-
PDF
- Split View
-
Views
-
Cite
Cite
Masaki Niwa, Yasufumi Daimon, Ken-ichi Kurotani, Asuka Higo, José L. Pruneda-Paz, Ghislain Breton, Nobutaka Mitsuda, Steve A. Kay, Masaru Ohme-Takagi, Motomu Endo, Takashi Araki, BRANCHED1 Interacts with FLOWERING LOCUS T to Repress the Floral Transition of the Axillary Meristems in Arabidopsis , The Plant Cell, Volume 25, Issue 4, April 2013, Pages 1228–1242, https://doi.org/10.1105/tpc.112.109090
- Share Icon Share
Abstract
Plant architecture shows a large degree of developmental plasticity. Some of the key determinants are the timing of the floral transition induced by a systemic flowering signal (florigen) and the branching pattern regulated by key factors such as BRANCHED1 (BRC1). Here, we report that BRC1 interacts with the florigen proteins FLOWERING LOCUS T (FT) and TWIN SISTER OF FT (TSF) but not with TERMINAL FLOWER1, a floral repressor. FT protein induced in leaves moves into the subtended bud, suggesting that FT protein also plays a role in promotion of the floral transition in the axillary meristem (AM). The brc1-2 mutant shows an earlier floral transition in the axillary shoots compared with the wild type, suggesting that BRC1 plays a role in delaying the floral transition of the AMs. Genetic and gene expression analyses suggest that BRC1 interferes with florigen (FT and TSF) function in the AMs. Consistent with this, BRC1 ectopically expressed in the shoot apical meristem delays the floral transition in the main shoot. These results taken together suggest that BRC1 protein interacts with FT and TSF proteins and modulates florigen activity in the axillary buds to prevent premature floral transition of the AMs.
INTRODUCTION
There is a large degree of developmental plasticity in plant architecture, which is determined in part by the timing of floral transition and the branching pattern. The floral transition is induced by a systemic flowering signal (florigen), and plant architecture is often remodeled upon the floral transition in the shoot apical meristem (SAM). In the rosette-forming plant Arabidopsis thaliana, floral transition is followed by rapid internode elongation and activation of axillary bud development in inductive long-day (LD) conditions. Since axillary bud development proceeds differently than that of the main shoot, the systemic flowering signal may also be perceived differently in the two locations.
Appropriate regulation of axillary bud development is achieved through integrating internal and environmental signals at each of the three developmental stages: axillary meristem (AM) initiation, bud differentiation, and shoot elongation. Among them, the regulation of AM initiation and shoot elongation has been studied intensively. Molecular genetic approaches have helped identify genes required for initiation of AMs. In Arabidopsis, REVOLUTA and related HD-ZIP genes are required to specify adaxiality of lateral organs and act to form AMs (Talbert et al., 1995; Emery et al., 2003). AM initiation requires the GRAS family gene LATERAL SUPPRESOR (Schumacher et al., 1999; Greb et al., 2003) and the Myb-like transcription factor (TF) REGULATOR OF AXILLARY MERISTEM1 (Keller et al., 2006; Müller et al., 2006). On the other hand, axillary shoot elongation is regulated by multiple plant hormones. Auxin produced in the main shoot suppresses axillary shoot elongation (Dun et al., 2006). Strigolactones, carotenoid-derived hormones produced in roots, also act as negative regulatory factors (Gomez-Roldan et al., 2008; Umehara et al., 2008; Crawford et al., 2010). Cytokinin is thought to be a secondary messenger and acts more locally in the buds to promote outgrowth.
Compared with initiation and elongation of the axillary bud, regulation of differentiation including floral transition is far less understood. One gene expressed in the axillary bud known to regulate differentiation is BRANCHED1/TEOSINTE BRANCHED1-LIKE1 (BRC1/TBL1; hereafter referred as BRC1). BRC1 encodes a member of the TEOSINTE BRANCHED1, CYCLOIDEA, and PCF (TCP) TF family and was identified as an ortholog of maize (Zea mays) Teosinte branched1 (Tb1) (Doebley et al., 1997; Aguilar-Martínez et al., 2007; Finlayson, 2007). It was suggested that Tb1-like genes act downstream of strigolactone signaling to suppress development of axillary shoots in Arabidopsis and pea (Pisum sativum) (Aguilar-Martínez et al., 2007; Finlayson, 2007; Brewer et al., 2009). Since axillary buds of the brc1 mutant show advanced development compared with the wild type, it was suggested that BRC1 acts as a negative regulator of differentiation in axillary buds (Aguilar-Martínez et al., 2007). The brc1 mutant occasionally develops ectopic AMs at cotyledonary axils, suggesting that BRC1 also has an inhibitory role in initiation of AMs (Aguilar-Martínez et al., 2007). It was also suggested that BRC1 suppresses the elongation of axillary shoots (Aguilar-Martínez et al., 2007; Finlayson, 2007). These pleiotropic effects reflect multiple roles of BRC1 in axillary bud development. The expression of BRC1 is regulated by environmental signals, such as planting density (Aguilar-Martínez et al., 2007; Finlayson et al., 2010). Based on these observations, BRC1 was proposed to be an integrator of axillary bud development (Aguilar-Martínez et al., 2007). A BRC1 paralog, BRC2, is thought to have a partially redundant but distinct role in axillary bud development (Aguilar-Martínez et al., 2007; Finlayson, 2007; Finlayson et al., 2010).
In Arabidopsis, initiation of axillary bud development is closely linked to floral transition in the SAM under LD conditions (Hempel and Feldman, 1994). The molecular mechanism controlling floral transition is well understood in Arabidopsis and other species. Among the key regulators of floral transition, FT acts as a floral pathway integrator. Both endogenous and environmental signaling pathways are integrated in the transcriptional regulation of FT, which encodes a 20-kD protein of the PEBP/RKIP (for phosphatidylethanolamine binding protein/Raf kinase inhibitor protein) family that acts as a long-distance flowering signal (Kardailsky et al., 1999; Kobayashi et al., 1999; Lifschitz et al., 2006; Corbesier et al., 2007; Jaeger and Wigge, 2007; Lin et al., 2007; Mathieu et al., 2007; Tamaki et al., 2007; Notaguchi et al., 2008). FT expression is induced in phloem companion cells in cotyledons and leaves under inductive LD conditions (Takada and Goto, 2003). FT protein then moves into the SAM, where it interacts with the bZIP TF FD, to induce the expression of a floral meristem identity gene, APETALA1 (AP1) (Abe et al., 2005; Wigge et al., 2005). A recent study in rice (Oryza sativa) showed that the interaction between Hd3a (an FT ortholog) and FD1 (an FD ortholog) is not direct but is mediated by 14-3-3 proteins (Taoka et al., 2011). Os-FD1 interacts with a 14-3-3 protein through a conventional 14-3-3 recognition motif R/K-X-X-S-X-P, whereas Os-Hd3a has a noncanonical binding site for 14-3-3 proteins (Yaffe et al., 1997; Pnueli et al., 2001; Taoka et al., 2011). Other PEBP/RKIP proteins are also involved in the regulation of the floral transition in Arabidopsis. TWIN SISTER OF FT (TSF), the paralog of FT, also acts as a floral promoter (Michaels et al., 2005; Yamaguchi et al., 2005). Although TSF has a minor role under LD conditions in which FT is robustly expressed, TSF makes a prominent contribution to the floral transition under short-day (SD) conditions (Yamaguchi et al., 2005). By contrast, TERMINAL FLOWER1 (TFL1) delays the floral transition through interaction with FD (Bradley et al., 1997; Hanano and Goto, 2011). TFL1 also plays a key role in maintenance of inflorescence meristems. In the tfl1 mutant under LD conditions, the main inflorescence is terminated with a fused floral structure and the axillary shoots are replaced by single flowers (Shannon and Meeks-Wagner, 1991; Alvarez et al., 1992).
In Arabidopsis, when floral transition is induced in the SAM, preexisting primordia develop into cauline leaves and newly formed primordia become floral buds. AMs are initiated and develop basipetally after floral transition of the SAM in LD conditions (Hempel and Feldman, 1994). It was suggested that AMs are at first insensitive to flowering signals since they form vegetative nodes before making flowers even though a sufficient amount of the systemic flowering signal is produced to induce floral transition in the SAM (Hempel and Feldman, 1994; Grbić and Bleecker, 1996). Therefore, florigen activity and/or perception are likely to be differently modulated in AMs than in the SAM.
In this study, we identified BRC1 as an interactor of FT without mediation by a 14-3-3 protein and demonstrated that BRC1 interacts with TSF but not TFL1. Our results indicate that BRC1 modulates the florigen activity in the AMs and confers a specific mode of regulation to the floral transition in AMs.
RESULTS
BRC1 Interacts with FT
The fact that FT acts with the bZIP protein FD in a transcriptional complex prompted us to seek other TFs as possible interacting proteins. To identify TFs that can interact with FT, we performed yeast two-hybrid screening using two different TF libraries. Robotized screening of the first library (Steve Kay Lab) revealed 64 candidates when the threshold was set to twofold higher than the average (see Supplemental Table 1 online). By manual screening of a second TF library (Mitsuda et al., 2010), 10 TFs were found to show reproducible interaction with FT (see Supplemental Figure 1 online). Interestingly, five candidates in the first screening and four in the second were TFs belonging to the TCP family. Because some TCP family members were not represented in the two TF libraries, we performed yeast two-hybrid assays with the individual TCP transcriptional factors that have been reported to be involved in various developmental processes. Among them, BRC1 reproducibly interacted with FT in yeast two-hybrid assays (Figure 1A). We focused on BRC1 as a good candidate to be a link between the floral transition and concomitant architectural remodeling through branch development.
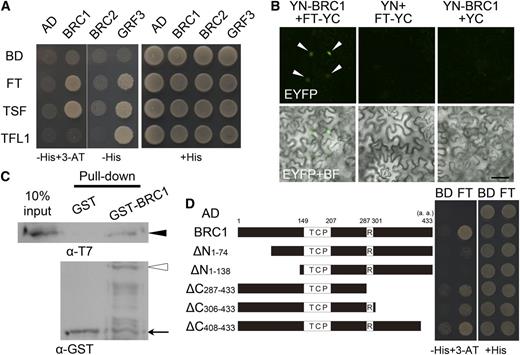
Protein Interaction between BRC1 and FT.
(A) Yeast two-hybrid analysis of protein interaction between BRC1, BRC2, or GRF3 and FT, TSF, or TFL1. Clones containing each combination of bait and prey vectors were grown on nonselective medium (+His) or on selective medium (−His). For the clones containing BRC1, 3-amino-1,2,4-triazole (3-AT) was added to the medium (left two rows). pCUY and pGAD424 empty vectors were used as a negative control (BD [binding domain] and AD [activation domain], respectively).
(B) BiFC analysis of the interaction between BRC1 and FT in N. benthamiana leaf epidermal cells. The N-terminal fragment of EYFP was fused to BRC1 (YN-BRC1) and the C-terminal fragment of EYFP to FT (FT-YC). The fusion proteins were transiently expressed under the cauliflower mosaic virus 35S promoter through agroinfiltration. EYFP fluorescence (top panels) and EYFP fluorescence merged with a bright-field (BF) image (bottom panels) are shown. Combinations in which one of the fusion constructs was replaced with a fragment of EYFP (YN or YC) were used as negative controls (central and right columns). Arrowheads indicate complemented EYFP fluorescence in the nucleus. Bar = 200 μm.
(C) In vitro pull-down assay demonstrating the interaction between BRC1 and FT. Closed arrowhead, open arrowhead, and arrow indicate T7-FT, GST-BRC1, and GST, respectively.
(D) Yeast two-hybrid assay of protein interaction between FT and truncated versions of BRC1. TCP, TCP domain; R, R domain; a.a., amino acids.
We further investigated interaction between BRC1 and two FT homologs, TSF (in the FT clade) and TFL1 (in the TFL1 clade), by yeast two-hybrid assay. TSF also showed interaction with BRC1, while TFL1 did not (Figure 1A). No interactions between the TCP protein BRC2, which is closely related to BRC1 (Aguilar-Martínez et al., 2007), and these PEBP/RKIP proteins were detected in our yeast two-hybrid experiments (Figure 1A).
Protein interaction between FT and BRC1 was further confirmed in plant cells by bimolecular fluorescence complementation (BiFC) assays in Nicotiana benthamiana and in vitro by pull-down assays. A clear BiFC signal was detected in the nucleus, indicating that FT interacts with BRC1 in plant cells and that the FT-BRC1 complex is localized to the nucleus (Figure 1B). BRC1 was able to pull down FT in the in vitro assay, indicating that their interaction is likely direct (Figure 1C).
Analysis using a series of deletion constructs showed that the C-terminal region of BRC1 including the conserved R domain is dispensable for interaction with FT (Figure 1D). However, removal of the entire C terminus after the TCP domain disrupted the interaction (see Supplemental Figure 2 online). In addition, a relatively small deletion of the N-terminal 74 amino acid residues abolished the interaction (Figure 1D). These results suggest the N-terminal region of BRC1 is necessary but not sufficient for interaction with FT.
14-3-3 Proteins Do Not Mediate the Interaction between BRC1 and FT
In rice, 14-3-3 proteins mediate the interaction between Hd3a (an FT ortholog) and FD1 (Taoka et al., 2011). Since Arabidopsis FT can interact with 14-3-3 proteins, it is likely that they mediate FT–FD interaction in Arabidopsis as well (Pnueli et al., 2001). This raises the question whether 14-3-3 proteins also mediate the interaction between BRC1 and FT. To explore whether 14-3-3 proteins mediate the interaction between BRC1 and FT, two sets of yeast two-hybrid assay were performed. First, we examined whether point mutations in FT that abolish interaction with 14-3-3 proteins also affect interaction with BRC1. All three mutant FT proteins tested still showed interaction with BRC1, suggesting that 14-3-3 proteins do not mediate the interaction (Figure 2A). BRC1 has a sequence conforming to a recognition motif of 14-3-3 proteins (R/K-X-X-S-X-P) in the C-terminal region (Yaffe et al., 1997; Taoka et al., 2011; Figure 2B). Mutation in this sequence (S390A) did not abolish interaction with FT, further supporting that 14-3-3 proteins are not required for the interaction (Figure 2A). Second, Ala scanning was performed to identify residues in FT required for interaction with BRC1. Among 46 mutant FT proteins tested, seven did not show interaction with BRC1 but could interact with a 14-3-3 protein (GENERAL REGULATORY FACTOR3 [GRF3; 14-3-3 psi]) (Figure 2C; see Supplemental Figure 3 online). When these residues were plotted on the FT three-dimensional structure model (Ahn et al., 2006; Protein Data Bank ID 1WKP), the region required for the interaction with BRC1 was distinct from the interaction interface between FT and 14-3-3 proteins (Taoka et al., 2011; Figure 2D). These results, taken together, indicate that the interaction between FT and BRC1 is not mediated by 14-3-3. Thus, BRC1 interacts with FT in a different manner than FD does.
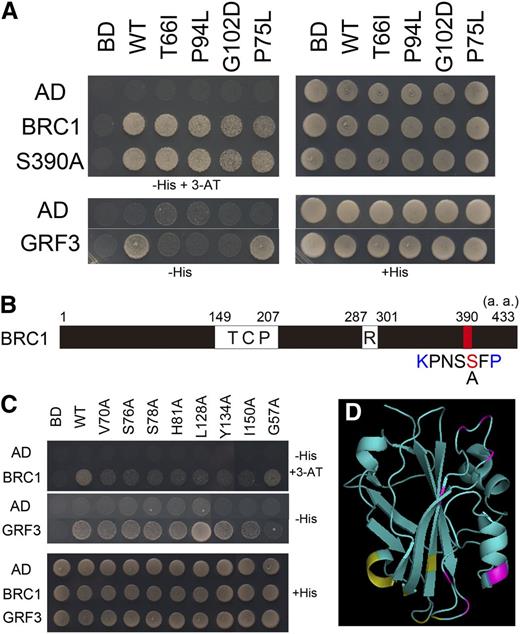
14-3-3 Proteins Do Not Mediate the Interaction between FT and BRC1.
(A) Yeast two-hybrid analysis of interaction between BRC1 and mutant FT proteins defective in interaction with 14-3-3 proteins. T66I, P94L, G102D, and P75L are mutant FT proteins, the former three of which do not interact with 14-3-3 proteins. S390A is a mutated BRC1 in which a Ser residue in a putative 14-3-3 recognition site (S390) was substituted by Ala. GRF3 was chosen as a representative 14-3-3 protein. Yeasts were grown on nonselective medium (+His) or on selective medium (−His and −His+3-AT). WT, the wild type.
(B) Schematic diagram of BRC1 protein depicting the putative 14-3-3 recognition site. TCP, TCP domain; R, R domain; a.a., amino acids.
(C) Yeast two-hybrid assay of interaction between mutant FT proteins and BRC1 or GRF3. Representative results for seven FT mutants defective in interaction with the BRC1 protein and a mutant defective in interaction with GRF3 were shown. These mutants were obtained by screening of Ala-scanned FT mutants (see Supplemental Figure 3 online). Yeasts were grown on nonselective medium (+His) or on selective medium (−His and −His+3-AT).
(D) Amino acid residues essential for interaction with BRC1 ([C], indicated by magenta) or 14-3-3 proteins ([A], indicated by yellow; Taoka et al., 2011) on a ribbon model of the FT protein.
Whether candidates from the yeast two-hybrid screen using the second TF library interact with FT through 14-3-3 proteins was also investigated. A bZIP TF, bZIP30, interacted with 14-3-3 proteins and failed to interact with the mutant FT that cannot interact with 14-3-3 proteins. This suggests that 14-3-3 is probably required to mediate the FT-bZIP30 interaction (see Supplemental Figure 1 online). Other candidates interacted with at least one of the mutant FT proteins that cannot interact with 14-3-3 proteins, indicating they probably do not require 14-3-3 proteins for interaction with FT (see Supplemental Figure 1 online).
FT Protein Can Move to an Axillary Bud from the Subtending Leaf
BRC1 is expressed in AMs and the brc1 mutant shows defective phenotypes specifically in the axillary buds (Aguilar-Martínez et al., 2007; Finlayson, 2007). On the other hand, FT is expressed in the leaf vasculature (Takada and Goto, 2003; Yamaguchi et al., 2005; Notaguchi et al., 2008), and FT protein moves into the SAM to promote floral transition (Corbesier et al., 2007; Jaeger and Wigge, 2007; Mathieu et al., 2007; Notaguchi et al., 2008). The observation that BRC1 and FT protein can interact in plant cells and in vitro strongly suggests that FT also has a role in the axillary buds.
To explore the possible expression of FT in the axillary buds, we examined the expression pattern of FT using gFT:GUS (for β-glucuronidase) plants (Notaguchi et al., 2008). Because of its large molecular mass (88.9 kD), FT-GUS fusion protein is a good indicator of expression pattern. It does not seem to move out of the cells where originally expressed (Notaguchi et al., 2008) and did not promote flowering when expressed in the leaf (see Supplemental Figure 4 online). As previously reported, GUS staining was observed in vascular bundles toward the periphery of the rosette leaf blade in gFT:GUS plants (Takada and Goto, 2003; Yamaguchi et al., 2005; Notaguchi et al., 2008; Figure 3A). As in the rosette leaves, FT expression was detected in the vasculature in the distal part of cauline leaves but not in the axillary bud (Figure 3B). Further observations of sections of axillary buds did not reveal staining in the buds at the cauline or rosette leaf axils (Figures 3C and 3D; see Supplemental Figure 4 online). We next examined whether FT protein expressed in a leaf blade moves into the axillary bud subtended by the leaf using the ProHSP18.2:FT-EGFP-inducible reporter system (Notaguchi et al., 2008). Induction of FT-EGFP (for enhanced green fluorescent protein) protein in a single leaf blade was confirmed after local transient heat treatment (see Supplemental Figure 5 online). One leaf blade was heated for 2 h to induce FT-EGFP fusion protein, and 24 h after the end of the heat treatment, FT-EGFP fluorescence in the axillary bud subtended by the heat-treated leaf was observed (Figure 3E). When the heat-treated leaf blade was removed by cutting the base of the leaf blade immediately after the heat treatment, fluorescence was not detected in the axillary bud (Figure 3F). These results showed that FT-EGFP fusion protein expressed in the leaf blade can move into the axillary bud. Based on expression in leaves and the ability to move into the axillary bud from the subtending leaf, we concluded that FT protein is likely to be present in axillary buds.
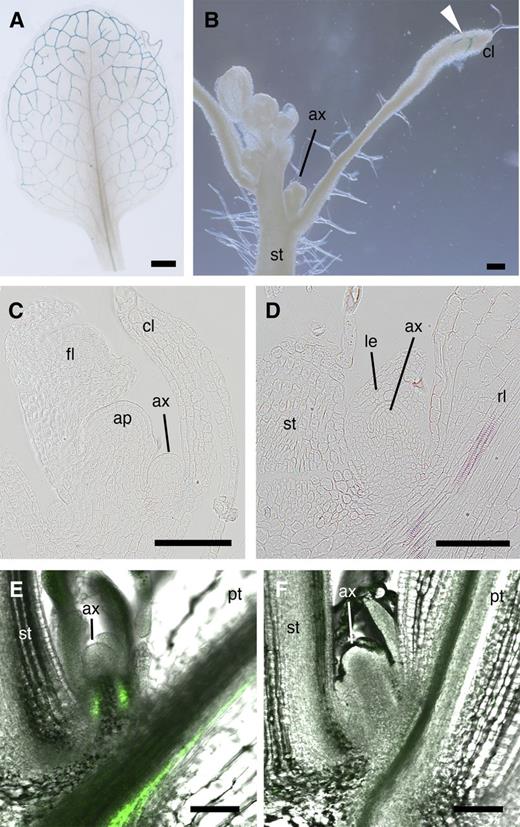
FT Expression Pattern and Movement of FT Protein into Axillary Buds.
(A) to (D) FT expression patterns monitored by GUS staining of gFT:GUS plant. Whole-mount preparations of a rosette leaf (A) and the main stem of a plant with the axillary bud subtended by a cauline leaf (B). Resin sections of the axillary buds subtended by a cauline leaf (C) and by a rosette leaf (D). Arrowhead in (B) indicates staining in the peripheral region of the cauline leaf.
(E) and (F) Movement of FT-EGFP into an axillary bud from the subtending leaf. EGFP fluorescence merged with a bright-field image of the axillary bud is shown. FT-EGFP expression was induced from the ProHSP18.2:FT-EGFP transgene by heat treatment of the leaf blade of a rosette leaf (see Supplemental Figure 5 online). EGFP fluorescence was observed 24 h after the end of the heat treatment. The treated leaf blade was either left intact (E) or cut off immediately after the heat treatment to prevent FT-EGFP export (F). The cut site is not seen in (F).
ap, apical meristem; ax, axillary meristem; cl, cauline leaf; fl, floral bud; pt, petiole of the heat-treated rosette leaf; rl, rosette leaf; st, stem. Bar in (A) = 1 mm and bars in other panels = 100 μm.
brc1-2 Mutants Show Accelerated Floral Transition in the AMs
In order to gain insight into the physiological role of the interaction between FT and BRC1, we looked for relevant phenotypes in the brc1-2 (tbl1-1) mutant (Alonso et al., 2003; Aguilar-Martínez et al., 2007; Finlayson, 2007). As previously reported, more axillary shoots grew out in the brc1-2 mutant than in the wild type (Aguilar-Martínez et al., 2007; Finlayson, 2007; Figure 4A). We also confirmed that the development of AMs is more advanced in brc1-2 than in the wild type (Aguilar-Martínez et al., 2007; Figure 4B). Further observation indicated that almost all the existing axillary buds proceeded to reproductive phase in brc1-2 mutant shortly after bolting of the main shoot. By contrast, lower axillary buds remained vegetative in the wild type (Figure 4B). Furthermore, the axillary shoots of the uppermost nodes formed no leaves in many brc1-2 plants, whereas two leaves were usually formed in wild-type plants (Figure 4A). Quantification of leaf number showed that brc1-2 has fewer leaves on the axillary shoots at every position compared with the wild type (Figure 4C). A similar phenotype was observed for the lowest cauline and uppermost rosette nodes in a previous report (Finlayson et al., 2010).
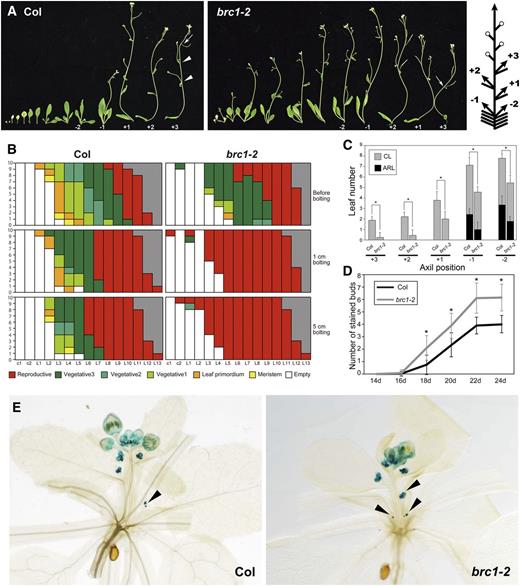
Early Floral Transition of the Axillary Shoots in brc1 Mutants.
(A) Axillary shoot phenotype of the wild type (Col) and brc1-2 mutant. Successive axillary shoots with the subtending leaf of 44-d-old plants are shown. Arrowheads and arrows indicate vegetative nodes and the first flowers in the most apical branches. Plus numbers represent cauline-leaf axils in the acropetal direction, and minus numbers represent rosette-leaf axils in the basipetal direction as shown in the right diagram. In the diagram, open circles, arrows, and bars represent flowers, shoots, and leaves, respectively.
(B) Developmental stages of the buds in the axils of cotyledons (c1 and c2) and rosette leaves (L1 to L15) of 10 plants of wild-type Col (left) and brc1-2 (right). Developmental stages were defined according to Aguilar-Martínez et al. (2007). Gray indicates absence of the leaf node such that the position is occupied by a flower. Observations were made on the days when the first floral buds became visible (before bolting), the main shoot became 1-cm long (1 cm bolting), and the main shoot became 5-cm long (5 cm bolting).
(C) Number of leaves formed on axillary shoots at the five axil positions of the wild type (Col) and brc1-2 mutant grown in LD. The axil position is defined in (A). Leaves in the basal part of axillary shoots without internodes are classified as axillary rosette leaves (ARL) and those with elongated internodes as cauline leaves (CL). Error bars indicate the sd (n = 9). The differences significant between Col and brc1-2 in two-tailed multiple t test with Bonferroni correction (P < 0.05) are indicated with asterisks.
(D) and (E) Expression of AP1 in the axillary buds monitored by ProAP1:GUS. Number of GUS-positive axillary buds in ProAP1:GUS plants in wild-type (Col) and brc1-2 background grown under LD (D). Error bars indicate the sd (n ≥ 21). The differences significant in two-tailed multiple t test with Bonferroni correction (P < 0.05) with respect to the wild type (Col) are indicated with asterisks. Representative 22-d-old ProAP1:GUS plants in wild-type (Col) and brc1-2 backgrounds (E). Arrowheads indicate GUS-positive axillary buds at rosette-leaf axils.
Because leaf number is generally considered to reflect the timing of the floral transition, we assumed that the reduction of leaf number in brc1-2 indicates earlier floral transition in the AMs. To confirm this possibility in the context of gene expression, we examined AP1 expression in the AMs using a ProAP1:GUS reporter (Hempel et al., 1997). In the SAM, the timing of the onset of AP1 expression did not differ between brc1-2 and wild-type backgrounds, in accordance with the fact that the leaf number of the main shoot did not significantly differ between the two genotypes. By contrast, the floral transition of the AMs proceeded differently between the brc1-2 mutant and wild-type plants. Eighteen days after germination, almost all the brc1-2 plants (92%; 22/24) had at least one AP1-expressing axillary bud, whereas only about a half of the wild-type plants (53%; 16/30) did. The quantification of AP1-expressing buds indicated that the brc1-2 mutant formed more reproductive axillary buds than did the wild type (Figures 4D and 4E). Introduction of a genomic fragment of BRC1 into brc1-2 complemented the leaf number phenotype (see Supplemental Figure 6 online). Therefore, we concluded that floral transition of the AM is accelerated in the brc1-2 mutant.
The ft-2 Mutation Is Epistatic to the brc1-2 Mutation
The observations that the number of leaves on the axillary shoots differed depending on the node positions in the wild type and that floral transition of AMs in the brc1-2 mutant was accelerated imply that floral transition of AMs is a regulated process (Figure 4). Furthermore, the observation that FT protein could move into the axillary buds suggests that FT could be involved in this regulation. To address this, we examined floral transition of AMs in ft-2 single and brc1-2 ft-2 double mutants. Compared with the wild type, the ft-2 mutant formed more leaves on its axillary shoots (Figure 5A), suggesting that floral transition in AMs was delayed in the ft-2 mutant. The brc1-2 ft-2 double mutant formed almost the same number of leaves as the ft-2 single mutant on axillary shoots of cauline leaves (Figure 5A). Thus, the acceleration of AM floral transition observed in the brc1-2 mutant requires functional FT in cauline axils. In axillary shoots of rosette leaves, the brc1-2 ft-2 mutant formed fewer leaves than the ft-2 mutant (Figure 5A). The elongation of axillary shoots at the rosette axils was also enhanced in brc1-2 ft-2 compared with ft-2 (Figure 5B).
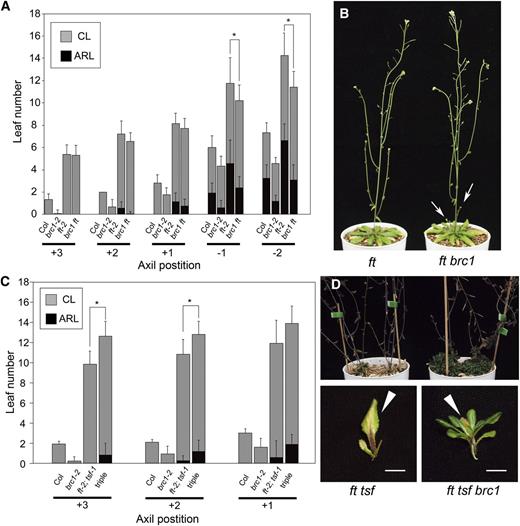
Delayed Floral Transition of Axillary Shoots in ft and ft tsf Mutants and Genetic Interaction with brc1.
(A) Number of leaves formed on the axillary shoots at the five axil positions of the wild type (Col) and brc1-2, ft-2, and brc1-2 ft-2 mutants grown in LD. Error bars indicate the sd (n = 12 for Col and brc1-2; n = 24 for ft-2 and brc1-2 ft-2). The differences significant between ft-2 and brc1-2 ft-2 in two-tailed multiple t test with Bonferroni correction (P < 0.05) are indicated with asterisks. ARL, axillary rosette leaves; CL, cauline leaves.
(B) Representative plants of ft-2 and brc1-2 ft-2 mutants grown in LD for 60 d Arrows indicate elongated axillary shoots at rosette axils.
(C) Number of leaves formed on the axillary shoots at the three axil positions of the wild type (Col) and brc1-2, ft-2 tsf-1, and brc1-2 ft-2 tsf-1 (triple) mutants grown in LD. Error bars indicate the sd (n = 12). The differences significant between ft-2 tsf-1 and brc1-2 ft-2 tsf-1 in two-tailed multiple t test with Bonferroni correction (P < 0.05) are indicated with asterisks.
(D) Axillary shoot phenotype of ft-2 tsf-1 and brc1-2 ft-2 tsf-1 mutants. Top panel shows the rosette part of the plants, and bottom panels show the uppermost rosette leaf with the axillary bud or shoot. Note that most of the primary rosette leaves on the main axis were decaying or senescent. Arrowheads in the bottom panels indicate the senescent rosette leaves. Bars = 5 mm.
Designation of the axil positions and classification of leaves in (A) and (C) are as in Figure 4.
[See online article for color version of this figure.]
In the ft mutant background, TSF promotes the floral transition during prolonged vegetative phase (Yamaguchi et al., 2005; Hiraoka et al., 2013). Because our yeast two-hybrid assay showed that TSF is also able to interact with BRC1 (Figure 1A), TSF is likely to be responsible for the acceleration of floral transition in the AMs at rosette axils in the brc1-2 ft-2 mutant. To test this hypothesis, the phenotype of the brc1-2 ft-2 tsf-1 triple mutant was examined. As observed in the brc1-2 ft-2 and ft-2 mutants, brc1-2 ft-2 tsf-1 formed almost the same number of leaves as the ft-2 tsf-1 double mutant in axillary shoots of cauline leaves (Figure 5C). The ft-2 tsf-1 double mutant did not form elongated axillary shoots on its rosette axils under our experimental conditions (Hiraoka et al., 2013; Figure 5D). In the brc1-2 ft-2 tsf-1 triple mutant, rosette leaves did form axillary buds and developed many leaves, but over 100 d after bolting of the main shoot, the buds failed to make flowers and did not elongate (Figure 5D). These results suggest that prompt floral transition and subsequent elongation of the axillary shoots in the brc1-2 mutant require either FT or TSF functions.
In contrast with the ft-2 single and ft-2 tsf-1 double mutants, both of which were epistatic to the brc1-2 mutant, the tfl1-17 mutant showed an additive effect on reduction in leaf number of axillary shoots in the double mutant with brc1-2 (see Supplemental Figure 7 online). Taking this together with the results of yeast two-hybrid assay (Figure 1A), BRC1 and TFL1 are likely to act independently to suppress floral transition in AMs.
Relationship between BRC1 and Flowering-Time Genes
FT acts together with FD to promote floral transition in the SAM. Because FD is also expressed in the AMs (Abe et al., 2005; Wigge et al., 2005; Figure 6A), it is likely that FD also has a role in floral transition of the AMs. Similar to the brc1-2 ft-2 mutant, the brc1-2 fd-1 mutant showed substantial suppression of the brc1-2 phenotype in terms of the leaf number of axillary shoots (Figure 6B). This indicates that FD also acts as a promoter of the floral transition in AMs.
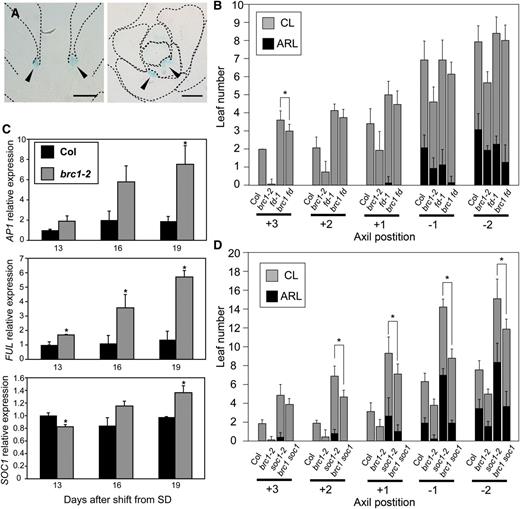
brc1 and Genes Acting Downstream of Florigen.
(A) Expression of FD in the AMs monitored by ProFD:GUS. Longitudinal (left) and transverse (right) sections of ProFD:GUS plants grown for 18 d under LD conditions are shown. Arrowheads indicate AMs at rosette axils. Dotted lines show the outline of the tissues.
(B) Number of leaves formed on the axillary shoots at the five axil positions of the wild type (Col) and brc1-2, fd-1, and brc1-2 fd-1 mutants. Error bars indicate the sd (n = 15). The difference significant between fd-1 and brc1-2 fd-1 in two-tailed multiple t test with Bonferroni correction (P < 0.05) is indicated with an asterisk. ARL, axillary rosette leaves; CL, cauline leaves.
(C) Relative expression levels of FT-downstream genes AP1, FUL, and SOC1 after the shift from SD to LD. Plants were grown for 24 SDs and transferred to LD. Rosette-axil samples were analyzed (see Methods). Error bars indicate the se for three biological replicates. Differences significant in two-tailed t test (P < 0.05) with respect to the wild type (Col) are indicated with asterisks.
(D) Number of leaves formed on axillary shoots at the five axil positions of the wild type (Col) and brc1-2, soc1-2, and brc1-2 soc1-2 mutants. Error bars indicate the sd (n = 9). The differences significant between soc1-2 and brc1-2 soc1-2 in two-tailed multiple t test with Bonferroni correction (P < 0.05) are indicated with asterisks.
Designation of the axil positions and classification of leaves in (B) and (D) are as in Figure 4.
It was proposed that AP1, FRUITFULL (FUL), and SUPRESSOR OF OVEREXPRESSION OF CO1 (SOC1) are downstream genes of the FT-FD floral transition pathway (Abe et al., 2005; Michaels et al., 2005; Teper-Bamnolker and Samach, 2005; Wigge et al., 2005; Yoo et al., 2005; Searle et al., 2006). To examine the effect of BRC1 on the expression of these genes, wild-type and brc1-2 mutant plants were grown under noninductive SD conditions and then transferred to LD. After bolting of the main shoot, a plant section containing the axils of the rosette leaves was harvested by removing the main shoot, expanded leaves, hypocotyl, and root at three time points (13, 16, and 19 d after the LD shift) and subjected to expression analysis. Quantitative RT-PCR showed that AP1 and FUL were already upregulated 13 d after the LD shift in brc1-2 compared with the wild type (Figure 6C). These two genes were further upregulated in brc1-2 during further growth in LD but remained at a low level in the wild type. Although SOC1 expression was not upregulated in brc1-2 compared with the wild type at 13 d after the LD shift, a gradual increase was observed during the subsequent growth in LD (Figure 6C). The floral meristem identity gene LEAFY (LFY) is expressed in an FT-independent manner. Although LFY expression level was elevated in brc1-2 13 d after the LD shift, unlike AP1 and FUL, subsequent upregulation did not occur at later time points (see Supplemental Figure 8 online). These results indicate that BRC1 represses photoperiod-dependent expression of FT downstream genes in axillary buds.
SOC1 functions in floral transition of AMs together with FUL and other SOC1-related MADS box genes (Melzer et al., 2008; Dorca-Fornell et al., 2011). In accordance with this, the soc1-2 mutant formed many more leaves on axillary shoots compared with the wild type (Figure 6D). Nevertheless, the soc1-2 mutation only partially suppressed the phenotype of the brc1-2 mutant (Figure 6D). These results suggest that, unlike the cases of FT and TSF, the brc1-2 phenotype is not fully dependent on SOC1 function.
BRC1 Can Delay Floral Transition When Expressed in the SAM
The observations described so far indicate that BRC1 has a role in preventing AMs from undergoing early floral transition. The abilities of FT to be transported into the axillary buds and to interact with BRC1 suggest that BRC1 is likely to act through inhibition of FT function. This raises the possibility that BRC1 could delay the floral transition of the main shoot when expressed ectopically in the SAM. To test this possibility, the flowering-time phenotype of transgenic plants expressing BRC1 in the SAM under the control of the FD promoter (ProFD:BRC1) was examined. As expected, ProFD:BRC1 plants showed significant delay in floral transition of the SAM under inductive LD (Figure 7; see Supplemental Figure 9 online). Previously, it was shown that overexpression of BRC1 under the control of cauliflower mosaic virus 35S promoter resulted in pleiotropic developmental defects (Aguilar-Martínez et al., 2007). In this study, ProFD:BRC1 plants showed mild retarded growth in early developmental stages but no obvious morphological alterations (Figure 7A). In SD, the effect of ProFD:BRC1 was more subtle (see Supplemental Figure 10 online).
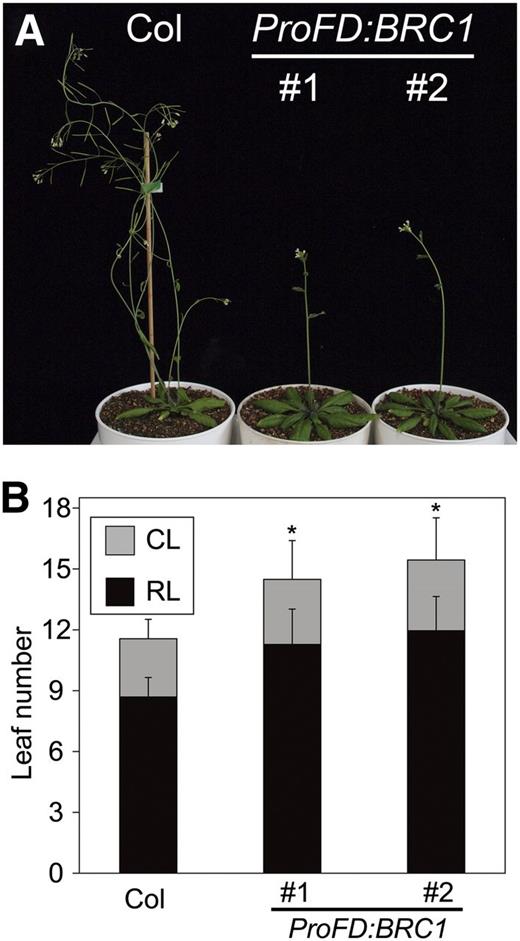
Ectopic Expression of BRC1 Delays Floral Transition in the SAM.
(A) Thirty-day-old plants of wild type (Col) and two independent lines of ProFD:BRC1 (#1 and #2) grown in LD.
(B) Flowering time measured by the number of rosette leaves (RL) and cauline leaves (CL). Error bars indicate the sd (n = 15). The differences significant in two-tailed Dunnett's test (P < 0.01) in the total leaf numbers with respect to Col are indicated with asterisks.
[See online article for color version of this figure.]
DISCUSSION
In this study, we showed that the BRC1 interacts with FT. BRC1 interacts also with TSF, whereas it did not interact with TFL1. This establishes BRC1 as an interactor specific to the FT clade of PEBP/RKIP proteins. Our study indicates that FT protein expressed in the leaf is transported into the axillary bud and promotes floral transition of the AM. BRC1 expressed in the axillary bud interacts with FT to inhibit its function. Thus, BRC1, previously proposed to be an integrator of branching signals within the axillary buds, plays a role in modulating the activity of a long-distance florigen signal to delay floral transition in AMs.
FT Is Able to Interact with Various TFs
Yeast two-hybrid screening using TF libraries revealed numerous candidate FT-interacting proteins. Although the functional significances of these interactions are beyond the scope of this study, it is to be noted that some of the candidates obtained here overlapped with recently reported candidates for interactors of FT and related proteins. Yeast two-hybrid screening using a cDNA library from inflorescence meristems, floral meristems, and floral buds identified bZIP52, TCP8, TCP15, and TCP22 as candidates to interact with FT in Arabidopsis (Liu et al., 2012). Yeast two-hybrid screening with Md-FT1, a homolog of FT in apple (Malus × domestica) showed that Md-VOZ1, Md-TCP2, and Md-TCP4 (belonging to the same clade as At-VOZ1, At-TCP2 and 24, and At-TCP3 and 4, respectively) interact with Md-FT1 (Mimida et al., 2011). Given the repeated identification in separate screenings, these TCP, bZIP, and VOZ proteins are promising candidates for factors acting with FT protein.
BRC1 Interacts with FT and TSF Proteins without Mediation by 14-3-3 Proteins
We identified BRC1 as specifically interacting with FT and TSF, but not with TFL1. By contrast, many of the previously reported FT interactors showed interaction with TFL1 as well. For example, FD and FD PARALOG interact with both FT/TSF and TFL1 (Abe et al., 2005; Wigge et al., 2005). Tomato (Solanum lycopersicum) SELF-PRUNING–interacting proteins (SPAK, SIP4, and SPGB) and maize FD homolog DELAYED FLOWERING also interact with FT clade and TFL1 clade proteins (Pnueli et al., 2001; Danilevskaya et al., 2008, 2010). For some FT interactors, such as FT-INTERACTING PROTEIN1 and other factors from the screening mentioned above, it remains to be examined whether they interact with TFL1 clade proteins (Mimida et al., 2011; Liu et al., 2012). FT clade and TFL1 clade proteins seem to share common interactors through the mediation of 14-3-3 proteins. FT and TFL1 share the ability to interact with a common subset of 14-3-3 isoforms (e.g., GRF3) (Figure 1A). In rice, 14-3-3 proteins mediate the formation of the Hd3a-FD1 (corresponding to FT-FD in Arabidopsis) complex (Taoka et al., 2011). In tomato, the SELF-PRUNING–interacting factors mentioned above also interact with 14-3-3 proteins (Pnueli et al., 2001). By contrast, BRC1 does not seem to require 14-3-3 proteins for interaction with FT.
It is likely that FT interacts with a variety of proteins using 14-3-3 proteins as a hub. Here, bZIP30 was found to be a putative 14-3-3–dependent FT interactor, whereas other candidates seemed to be independent of 14-3-3 proteins (see Supplemental Figure 1 online). These observations indicate that interaction mediated by 14-3-3 is not the only way in which FT interacts with TFs, raising the possibility that the protein complex containing FT has more components than known at the moment. Another possibility is that FT uses different sets of partners in different cells or for different functions.
BRC1 Is a Negative Regulator of Floral Transition in AMs
FT acts as a systemic flowering signal (florigen) and moves from the leaves to the SAM (Corbesier et al., 2007; Jaeger and Wigge, 2007; Lin et al., 2007; Mathieu et al., 2007; Tamaki et al., 2007; Notaguchi et al., 2008). FT protein expressed under the HSP18.2 promoter in a single leaf also moved into the subtending axillary bud (Figure 3). In addition, ft-2 mutants showed delayed floral transition in AMs (Figure 5). Taken together, these findings demonstrate that FT is a key regulator of floral transition in AMs as well as in the SAM. Recent work showed that FT is involved in the regulation of axillary shoot elongation (Hiraoka et al., 2013), indicating that FT has an additional role in axillary buds after the transition.
On the other hand, BRC1 was previously proposed to be an integrating repressor of axillary bud development (Aguilar-Martínez et al., 2007). BRC1 suppresses the initiation, differentiation, and elongation of axillary buds (Aguilar-Martínez et al., 2007; Finlayson, 2007). In this study, the brc1-2 mutant showed a reduction in the number of leaves on axillary shoots, as was, in part, reported previously (Finlayson et al., 2010), and earlier onset of AP1 expression in the axillary buds (Figure 4). Furthermore, when ectopically expressed in the SAM, BRC1 delayed floral transition of the main shoot, with an increase in the number of leaves formed on the main axis (Figure 7). These observations, taken together, strongly suggest that BRC1 acts as a negative regulator of the floral transition in AMs.
Since BRC1 protein interacts with FT and TSF proteins, and brc1 and ft (tsf) mutants showed opposite phenotypes in the AMs, BRC1 and FT play antagonistic roles likely through their protein–protein interaction. Genetic analyses indicated that the ft-2 and tsf-1 mutations are epistatic to brc1-2, suggesting that BRC1 inhibits FT and TSF functions (Figure 5). This is supported by the SD-to-LD shift transcript profiling experiment, where genes downstream of FT were overinduced in the brc1-2 mutant (Figure 6).
TFL1 also antagonizes FT function, but through competitive interaction with the common partner, FD (Bradley et al., 1997; Abe et al., 2005; Wigge et al., 2005; Hanano and Goto, 2011). Under LD conditions, when florigen activity is high, the main (primary) inflorescence of the tfl1 mutant makes only a few flowers and terminates soon after bolting (Shannon and Meeks-Wagner, 1991; Alvarez et al., 1992). In addition, TFL1 is expressed in the AMs as well as in the SAM, and some axillary shoots are replaced by solitary flowers in tfl1 mutants (Shannon and Meeks-Wagner, 1991; Alvarez et al., 1992; Bradley et al., 1997), indicating TFL1 is also involved in the suppression of phase transition in AMs. However, BRC1 showed no interaction with TFL1, and the tfl1-17 brc1-2 double mutant showed an additive reduction in the leaf number of axillary shoots under SD conditions (see Supplemental Figure 7 online). These results suggest that TFL1 and BRC1 act independently.
Although the mechanism by which BRC1 modulates the activity of FT/TSF remains to be clarified, the observation that FT interacts with BRC1 through a different region than the 14-3-3 protein interaction surface (Figure 2) supports the possibility that BRC1 binds to the FT/14-3-3/FD complex. In the deduced structure of the FT/14-3-3/FD complex based on Taoka et al. (2011), the positioning of the region of FT responsible for interaction with BRC1 would allow binding of BRC1 to the complex (see Supplemental Figure 11 online). If this is the case, BRC1 could inhibit the transcriptional activator function of the FT/14-3-3/FD complex. However, the possibility that BRC1 traps FT and prevents it from interacting with 14-3-3 proteins cannot be excluded.
Possible Conservation of the Antagonistic Mechanism between BRC1 and FT
The BRC1-like and FT-like genes seem to be conserved among species. BRC1 was first identified as a maize Tb1 ortholog in Arabidopsis (Aguilar-Martínez et al., 2007; Finlayson, 2007). Similar to the phenotype of Arabidopsis brc1 mutants, maize tb1 mutants show increased numbers of elongated axillary shoots (Doebley et al., 1995). Based on comparative genomics and expression analysis, it was suggested that Zea mays CENTRORADIALIS8 (ZCN8) is a maize counterpart of FT (Danilevskaya et al., 2008; Meng et al., 2011; Lazakis et al., 2011). The FD orthologous gene Zm-DLF1 was also identified. Mutation in Zm-DLF1 resulted in a late-flowering phenotype (Muszynski et al., 2006). Zm-ZCN8 interacts with Zm-DLF1, indicating that the FT-FD flowering activator complex is also conserved in maize. TB1/FINE CULM1 from rice is orthologous to Zm-Tb1, and the fc1 mutant shows enhanced tillering (Takeda et al., 2003). Os-Hd3a (an FT ortholog) and Os-FD1 interact with each other and promote floral transition in rice (Taoka et al., 2011). The tomato BRC1b and SINGLE FLOWER TRUSS genes, BRC1 and FT orthologs, respectively, were also characterized (Lifschitz et al., 2006; Martín-Trillo et al., 2011). These studies suggest that there is functional conservation of the FT-like and BRC1-like genes as florigens and integrators of the axillary bud development, respectively. Our study proposes that these two pathways interact in the AMs of Arabidopsis. It is worth investigating whether BRC1-like genes have conserved function in regulation of floral transition in AMs and whether FT-like and BRC1-like proteins interact with each other in these species.
The Significance of Regulating AM Floral Transition with Florigen and BRC1
In wild-type Arabidopsis, not all axillary buds elongate, and in ProAP1:GUS plants, buds formed at lower axils remain vegetative for longer after bolting of the main shoot (Figure 4). This may contribute to preventing existent buds from elongating simultaneously and preparing for future alterations in environmental and autonomous conditions.
AM-specific modulation of florigen activity by BRC1 might be more important under adverse growth conditions, when flowering is accelerated. For example, an increased number of neighboring plants leads to a change in light quality to a low ratio of red to far-red radiation (reviewed in Smith, 1982). When plants grow under far-red-rich light, FT, as an integrator gene of the flowering pathway, is upregulated and induces premature floral transition (Devlin et al., 2003; Endo et al., 2005; Sessa et al., 2005; Wollenberg et al., 2008). On the other hand, expression of BRC1 is also increased by a high planting density and in phyB mutants (Aguilar-Martínez et al., 2007; Finlayson et al., 2010). Although overproduced FT protein potentially activates the developmental program in undesirable parts of the plant, such as AMs, increased levels of BRC1 will suppress florigen activity specifically in AMs and thus enable the plant to concentrate its limited resources on the main shoot and promote reproductive success. Regulation of FT expression by environmental and internal signals is well studied (reviewed in Simpson and Dean, 2002; Boss et al., 2004; Fornara et al., 2010), and expression of BRC1 is also affected by external and hormonal cues (Aguilar-Martínez et al., 2007; Finlayson, 2007). These signals would converge to create a balance between FT and BRC1 and regulate floral transition in AMs. This additional mode of regulation in AMs enables modulation of the timing of axillary bud differentiation and thus determines plant architecture depending on external and internal conditions.
METHODS
Plant Materials and Growth Conditions
Arabidopsis thaliana Columbia-0 (Col) was used as the wild type. The ProAP1:GUS reporter (POP40) was kindly provided by M.F. Yanofsky (University of California at San Diego). The T-DNA insertion mutant brc1-2 (tbl1-1) was obtained from the ABRC and corresponds to the line SALK_091920 previously characterized (Aguilar-Martínez et al., 2007; Finlayson, 2007). Plant materials below were previously described: gFT:GUS (Notaguchi et al., 2008), ProFD:GUS (Abe et al., 2005), ft-2 (Imura et al., 2012), fd-1 (Abe et al., 2005), and soc1-2 (Lee et al., 2000). ProHSP18.2:FT-EGFP was constructed by fusing a promoter fragment (−853 to −1, relative to ATG) of the Arabidopsis HSP18.2 gene (Takahashi and Komeda, 1989) with the FT-EGFP open reading frame (ORF). ProFD:BRC1 was constructed based on the previously described ProFD:GUS construct (Abe et al., 2005) by replacing the GUS ORF with the BRC1 ORF. Seeds were stratified by keeping them at 4°C for 2 to 4 d and then transferred to 22°C LD (16 h light/8 h dark) with white fluorescent light (∼80 μmol m−2 s−1) or SD (8 h light/16 h dark) with white fluorescent light (∼100 μmol m−2 s−1) conditions. For analyses of the axillary shoot phenotypes and FT protein movement, plants were grown on vermiculite. Developmental stages of the axillary buds were determined according to Aguilar-Martínez et al. (2007). Observations were made on the days when the individual plant first showed a visible floral bud (before bolting), reached 1 cm in height (1 cm bolting), and reached 5 cm in height (5 cm bolting). The leaf number of the axillary shoot was counted after internode elongation. Leaves at the base of the axillary shoot without a visible elongated stem were counted as axillary rosette leaves and leaves on the elongated shoots as cauline leaves. For expression analyses, plants were grown on half-strength Murashige and Skoog medium supplemented with 0.5% Suc and 0.8% agar.
Plasmid Constructs
Plasmid constructs used in this study and primers used for cloning are described in Supplemental Tables 2 and 3 online.
Yeast Two-Hybrid Assay
For the first yeast two-hybrid screening, a TF library containing 1729 TFs was used (Pruneda-Paz et al., 2009). The ORF of the full-length FT protein was cloned in pENTR/D-TOPO (Life Technologies) and then transferred to pDEST32 (Life Technologies) using LR recombination reaction. The lacZ activity was measured by β-galactosidase assay. For the second screening and two-hybrid assays, the FT ORF was cloned in pCUY vector (Suzuki et al., 2001). A TF library containing 1503 TFs constructed in pDEST_GAD424 was described previously (Mitsuda et al., 2010). Plasmids isolated from positive colonies were transformed into Escherichia coli (DH5α) for sequencing. The interaction was confirmed after retransformation of yeast. pCUY and pGAD424 (Clontech/TAKARA) were used for construction of the other yeast two-hybrid constructs. The appropriate plasmids were transformed into a yeast strain AH109 (Clontech/Takara) using the lithium acetate method and selected on synthetic dropout medium plates lacking Leu and Trp. After 3 or 4 d of incubation at 30°C, yeast cells were transferred to liquid synthetic dropout medium lacking Leu and Trp and incubated for 24 h. After measuring OD600, 1 mL of each culture was centrifuged and resuspended in TE buffer, pH 7.4, to a final OD600 of 0.33. Five microliters of suspended yeast was spotted on synthetic dropout medium lacking Leu, Trp, and His for the His requirement test (−His). 3-Amino-1,2,4-triazole (0.5 mM) was added to the medium for prevention of nonspecific growth, where specified (−His+3-AT). As positive controls, yeast cells were also spotted on synthetic dropout medium lacking Leu and Trp (+His). These plates were incubated at 30°C and scored for growth of the yeast cells. To visualize the location of the residues important for protein interaction, an FT ribbon model was produced using Pymol (http://www.pymol.org) from the Protein Data Bank file (Ahn et al., 2006; Protein Data Bank ID 1WKP).
BiFC Assay in N. benthamiana Leaf Epidermal Cells
A split EYFP fragment encoding the N-terminal fragment of EYFP (YN; 1 to 155 amino acids) was cloned into pBI121 vector and then BRC1 ORF was inserted in frame after the YN to make YN-BRC1. Other constructs (FT-YC, YN, and YC in pBI121) were described previously (Abe et al., 2005). Nicotiana benthamiana plants were grown under continuous light conditions at 23°C. Agrobacterium tumefaciens strain pMP90 containing an appropriate construct was grown at 28°C in L-broth to the stationary phase. Bacterial cells were harvested by centrifugation and resuspended in 10 mM MgCl2 and 10 μg/mL acetosyringone (Wako) and left for 3 h at room temperature. The bacterial suspensions were infiltrated into the abaxial air spaces of leaves of 2- to 4-week-old plants using 1-mL syringes. Plants were kept for 36 h after the infiltration in continuous light conditions at 23°C. Complemented EYFP fluorescence was excited at 515 nm by an argon laser and detected in a range from 530 to 630 nm with confocal laser scanning microscopy (FV1000; Olympus).
In Vitro Pull-Down Assay
The BRC1 full-length ORF was cloned into pET41a (Novagen), and FT full-length ORF was cloned into pET24d (Novagen). The plasmids were transformed into E. coli BL21 (DE3) to express glutathione S-transferase (GST)-BRC1 and T7-FT, respectively. pET41a empty vector was used to express GST. For GST pull-down assays, GST-BRC1 or GST bacterial lysate was incubated with Glutathione Sepharose 4B beads (GE Healthcare), then 20 μL of the slurry (50% [w/v]) was mixed with 10 μL of T7-FT bacterial lysate and 220 μL of NETN buffer (50 mM Tris-HCl, pH 7.5, 100 mM NaCl, 1 mM EDTA, and 0.5% Nonidet P-40) and was gently stirred at 4°C for 2 h. The beads were washed thoroughly with the NETN buffer five times and subjected to SDS-PAGE and immunoblot analyses. T7-FT protein was detected with anti-T7 antibody (Novagen) and visualized using ECL plus (GE Healthcare) with Image Quant LAS 4000mini (GE Healthcare).
GUS Staining
Plants were fixed for 15 min in 90% acetone on ice and washed in PBS buffer twice. Fixed samples were incubated at 37°C for ∼16 h with staining solution (0.5 mg/mL X-Gluc, 50 mM sodium phosphate buffer, pH 7.0, 0.5 mM potassium ferrocyanide, 0.5 mM potassium ferricyanide, and 0.2% TritonX-100). For resin sectioning, samples were dehydrated through an ethanol series and embedded in Technovit 7100 (Heraeus Kulzer) and sectioned at a thickness of 5 μm with a microtome (RM2155; Leica). The ProAP1:GUS plant was observed with microscopy, and the number of stained buds was counted.
Single-Leaf Heat Treatment and Fluorescence Microscopy
ProHSP18.2:FT-EGFP plants grown under LD conditions for 22 d were subjected to single-leaf heat treatment essentially as described previously (Notaguchi et al., 2008). The leaf blade of the sixth leaf was exposed to 37°C for 2 h by placing it in the water-filled space between a heated copper plate and a slide glass to ensure good contact. Twenty-four hours after heat treatment, plants were embedded in 4% agar gel with PBS and sectioned at a thickness of 80 μm with a vibratome (VIB-1500; Intracel). GFP fluorescence was excited at 488 nm by an argon laser and detected in a range from 500 to 600 nm with confocal laser scanning microscopy (FV1000; Olympus).
Quantitative RT-PCR Analysis
Plants grown under SD for 24 d were transferred to LD and harvested after 13, 16, and 19 d. Rosette-axil samples were obtained by removing the main shoot, leaves, hypocotyl, and root. Total RNA was extracted using TRIzol reagent (Life Technologies) and treated with DNase I Amplification Grade (Life Technologies) according to the manufacturer's instructions. Total RNA (0.5 μg) was reverse transcribed in a 20-μL reaction mixture using Transcriptor (Roche Applied Science). The resultant cDNA solution was diluted with 30 volumes of water, and 3-μL aliquots were analyzed. Quantitative RT-PCR analyses were performed with the CFX96 system (Bio-Rad Laboratories) using SYBR green I. The primers used are described in Supplemental Table 4 online. Quantitative RT-PCR results were normalized to the geometric means of ASCORBATE PEROXIDASE3 (APX3; Hazen et al., 2005), REGULATORY PARTICLE TRIPLE-A 1A (RPT1a; Michael et al., 2008), and THIOREDOXIN H-TYPE3 (TRX3; Michael et al., 2008) and shown as the average of nine different reactions (biological × technical triplicate). Internal control genes used in this study were selected from nine genes according to Vandesompele et al. (2002).
Accession Numbers
Arabidopsis Genome Initiative locus identifiers for genes mentioned in this article are as follows: At3g18550 (BRC1), At1g65480 (FT), At1g68800 (BRC2), At4g20370 (TSF), At5g03840 (TFL1), At5g38480 (GRF3), At2g21230 (bZIP30), At4g35900 (FD), At1g69120 (AP1), At5g60910 (FUL), At2g45660 (SOC1), At5g61850 (LFY), At4g35000 (APX3), At1g53750 (RPT1a), At5g42980 (TRX3), At5g59720 (HSP18.2), At1g06850 (bZIP52), At4g18390 (TCP2), At1g53230 (TCP3), At3g15030 (TCP4), At1g58100 (TCP8), At1g69690 (TCP15), At1g72010 (TCP22), and At4g18390 (TCP24).
Supplemental Data
The following materials are available in the online version of this article.
Supplemental Figure 1. Yeast Two-Hybrid Assay between FT and Putative FT-Interacting Proteins.
Supplemental Figure 2. Yeast Two-Hybrid Assay between FT and BRC1 Fragments.
Supplemental Figure 3. Yeast Two-Hybrid Assay between Ala-Scanned Mutant FT Proteins and BRC1 or GRF3.
Supplemental Figure 4. Expression Analysis Using FT-GUS Fusion Protein.
Supplemental Figure 5. Heat Treatment of Leaf Blades of ProHSP18.2:FT-EGFP Plants.
Supplemental Figure 6. Complementation Test of the brc1-2 Mutant Using a Genomic Fragment of BRC1.
Supplemental Figure 7. BRC1 and TFL1 Likely Act Independently in the Regulation of Floral Transition of Axillary Shoots.
Supplemental Figure 8. Relative Expression of LFY after a Shift from SD to LD.
Supplemental Figure 9. Relative Expression of BRC1 in ProFD:BRC1 Plants.
Supplemental Figure 10. Flowering-Time Phenotype of ProFD:BRC1 Grown under SD.
Supplemental Figure 11. Amino Acid Residues Essential for the Interaction with BRC1 Shown on a Ribbon Model of FT in the Complex with 14-3-3 Protein and FD.
Supplemental Table 1. List of Candidate FT-Interacting Proteins Obtained from Yeast Two-Hybrid Screening of the Kay Lab Transcription Factor Library.
Supplemental Table 2. Constructs.
Supplemental Table 3. Primers Used in Plasmid Construction.
Supplemental Table 4. Primers Used in qRT-PCR.
Acknowledgments
We thank M.F. Yanofsky (University of California at San Diego) for POP40 seeds and the ABRC for brc1-2 seeds. We also thank Ayako Yamaguchi (Kyoto University) for discussion and Yuki Tomita (Kyoto University) and Fumie Tobe (National Institute of Advanced Industrial Science and Technology) for excellent technical assistance. This work was supported by Grants-in-Aid for Scientific Research on Priority Areas (19060012 and 19060016 to T.A.) from the Ministry of Education, Culture, Sports, Science, and Technology of Japan, Grants-in-Aid for the Japan Society for the Promotion of Science Fellows (22-6127 to M.N.), a Grant-in-Aid for Young Scientists (B) (21770064 to N.M.) from the Japan Society for the Promotion of Science, Mitsubishi Foundation (to T.A.), and National Institutes of Health Grants GM56006, GM67837, and GM092412 (to S.A.K.).
Glossary
- SAM
shoot apical meristem
- LD
long-day
- AM
axillary meristem
- SD
short-day
- TF
transcription factor
- BiFC
bimolecular fluorescence complementation
- GUS
β-glucuronidase
- EGFP
enhanced green fluorescent protein
- Col
Columbia-0
- ORF
open reading frame
- GST
glutathione S-transferase
AUTHOR CONTRIBUTIONS
M.N., Y.D., S.A.K., M.O.-T., M.E., and T.A. designed the research. M.N., K.K., Y.D., A.H., J.L.P.-P., G.B., and N.M. performed research. M.N., J.L.P.-P, G.B, M.E., and T.A. analyzed data. M.N. and T.A. wrote the article.
References
Fornara, F., de Montaigu, A., and Coupland, G. (2010). SnapShot: Control of flowering in Arabidopsis. Cell 141: 550, 550.e551–552.
Vandesompele, J., De Preter, K., Pattyn, F., Poppe, B., Van Roy, N., De Paepe, A., and Speleman, F. (2002). Accurate normalization of real-time quantitative RT-PCR data by geometric averaging of multiple internal control genes. Genome Biol. 3: RESEARCH0034.
Author notes
Current address: Graduate School of Agricultural and Life Sciences, University of Tokyo, Bunkyo-ku, Tokyo 113-8657, Japan.
Current address: Bioscience and Biotechnology Center, Nagoya University, Chikusa-ku, Nagoya 464-8601, Japan.
Current address: Department of Integrative Biology and Pharmacology, University of Texas Health Science Center at Houston, Houston, TX 77030.
Current address: Dana and David Dornsife College of Letters, Arts, and Sciences, University of Southern California, Los Angeles, CA 90089.
Address correspondence to [email protected].
The author responsible for distribution of materials integral to the findings presented in this article in accordance with the policy described in the Instructions for Authors (www.plantcell.org) is: Takashi Araki ([email protected]).

Some figures in this article are displayed in color online but in black and white in the print edition.

Online version contains Web-only data.

Open Access articles can be viewed online without a subscription.