree was constructed using the MEGA program version 7 (www.megasoftware.net) and the neighbor-joining method, whose parameters are Poisson correction, pairwise deletion, and bootstrap (1000 replicates; random seed).
RNA Extraction and Real-Time PCR Analysis
Total RNA was extracted from immature endosperms and other tissues using TRIzol reagent (Invitrogen) and then purified with the RNeasy Mini Kit after DNaseI digestion (Qiagen). Reverse transcription was conducted with Super Script III First Strand Kit (Invitrogen). Quantitative RT-PCR was performed with SYBR Green I. The comparative CT method (ΔΔCT method) was employed for the relative quantification of gene expression, and the maize Actin gene was used as the reference. Statistically significant differences of expression levels of genes were calculated by Student's t test. All primers used in the study are listed in Supplemental Table 3.
RNA in Situ Hybridization
Wild-type kernels at four time points (6, 8, 12, and 16DAP) were used for RNA in situ hybridization as described in our previous work (Zhang et al., 2015). Tissue processing and in situ hybridization experiment using 10-mm sections and was observed with a Leica M165 FC.
Tobacco Subcellular Localization
The amplified Fl3 coding sequence was fused to a reporter gene encoding eGFP, which was then cloned into pCAMBIA1301 plasmid driven by the 35S promoter. Agrobacterium (strain GV3101) harboring this construct was infiltrated into 3-week-old Nicotiana benthamiana leaves using a needleless syringe. At least three replicates were performed. The eGFP signal was observed and imaged using a confocal microscope (LSM510; Zeiss).
Gene Imprinting Analysis
To analyze the differential allelic expression, the Fl3 coding sequences were amplified from cDNAs, which were reverse transcribed from 10-DAP endosperms of WT × fl3, fl3 × WT, A619 × Fangyin, and Fangyin × A619, respectively. The PCR products were ligated into the pEASY-Blunt Zero (Transgene) and transformed into the Escherichia coli strain DH5a. Positive colonies were sequenced for subsequent genotyping. At least 100 sequences were counted for each sample.
To analyze the differential DNA methylation in two allelic Fl3 promoters, genomic DNAs extracted from 10-DAP endosperms of A619 × Fangyin and Fangyin × A619 were treated with or without the DNA methylation-sensitive restriction enzyme ClaI. The Fl3 promoters were amplified from the treated and untreated DNAs of A619 × Fangyin and Fangyin × A619, respectively. The cloned fragments were sequenced and genotyped as described above. At least 100 sequences were counted for each sample.
Random Binding Site Selection
Random binding site selection was performed according to described procedures (Liu et al., 2008). Briefly, the 80-bp dsDNA probe pool with each piece containing a 32-bp random sequence at the center was incubated with the His-FL3 fusion protein that was bound to Ni-NTA magnetic agarose beads. After washing, the DNA bound to His-FL3 was eluted. The eluted DNA was amplified by PCR and incubated with His-FL3 fusion protein again. Five rounds of binding and amplification were performed before the eluted DNA was cloned into the vector pEASY-Blunt Zero (Transgene). Seventy-two independent clones were randomly selected for sequencing analysis.
Protein Interaction Experiments
For yeast two-hybrid assays, Fl3 CDS and other deleted Fl3 fragments were ligated to the pGBKT7 plasmid and then transformed into the yeast strain Y2HGold, while the Rpc53 and Tfc1 CDSs were inserted in pGADT7 plasmid and then transformed into the strain Y187. Maize homologs of RPC53 and TFC1 were retrieved through MaizeGDB BLAST (www.maizegdb.org) using Arabidopsis thaliana annotation information (www.arabidopsis.org). pGBKT7-FL3 was the bait for yeast two-hybrid screening, and the yeast library was produced by OE Biotechnology using 10-DAP endosperms. All yeast experiments were referenced in Clontech's Yeast Protocols Handbook.
To make constructs for BiLC experiments, coding sequences of Fl3, Rpc53, and Tfc1 were cloned into JW771 (N-terminal half of luciferase, NLUC) and JW772 (C-terminal half of luciferase, CLUC), respectively, yielding FL3-NLUC and RPC53/TFC1-CLUC constructs for the luciferase complementation image assay, following the protocol described elsewhere (Gou et al., 2011). Agrobacterium (strain GV3101) harboring the above constructs was infiltrated into 3-week-old N. benthamiana leaves using a needleless syringe. After growing for 72 h under the condition of 16 h of light and 8 h of dark, leaves were injected with 0.8 mM luciferin and the resulting luciferase signals were captured using Tanon-5200 system. These experiments were repeated at least three times with similar results. Quantitative analysis was performed using Image J software (http://rsb.info.nih.gov/ij/).
RNA-Seq Analysis
Total RNAs was extracted from 10-DAP old endosperms of the wild type and fl3 using TRIzol reagent (Invitrogen) and were then purified with the RNeasy Mini Kit (Qiagen). RNA-seq libraries were prepared according to the Illumina Standard library preparation kit. Illumina HiSeq 2500 was used as a platform for RNA-seq at Shanghai OE Biotech. FastQC combined with the NGS QC TOOLKIT v2.3.3 were used for quality control checks on raw sequencing data. Clean reads were aligned to the B73 reference genome (RefGen_v3) and the reference gene model data set (FGS 5b) using TopHat/Bowtie2 (ccb.jhu.edu/software/tophat/). The gene expression value was normalized as fragments per kilobase of transcript per million mapped reads (FPKM). A gene was considered expressed only when its FPKM value was greater than zero in the three biological replicates. The differentially expressed genes were produced with the threshold of a false discovery rate <0.05 by the DESeq Software Packages (bioconductor.org/). GO enrichment of DEGs was performed using R based on the hypergeometric distribution.
Accession Numbers
Sequence data from this study can be found in GenBank/EMBL data libraries under accession number NM_001152112 for fl3, XM_008669830 for Rpc53, XM_008663148 for Tfc1, and GSE95039 for RNA-seq data.
Supplemental Data
Supplemental Figure 1. Segregation of Vitreous and Floury Seeds in fl3/+ × B73 and Self-Pollinated fl3/+ Ears.
Supplemental Figure 2. The Fl3 Gene Structure and Polymorphic Variations in 136 Maize Inbred Lines.
Supplemental Figure 3. Amino Acid Sequence Alignment of FL3 and Its Homologs.
Supplemental Figure 4. Phylogenetic Analysis of FL3 and Its Homologs.
Supplemental Figure 5. Seed Phenotypes and Quantitative RT-PCR Analysis of Transgenic Rice Seeds Expressing the Maize Wild-Type Fl3 and Mutant fl3 Gene.
Supplemental Figure 6. Knockout and knockdown Mutants of Fl3.
Supplemental Figure 7. Subcellular Localization of FL3/fl3.
Supplemental Figure 8. Yeast Two-Hybrid Assay of the Transcriptional Activation Ability by FL3.
Supplemental Figure 9. Relative Expressions of the Starch Biosynthetic Pathway and Zein Genes in Wild-Type and fl3 10-DAP Endosperms Analyzed by the Quantitative RT-PCR.
Supplemental Figure 10. Other PLATZ Proteins Interact with RPC53.
Supplemental Table 1. Eleven Candidate Genes Identified with FL3 Bait through Yeast Two-Hybrid Screening.
Supplemental Table 2. The Differential Expression Genes in the Starch Biosynthetic Pathway and Zein Gene Family.
Supplemental Table 3. Primer List.
Supplemental Data Set 1. The Expression Patterns of 19 Candidate Genes.
Supplemental Data Set 2. The List of 136 Inbred Lines and Their Fl3 Allelic Types.
Supplemental Data Set 3. The Differential Expression Genes of fl3 Compared with the Wild Type.
Supplemental Data Set 4. GO Terms Enriched in the Downregulated Genes of fl3.
Supplemental File 1. Text File of the Alignment Corresponding to the Phylogenetic Analysis in Supplemental Figure 4.
Acknowledgments
This research was supported by the National Natural Science Foundation of China (91635303 to Y.W.), by the Chinese Academy of Sciences (XDPB0401 and XDA08020107 to Y.W.), by the Ministry of Science and Technology of China (2016YFD0100500), and by a Chinese Thousand Talents Program Grant (to Y.W.). We thank Xiaoshu Gao, Xiaoyan Gao, Zhiping Zhang, and Jiqin Li (Institute of Plant Physiology and Ecology, SIBS, CAS) for technical support.
AUTHOR CONTRIBUTIONS
Q.L., J.W., and Y.W. designed the research, analyzed the data, and wrote the manuscript. Q.L., J.W., J.Y., X.Z., X.X., C.L., M.F., Q.W., Z.Z., and Y.W. performed the research.
References
Author notes
These authors contributed equally to this work.
Address correspondence to [email protected].
The author responsible for distribution of materials integral to the findings presented in this article in accordance with the policy described in the Instructions for Authors (www.plantcell.org) is: Yongrui Wu ([email protected]).

Articles can be viewed without a subscription.