-
PDF
- Split View
-
Views
-
Cite
Cite
Sara Fuentes, Karin Ljung, Karim Sorefan, Elizabeth Alvey, Nicholas P. Harberd, Lars Østergaard, Fruit Growth in Arabidopsis Occurs via DELLA-Dependent and DELLA-Independent Gibberellin Responses , The Plant Cell, Volume 24, Issue 10, October 2012, Pages 3982–3996, https://doi.org/10.1105/tpc.112.103192
- Share Icon Share
Abstract
Fruit growth and development depend on highly coordinated hormonal activities. The phytohormone gibberellin (GA) promotes growth by inducing degradation of the growth-repressing DELLA proteins; however, the extent to which DELLA proteins contribute to GA-mediated gynoecium and fruit development remains to be clarified. Here, we provide an in-depth characterization of the role of DELLA proteins in Arabidopsis thaliana fruit growth. We show that DELLA proteins are key regulators of reproductive organ size and important for ensuring optimal fertilization. We demonstrate that the seedless fruit growth (parthenocarpy) observed in della mutants can be directly attributed to the constitutive activation of GA signaling. It has been known for >75 years that another hormone, auxin, can induce formation of seedless fruits. Using mutants with complete lack of DELLA activity, we show here that auxin-induced parthenocarpy occurs entirely through GA signaling in Arabidopsis. Finally, we uncover the existence of a DELLA-independent GA response that promotes fruit growth. This response requires GIBBERELLIN-INSENSITIVE DWARF1–mediated GA perception and a functional 26S proteasome and involves the basic helix-loop-helix protein SPATULA as a key component. Taken together, our results describe additional complexities in GA signaling during fruit development, which may be particularly important to optimize the conditions for successful reproduction.
INTRODUCTION
Phytohormones coordinate multiple aspects of plant growth and development, including fruit initiation, which is a momentous step in the dispersal and survival strategies of flowering plants (angiosperms). Fruit initiation has traditionally been attributed to the action of three hormones, namely, auxin, gibberellin (GA), and cytokinins (Gillaspy et al., 1993). Accordingly, application of these hormones either alone or in combination can induce fruit growth even in the absence of fertilization (parthenocarpy) across a wide variety of plant species (Gustafson, 1936; King, 1947; Srinivasan and Morgan, 1996; Vivian-Smith and Koltunow, 1999; Ozga et al., 2002; Ozga and Reinecke, 2003). In recent years, many of the molecular and genetic mechanisms underlying the action of phytohormones in fruit initiation have been identified, uncovering the complexity of this regulatory network. Several lines of evidence have shown that upon fertilization, a seed-originating auxin signal is generated (Rotino et al., 1997; Mezzetti et al., 2004; Dorcey et al., 2009). This fertilization-dependent auxin signal is proposed to upregulate GA biosynthesis, which in turn activates GA signaling in the ovules and valves, thereby stimulating fruit growth (van Huizen et al., 1995; Ngo et al., 2002; Serrani et al., 2008; Dorcey et al., 2009; Ozga et al., 2009).
GA metabolism highlights the complexity of the hormonal regulation of fruit development. GA biosynthesis is tightly regulated through the action of two multigenic families encoding GA 20-oxidases (GA20ox) and GA 3-oxidases (GA3ox) (Chiang et al., 1995; Phillips et al., 1995; Xu et al., 1995). These enzymes catalyze consecutive steps of GA biosynthesis leading to bioactive GA production. GA metabolism also comprises GA-inactivating pathways, and the major GA-inactivating pathway, the 2β-hydroxylation pathway, involves the activity of GA 2-oxidases (GA2ox) (Thomas et al., 1999). GA homeostasis in fruit initiation and development is determined through feedback regulation of GA biosynthesis and catabolism. Whereas expression of most GA20ox and GA3ox genes is downregulated in response to elevated bioactive GA levels or increased GA signaling, the opposite is true for GA2ox gene expression (Hedden and Phillips, 2000; Dill and Sun, 2001; Silverstone et al., 2001; Olszewski et al., 2002; Zentella et al., 2007; Rieu et al., 2008; Achard and Genschik, 2009). Upon fertilization, expression of GA biosynthesis genes was found to be upregulated in the ovules, whereas GA2ox expression was downregulated in ovules and fruit valves (Dorcey et al., 2009).
In addition to GA metabolism, GA signaling is also tightly regulated during fruit development. Central to GA signaling are DELLA proteins, which are localized in the nucleus and characterized by a conserved DELLA motif in their N-terminal domain and a SCARECROW-like C-terminal domain (Peng et al., 1997; Silverstone et al., 1998). DELLAs form part of the wider GRAS family of regulatory proteins (Bolle, 2004) and five DELLA genes have been identified in Arabidopsis thaliana (GA-INSENSITIVE [GAI], REPRESSOR OF GA1-3 [RGA], RGA-LIKE1 [RGL1], RGL2, and RGL3) (Peng et al., 1997; Silverstone et al., 1998; Sanchez-Fernandez et al., 1998; Dill and Sun, 2001; King et al., 2001; Lee et al., 2002; Wen and Chang, 2002). According to the relief of restraint model (Harberd, 2003), DELLA proteins act as growth repressors and GA-mediated DELLA degradation is required to overcome this restraint. In agreement with their function as growth repressors, it has previously been reported that reduction in DELLA activity can promote parthenocarpic fruit growth (Martí et al., 2007; Dorcey et al., 2009).
At low GA levels, DELLA proteins impair the activity of basic helix-loop-helix transcription factors by interacting with their DNA binding domain (de Lucas et al., 2008; Feng et al., 2008). The binding of GA to the GA receptor GIBBERELLIN-INSENSITIVE DWARF1 (GID1) promotes interaction of GID1 with the DELLA domain of DELLA proteins (Griffiths et al., 2006; Ueguchi-Tanaka et al., 2007; Willige et al., 2007; Murase et al., 2008). The GID1-GA-DELLA complex is subsequently recognized by the SCFSLY1/GID2 E3 ubiquitin-ligase complex, which mediates ubiquitination of DELLA proteins. This ubiquitin mark destines the DELLA proteins for degradation via the 26S proteasome, thereby releasing their inhibitory interaction with transcription factors and allowing growth (McGinnis et al., 2003; Sasaki et al., 2003; Dill et al., 2004; Fu et al., 2004; de Lucas et al., 2008; Feng et al., 2008; Arnaud et al., 2010). In recent years, a proteolysis-independent mechanism of DELLA inactivation has also been described, showing that overexpression of GID1 can rescue some of the phenotypes observed in sleepy1 (sly1) mutants without affecting DELLA protein levels (Ariizumi et al., 2008). However, although GA-mediated GID1–DELLA protein interaction is sufficient to stimulate some GA responses, 26S proteasome–mediated DELLA degradation is still required to restore most DELLA-dependent GA responses (Ariizumi et al., 2008). Thus, the core GA responses are still likely to rely on both GID1-mediated GA reception and 26S proteasome–mediated degradation.
Here, we aim to obtain an in-depth understanding of GA signaling in the context of gynoecium and fruit development. To achieve this, we systematically analyzed the role of DELLA proteins by studying fruit development in the Arabidopsis global-DELLA (gai-t6 rga-t2 rgl1-1 rgl2-1 rgl3-4) mutant, which lacks all five DELLA proteins (hereafter referred to as the global mutant). Our results show that DELLA proteins play an important role in determining both reproductive organ size and the size of tissues involved in the promotion of fertilization. We show that the parthenocarpic development observed in emasculated della mutants (i.e., facultative parthenocarpy) can be directly attributed to the constitutive activation of GA signaling, and, based on a detailed genetic analysis, we conclude that the DELLA proteins RGA, GAI, RGL1, and RGL2 play partially redundant roles in the regulation of pistil growth. Our analyses also reveal that auxin-induced parthenocarpic fruit formation occurs upstream of GA signaling and is exclusively dependent on a functional GA signaling machinery. Finally, we describe and characterize a DELLA-independent GA response, which demonstrates that GA signaling during fruit development is more complex than previously described and uncouples a GA response from the action of DELLA proteins.
RESULTS
DELLAs Regulate Gynoecium, Style, and Stigma Development
The Arabidopsis gynoecium (or pistil) is a complex structure, which upon fertilization forms the fruit. It is divided into distinct tissues, such as the stigma, style, and ovary (Figure 1A). These tissues are made up of several different cell types (Roeder and Yanofsky, 2006), and successful reproduction therefore relies on a highly coordinated developmental program to ensure efficient fertilization of the ovules followed by the development and dispersal of the seeds.
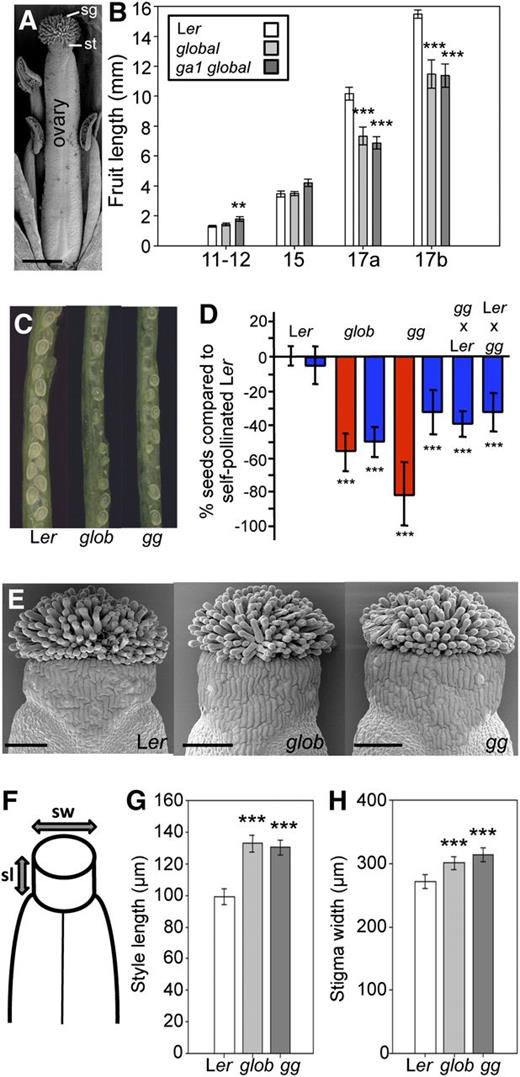
Fertility and Fruit Development in global and ga1 global Mutants.
(A) Scanning electron micrograph of stage 13 Arabidopsis gynoecium with stigmatic tissue (sg), style (st), and ovary indicated. Bar = 400 µm.
(B) Fruit development in Ler, global, and ga1 global mutant plants through stages 11-12, 15, 17a, and 17b. Values significantly different from those of Ler fruits are indicated by asterisks (**P < 0.01 and ***P < 0.001, Student's t test). Error bars: 95% confidence interval (CI). n ≥ 12.
(C) Reduced number of seeds in self-pollinated global (glob) and ga1 global (gg) fruits compared with Ler.
(D) Reduced seed set in self-pollinated, hand-pollinated, and cross-pollinated global (glob) and ga1 global (gg) mutants. Values significantly different from those of self-pollinated Ler are indicated by asterisks (***P < 0.001, Student's t test). Error bars: 95% CI, n ≥ 10. Red, self-pollinated; blue, hand-pollinated.
(E) Scanning electron micrographs of stage 12 gynoecia from Ler, global (glob), and ga1 global (gg). Bars = 100 µm.
(F) Schematic representation of style length (sl) and stigma width (sw) of stage 12 gynoecia.
(G) and (H) Quantification of pistil phenotypes. Style length (G) and stigma width (H) of stage 12 gynoecia from Ler, global (glob), and ga1 global (gg). Values significantly different from those of Ler are indicated by asterisks (***P < 0.001, Student's t test). Error bars: 95% CI, n ≥ 37.
In order to study the role of DELLA proteins throughout the different stages of fruit development, we compared gynoecium, style, and stigma development between the wild type and the quintuple global mutant (lacking all five DELLA proteins; see Methods for details). Several studies have shown that DELLA proteins are involved in positive regulation of GA biosynthesis, suggesting that mutants lacking DELLA proteins have low levels of biologically active GAs (Hedden and Phillips, 2000; Silverstone et al., 2001; Dill and Sun, 2001; Olszewski et al., 2002; Zentella et al., 2007; Achard and Genschik, 2009). Nevertheless, to test whether such reduced GA levels could cause growth abnormalities even in the absence of DELLAs, the sextuple mutant ga1 global in which global is combined with the GA biosynthesis mutant ga1-3 (Sun et al., 1992) was also used throughout this study. In agreement with a previous study, using the quadruple-DELLA mutant lacking GAI, RGA, RGL1, and RGL2 (Cheng et al., 2004), we noticed that ga1 global mutants exhibited slightly longer fruits than the wild type at stages 12 and 15 (developmental stages defined in Smyth et al., 1990) (Figure 1B). After stage 15, the overall fruit length of both global and ga1 global was reduced compared with the wild type (Figure 1B). Reduced fruit length is a phenotype often associated with inefficient fertilization (Vivian-Smith et al., 2001; Cox and Swain, 2006). Accordingly, we found that both mutants contained aborted ovules (Figure 1C), and quantification of seed set in both self- and hand-pollinated global and ga1 global mutants showed significantly reduced seed production (Figure 1D).
It has previously been suggested that absence of RGL1, RGL2, and RGA is sufficient to restore normal seed set in the ga1-3 mutant (Cheng et al., 2004). However, in this study, careful hand-pollination of ga1 global with ga1 global or wild-type Landsberg erecta (Ler) pollen did not restore normal seed set (Figure 1D). Hand-pollination of Ler pistils with ga1 global pollen did not restore normal seed set either (Figure 1D), demonstrating that the reduced fertility in ga1 global is due to both maternally and paternally derived defects. The paternally derived fertility defects observed in ga1 global could be caused either by abnormal pollen tube growth or defects in anther and/or pollen development. Interestingly, both global and ga1 global exhibited expanded style length and widened stigmatic tissue compared with the wild type (Figures 1E to 1H), and it is therefore possible that the DELLA proteins regulate growth of these tissues to allow efficient fertilization. Furthermore, application of GA3 to emasculated wild-type pistils mimicked the style length promotion observed in global plants (see Supplemental Figure 1 online), confirming that style and stigma growth is subject to GA-mediated regulation by DELLA proteins. Based on this phenotypic analysis, we conclude that DELLA proteins are required to mediate optimal growth of fruit tissues and ensure efficient fertilization.
DELLA Genes Show Distinct Expression Profiles throughout Arabidopsis Fruit Development
Previous studies in Arabidopsis have shown that individual DELLA proteins can contribute differently to distinct developmental programs. For example, RGA and GAI proteins function in many vegetative processes, such as stem elongation (Dill and Sun, 2001; King et al., 2001), whereas RGL2 is the main DELLA protein regulating seed germination (Lee et al., 2002; Tyler et al., 2004). Furthermore, it was previously demonstrated that individual DELLA genes follow distinct developmental expression profiles in Arabidopsis (Tyler et al., 2004), which can in some cases provide information about their relative importance in the different developmental programs. Therefore, to obtain a first indication of the role played by individual DELLA proteins in fruit development, we analyzed the expression profiles of the five Arabidopsis DELLA genes by quantitative real-time PCR.
The expression profiles of the DELLA genes followed three different patterns during fruit development (Figures 2A to 2C). Consistent with previous data (Tyler et al., 2004), RGA expression was high and practically constant throughout the stages tested here (Figure 2A). The transcript levels of GAI, RGL1, and RGL2 were relatively high prior to fertilization (stage 11-12) and during fruit elongation (stages 15 and 17a) but decreased when the final fruit length was achieved (stage 17b) (Figure 2B). RGL3 expression was high before fertilization (stages 11 and 12) but decreased soon after until it increased again at the final stage of fruit development (stage 17b) (Figure 2C). Although transcriptional regulation is only one of the many possible mechanisms that control DELLA protein activity (Achard and Genschik, 2009), the fact that expression of DELLA genes follows different profiles throughout Arabidopsis fruit development suggests that different DELLA genes might be important at different stages of fruit growth.
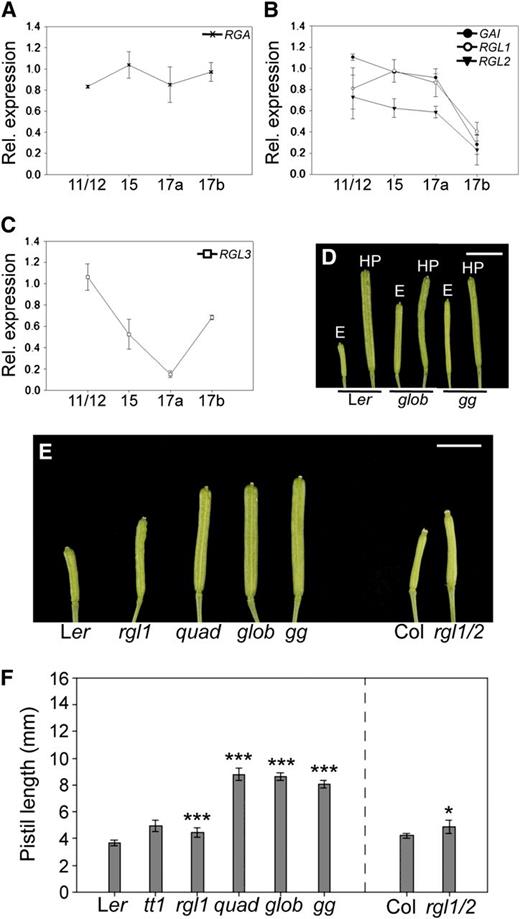
Facultative Parthenocarpy in della Mutants.
(A) to (C) Expression profiles of DELLA genes through stages 11-12, 15, 17a, and 17b of Arabidopsis fruit development. RGA (A), GAI, RGL1, and RGL2 (B), and RGL3 (C). Relative gene expression levels were calculated by quantitative RT-PCR as described in Methods. Error bars indicate sd, n = 3.
(D) Lack of DELLA proteins causes parthenocarpic fruit development in Arabidopsis flowers. Representative emasculated (E) and hand-pollinated (HP) Ler, global, and ga1 global gynoecia 7 DPA. Bar = 5 mm.
(E) Representative emasculated Ler, rgl1, quadruple-DELLA (quad), global (glob), ga1 global (gg), Columbia-0 (Col), and rgl1 rgl2 gynoecia 7 DPA. Bar = 3mm.
(F) Facultative parthenocarpic della mutants. Note that the gai mutation is in the tt1 mutant background. Hence, for mutants with the gai mutation (quad, global, and gg), both tt1 and Ler are included as controls. The rgl1 rgl2 (rgl1/2) mutant is in the Col-0 background. Asterisks indicate values significantly different from control emasculated pistils (*P < 0.05 and ***P < 0.001, Student's t test). Emasculated pistils were measured 7 DPA. Error bars: 95% CI, n ≥ 19.
Lack of DELLA Proteins Causes Facultative Parthenocarpy in Arabidopsis
The role of DELLA proteins in fruit growth repression is clearly noticeable upon global and ga1 global emasculation. Emasculation of wild-type pistils 2 d before anthesis did not promote significant elongation, while emasculated global and ga1 global pistils developed into parthenocarpic fruits more than twice the length of wild-type emasculated pistils (Figure 2D).
This is consistent with previous studies demonstrating that silencing of a single DELLA gene in tomato (Solanum lycopersicum) and lack of four DELLA proteins in Arabidopsis result in parthenocarpic fruit growth (Martí et al., 2007; Dorcey et al., 2009). However, little is known about the relative importance of the individual DELLA proteins in parthenocarpic fruit growth. The expression patterns obtained in our study do not provide sufficient information to elucidate which of the DELLAs have a greater contribution to this process. Therefore, a detailed analysis of the parthenocarpy-conferring capacity of different della mutant combinations was performed.
Analysis of single Arabidopsis della mutants showed that only emasculation of rgl1 resulted in slight but significant fruit elongation (Figures 2E and 2F; see Supplemental Figure 2 online), while in double mutant combinations, only emasculated rgl1 rgl2 pistils grew significantly longer than the wild type (Figures 2E and 2F; see Supplemental Figure 2 online). However, an additive effect was observed upon emasculation of multiple della mutants, and emasculation of quadruple-DELLA, global, and ga1 global pistils resulted in substantial parthenocarpic fruit growth (Figures 2E and 2F; see Supplemental Figure 2 online). Thus, lack of at least four DELLA proteins is required for a sizeable parthenocarpic fruit growth promotion, suggesting a certain degree of functional redundancy. Although RGL3 expression differed from that of the other four DELLA genes (Figure 2C), no significant difference was observed between parthenocarpic quadruple-DELLA and global mutants (Figures 2E and 2F). Thus, GAI, RGA, RGL2, and particularly RGL1 are likely to play the main role in restricting fruit growth in the absence of fertilization with at most a very minor contribution from RGL3.
Tissue Structure in Parthenocarpic della Mutant Fruit Walls Resembles the Structure of Pollinated and GA-Treated Wild-Type Fruits
To analyze the cellular basis underpinning the growth observed in parthenocarpic global and ga1 global fruits, we compared longitudinal sections of wild-type and mutant fruit walls (valves). Arabidopsis valves are composed of six cell layers: an outer exocarp layer, three layers of mesophyll cells, and two endocarp layers (endocarp a [innermost] and endocarp b). In contrast with emasculated wild-type pistils where the different tissue layers were not clearly differentiated, tissue layers in global and ga1 global parthenocarpic fruits were easily distinguishable and resembled the structure of pollinated or GA3-treated wild-type fruits (Figure 3A). By measuring the cell length in each individual tissue layer of emasculated wild-type pistils and parthenocarpic global and ga1 global fruits, we found that lack of DELLA proteins led to significantly longer cells across all tissue layers (Figure 3B; statistical analysis in Supplemental Figure 3A online). Moreover, GA3 treatment of emasculated wild-type pistils induced cell elongation across all tissue layers to a similar extent as pollination and as the emasculation of global and ga1 global pistils (Figure 3B), suggesting that GA-mediated DELLA degradation is responsible for the parthenocarpy observed in these fruits.
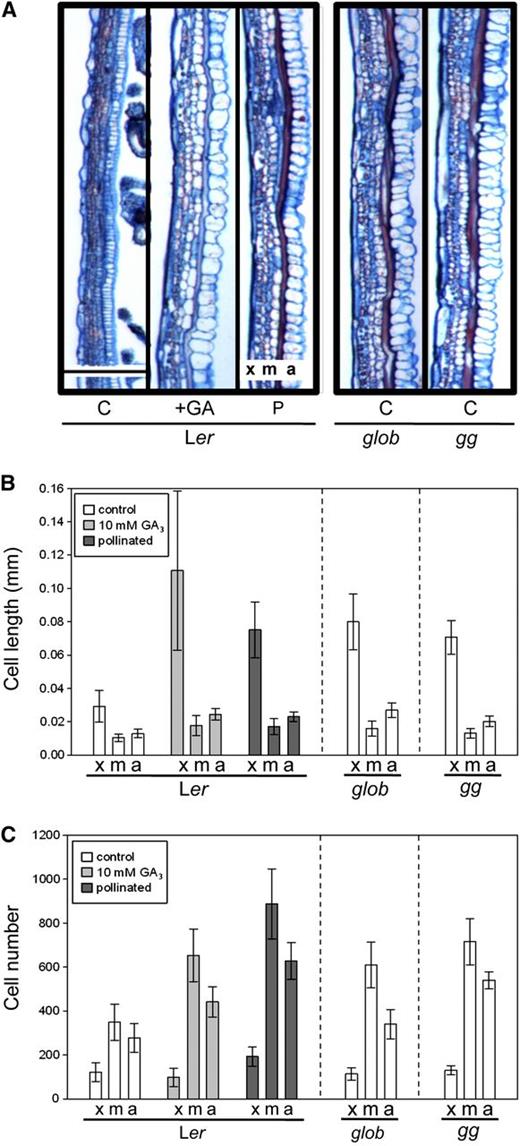
Tissue Analysis of Ler, global Mutant, and ga1 global Mutant Fruits.
(A) Longitudinal sections of Ler, global (glob), and ga1 global (gg) fruits 7 DPA. C, emasculated and control-treated; +GA, emasculated and 10 mM GA3-treated; P, self-pollinated. Bar = 0.2 mm.
(B) Comparison of cell length parallel to the fruit elongation axis in Ler, global (glob), and ga1 global (gg) exocarp (x), mesocarp (m), and endocarp a (a) tissue layers.
(C) Comparison of cell number normal to the fruit elongation axis in Ler, global (glob), and ga1 global (gg) exocarp (x), mesocarp (m), and endocarp a (a) tissue layers. Error bars: 95% CI, n ≥ 4. Statistical analysis is in Supplemental Figure 3 online.
Induction of growth can also occur through cell division. We therefore quantified cell numbers in the individual tissue layers of wild-type and mutant fruits. This analysis showed that only mesophyll and endocarp a tissue layers of parthenocarpic global and ga1 global fruits had significantly higher cell numbers when compared with emasculated wild-type pistils (Figure 3C; see Supplemental Figure 3B online), suggesting that lack of DELLA proteins may have a greater effect on cell elongation than on cell division.
Together, these data demonstrate that GA treatment/lack of DELLA promotes fruit growth in all layers. All layers respond and elongate in a coordinated fashion. However, different layers have specific responses in the extent of cell division versus cell elongation. The exocarp elongates mainly through cell elongation, whereas mesophyll and endocarp a layers both elongate and promote cell division, thus increasing the number of cells. Therefore, absence of DELLA activity in the pistil is sufficient to induce appropriate cell differentiation events similar to those observed upon pollination.
Auxin-Induced Fruit Elongation Requires GA Signaling
Application of the plant hormone auxin has long been known to induce parthenocarpic fruit development in a range of plant species (Gustafson, 1936; Rotino et al., 1997). Several studies have tried to unravel the basis for this effect, and it has been suggested that auxin is produced in the ovules upon fertilization which in turn induces fruit growth by promoting GA biosynthesis in other parts of the fruit (Ozga et al., 2009). The complete lack of DELLA activity in the global and ga1 global mutants provides an excellent system to address this hypothesis directly.
Although emasculation of global and ga1 global pistils resulted in significant parthenocarpic fruit development, these fruits were shorter than pollinated mutant and wild-type fruits (Figure 2D); however, since pollination of mutant pistils results in growth promotion, they clearly have the potential to elongate. First, we tested whether externally applied auxin could stimulate further fruit growth in these mutants. As previously reported, application of 0.1 or 1 mM indole-3-acetic acid (IAA) significantly promoted elongation in emasculated wild-type pistils (Vivian-Smith and Koltunow, 1999; Figure 4A). By contrast, global and ga1 global pistils did not respond to IAA treatment (Figures 4B and 4C). These data suggest that auxin-induced parthenocarpy occurs entirely through DELLA-dependent GA signaling. Second, we investigated whether the parthenocarpy observed in the della mutants is accompanied by changes in auxin concentrations and performed direct measurements of the naturally occurring auxin, IAA. Emasculated pistils from wild-type plants contained fivefold less IAA per mg tissue than did the hand-pollinated fruits (Figure 4D), and this difference is likely at least partly to reflect the high level of auxin produced by developing seeds (Magnus et al., 1997). Hand-pollinated global fruits had lower IAA levels than did hand-pollinated wild-type pistils (Figure 4D), which again can be attributed to the reduced seed number (Figures 1C and 1D). As in the wild type, IAA content in emasculated parthenocarpic global fruits was approximately fivefold lower than in pollinated global fruits, which demonstrates that the facultative parthenocarpy observed in della mutants is not the result of elevated auxin levels, but a direct consequence of DELLA protein absence. Therefore, the data suggest that the mechanism inducing parthenocarpic fruit growth in della mutants is operating downstream of the ovule-derived auxin signal. This finding supports the previously suggested hypothesis that auxin is required to induce GA biosynthesis (Dorcey et al., 2009; Ozga et al., 2009), which in turn promotes DELLA degradation to relieve growth restraint. Interestingly, the parthenocarpic global fruits contained significantly less IAA than did emasculated wild-type pistils (Figure 4D), which could suggest the presence of a feedback loop in which DELLA proteins induce auxin production.
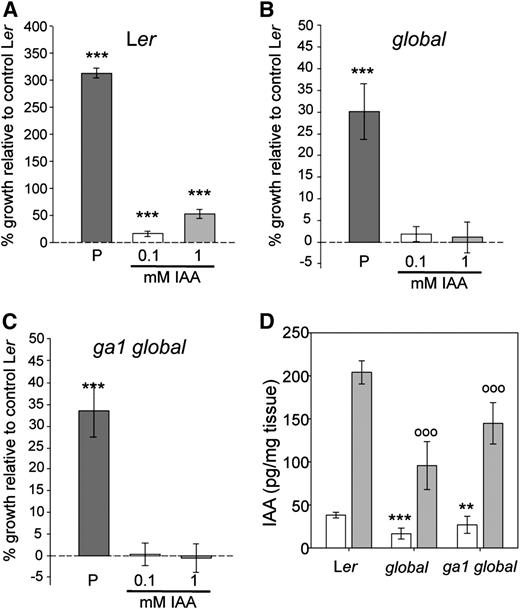
Auxin-Induced Fruit Growth Is Dependent on GA Signaling.
(A) to (C) Percentage growth change in Ler (A), global (B), and ga1 global (C) in response to increasing concentrations of IAA (0.1 and 1 mM). Pistils were measured 7 DPA. Buffer solution was used as the control solution (0 mM). P, self-pollinated. Values significantly different from control pistils in each of the panels are indicated by asterisks (***P < 0.001, Student's t test). Error bars: 95% CI, n ≥ 20.
(D) IAA levels measured in emasculated (white) and hand-pollinated (gray) Ler, global mutant, and ga1 global mutant fruits 7 DPA. Asterisks indicate values significantly different from Ler emasculated pistils (**P < 0.01 and ***P < 0.001, Student's t test), and white circles indicate values significantly different from pollinated Ler fruits (oooP < 0.001, Student's t test). Error bars: 95% CI, n = 3.
Arabidopsis Fruit Growth Is Partially Determined by a DELLA-Independent GA Response
To investigate whether elongation could be further promoted in emasculated global and ga1 global pistils, a series of hormonal treatments were performed. In comparison to tissues such as roots and leaves, relatively high hormone concentrations are required to observe effects in pistils and fruits (Srinivasan and Morgan, 1996; Vivian-Smith and Koltunow, 1999; Dorcey et al., 2009), which is most likely due to a reduced absorption capacity through their waxy surface. To determine the effect of added hormone solutions under our plant growth conditions, dose–response experiments were performed based on previous studies (Vivian-Smith and Koltunow, 1999).
Application of GA3 promoted parthenocarpic fruit development in wild-type Arabidopsis plants and to a greater extent than IAA application (Figure 5A) in agreement with previous findings (Vivian-Smith and Koltunow, 1999). Unexpectedly, significant fruit elongation was also observed in global and ga1 global upon GA3 treatment in a dose-dependent manner (Figures 5B and 5C), revealing the existence of a DELLA-independent GA response.
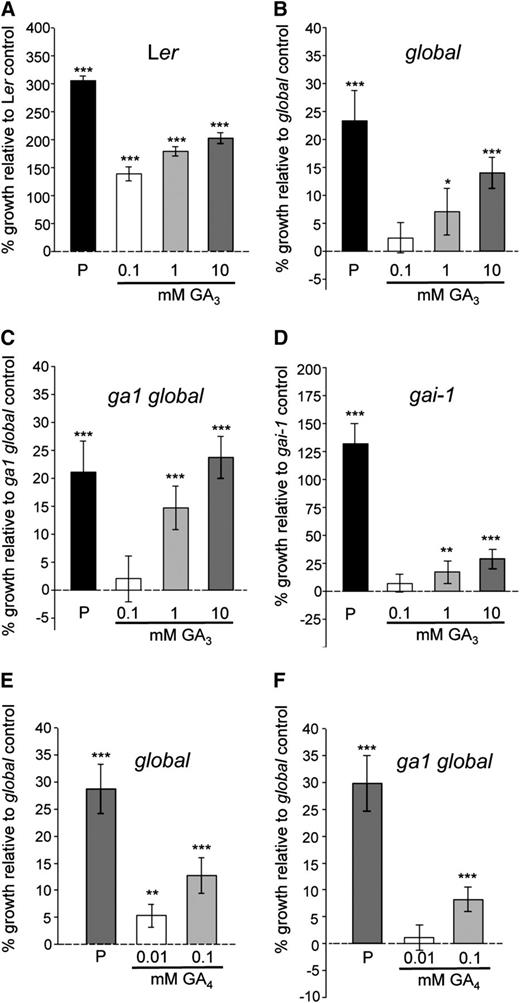
GA3 and GA4 Promote Fruit Elongation in Ler, global, ga1 global, and gai-1.
(A) to (D) Percentage growth increase in emasculated Ler (A), global (B), ga1 global (C), and gai-1 (D) plants in response to increasing concentrations of GA3 (0.1, 1, and 10 mM).
(E) and (F) Percentage growth increase in emasculated global (E) and ga1 global (F) in response to GA4 (0.01 and 0.1 mM).
Pistils were measured 7 DPA. Buffer solution was used as the control solution (0 mM). P, self-pollinated. Values significantly different from control pistils in each of the panels are indicated by asterisks (*P < 0.05, **P < 0.01, and ***P < 0.001, Student's t test). Error bars: 95% CI, n ≥ 16.
GA3 is not a naturally occurring GA in Arabidopsis and, in order to rule out that the growth promotion observed in global and ga1 global pistils could be due to the artificial nature of this compound, pistil growth experiments were repeated with the bioactive GA, GA4 (Ross et al., 2008). Application of GA4 to emasculated global and ga1 global pistils also promoted elongation (Figure 5E), confirming the existence of a DELLA-independent GA response during fruit development. Lower concentrations of GA4 than GA3 were required to promote global and ga1 global pistil elongation (Figures 5B, 5C, 5E, and 5F), which is probably due to GA4 being a naturally occurring GA in Arabidopsis while GA3 is not. Nevertheless, GA3 is the GA that has traditionally been used in most Arabidopsis studies (Vivian-Smith and Koltunow, 1999); thus, in order to allow comparisons with previous studies, GA3 was also employed as the main GA in this study.
To gain further insight into how the newly discovered DELLA-independent GA response might be regulated, we tested the response of gai Dominant (gai-1) mutant pistils to GA3 application. The gai-1 mutant contains a mutated form of the DELLA protein GAI that is resistant to GA-mediated protein degradation (Peng et al., 1997). Hence, the gai-1 mutant does not respond to GAI-dependent GA signaling. In contrast with what has previously been reported (Vivian-Smith and Koltunow, 1999), application of GA3 to gai-1 pistils resulted in significant growth promotion under our experimental conditions (Figure 5D). Although contributions to growth may occur due to degradation of other DELLA proteins in the gai-1 mutant pistils, this result further supports the existence of a DELLA-independent GA response in fruit development as observed in global and ga1 global mutants.
The DELLA-Independent GA Response Requires Both the GID Receptor and the 26S Proteasome and Is SPT Dependent
Previous studies have shown that GID1 receptor-mediated GA perception and 26S proteasome–mediated DELLA degradation are necessary for most GA responses (Fu et al., 2002; Ueguchi-Tanaka et al., 2005; de Lucas et al., 2008; Feng et al., 2008; Gao et al., 2011; Sun, 2011). Thus, whether the newly discovered DELLA-independent GA response also relies on GID1-mediated GA reception and 26S proteasome–mediated degradation was tested.
Three GID1 GA receptors have been identified in Arabidopsis (Griffiths et al., 2006; Nakajima et al., 2006). Genetic studies have shown that the gid1a-1 gid1b-1 gid1c-1 (gid1a/1b/1c) triple mutant exhibits severe GA-related developmental defects, including delayed flowering time (Griffiths et al., 2006), and under our growth conditions, gid1a/1b/1c triple mutant plants only flowered after 2 months of vegetative development. Measurements in vegetative tissue demonstrated that the gid1a/1b/1c mutant accumulates high levels of GA (Griffiths et al., 2006). To facilitate a potential response above a high background, only the highest GA3 concentration was used to determine whether the newly discovered DELLA-independent GA response relies on GID1-mediated GA reception. Application of 10 mM GA3 to gid1a/1b/1c triple mutant pistils did not promote elongation (Figure 6A), suggesting that the DELLA-independent GA response requires GID1-mediated GA reception.
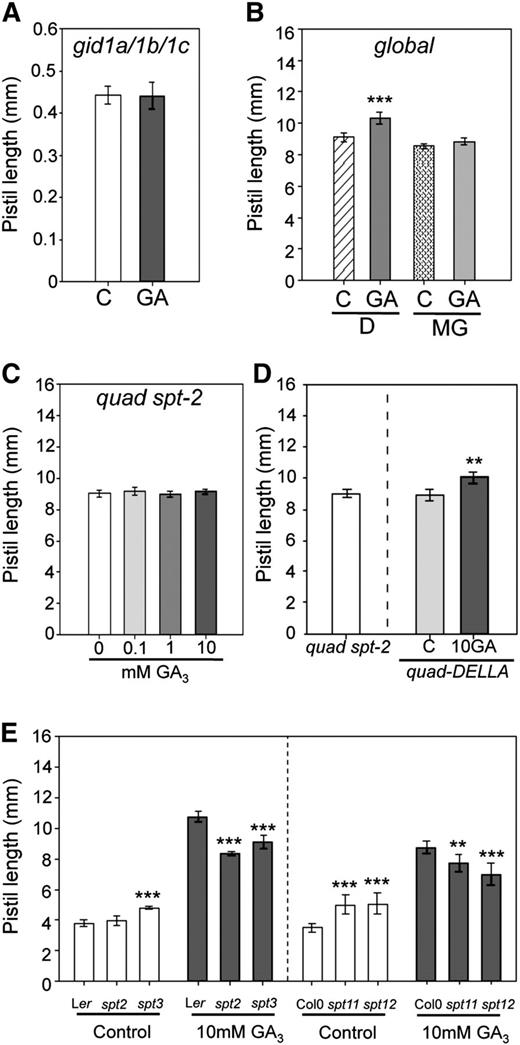
Function of GID1 Receptors, 26S Proteasome, and SPT in the DELLA-Independent GA Response.
(A) Pistil length of gid1a/1b/1c triple mutant in response to 10 mM GA3. Pistils were measured 7 DPA. Buffer solution was used as the control solution. Error bars: 95% CI, n ≥ 12.
(B) Blocking of the DELLA-independent response in global mutant pistils in response to the 26S proteasome inhibitory drug, MG132 (MG). Pistils were measured 7 DPA. Buffer solution was used as the control solution (C) and MG132 was diluted in 0.01% DMSO (D).
(C) quad-DELLA spt-2 (quad spt-2) mutant pistils do not respond to increasing concentrations of GA3.
(D) Pistil length of emasculated quad-DELLA spt-2 mutants compared with quad-DELLA control and 10 mM GA3 treated (**P < 0.01, Student's t test). Error bars: 95% CI, n ≥ 32.
(E) Pistil length of emasculated and GA3-treated pistils of spt-2, spt-3, spt-11, and spt-12 mutants compared with Ler and Col-0 plants (**P < 0.01 and ***P < 0.001, Student's t test). Error bars: 95% CI, n ≥ 18.
In order to determine whether the 26S proteasome is also involved in the DELLA-independent GA response, treatments with MG132 (a 26S proteasome-specific inhibitor) and GA3 were combined (Figure 6B; see Supplemental Figure 4 online). The growth promotion response observed upon 10 mM GA3 application was completely blocked by pretreatment with 100 µM MG132 (Figure 6B; see Supplemental Figure 4 online), demonstrating that the 26S proteasome is a necessary component of the DELLA-independent GA response pathway.
The SLY1 gene in Arabidopsis encodes an F-box protein of the SCFSLY1 complex. Interaction of SLY1 with DELLA proteins promotes their ubiquitination and subsequent degradation in the 26S proteasome (McGinnis et al., 2003; Dill et al., 2004; Feng et al., 2008). In order to determine whether SLY1 is part of the DELLA-independent GA pathway, emasculated sly1-10 pistils were treated with GA (see Supplemental Figure 5 online). A significant growth promotion was observed in GA-treated sly1-10 pistils, which suggests that SLY1 is not required for the DELLA-independent GA response (see Supplemental Figure 5 online). This may not be entirely unexpected as the interaction between F-box proteins and their target proteins confers substrate specificity to the SCF complex (del Pozo and Estelle, 2000; Gagne et al., 2002). Thus, our results support that SLY1 F-box protein is specifically involved in DELLA protein degradation.
In addition to the GID1 receptors and the 26S proteasome, more recently, the basic helix-loop-helix transcription factor SPT has also been shown to be involved in GA responses. SPT not only represses GA biosynthesis in dormant seeds (Penfield et al., 2005) but also interacts with DELLA proteins in yeast two-hybrid assays (Gallego-Bartolomé et al., 2010; Josse et al., 2011). SPT was originally characterized as a major player in style development in the gynoecium (Alvarez and Smyth, 1999; Heisler et al., 2001). More recent studies have revealed that SPT also functions redundantly with other factors to promote fruit opening upon maturity in a process that requires GA signaling (Arnaud et al., 2010; Girin et al., 2011; Groszmann et al., 2011). It is therefore plausible that SPT function contributes to the newly discovered DELLA-independent GA response.
To test the role of SPT, we obtained the quadruple-DELLA spt-2 quintuple mutant (Josse et al., 2011). The spt-2 allele results from an amino acid substitution in the putative DNA binding domain of SPT (Heisler et al., 2001; Penfield et al., 2005) and the mutant phenotype is fully complemented by the wild-type SPT gene (Heisler et al., 2001). Here, we found that in contrast with quad-DELLA, global, and ga1 global mutants, GA3 treatment of emasculated quad-DELLA spt-2 mutants did not promote fruit elongation (Figures 6C and 6D). These data therefore suggest that SPT is required for the DELLA-independent GA response. Moreover, the lack of GA-induced growth in the quad-DELLA spt-2 mutant compared with the quad-DELLA mutant also provides genetic evidence for complete loss of DELLA activity in the quad-DELLA mutant during fruit growth.
Comparison of emasculated spt-3, spt-11, and spt-12 mutant pistils showed that these pistils were significantly longer than wild-type emasculated pistils (Figure 6E) and, hence, partially parthenocarpic. Based on these data and in agreement with other studies (Ichihashi et al., 2010; Sidaway-Lee et al., 2010; Josse et al., 2011), we hypothesize that in the absence of GA, SPT acts as fruit growth repressor. Upon GA treatment, all spt loss-of-function alleles showed decreased responsiveness when compared with wild-type pistils (Figure 6E). These data support that a functional SPT is required for the DELLA-independent GA response.
Since the DELLA-dependent and DELLA-independent GA pathways share key mechanisms such as involvement of GID1 receptors and the 26S proteasome, we wondered whether SPT, like DELLA proteins, is degraded in response to GA. This possibility was investigated by immunoblotting analysis using a transgenic line in which SPT is fused to the hemagglutinin (HA) epitope tag and expression is driven by the 35S promoter (Sidaway-Lee et al., 2010). Immunoblotting with an HA antibody revealed no SPT-HA protein degradation in fruits upon GA3 treatment (Figure 7A).
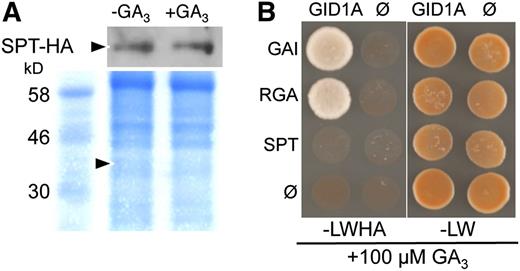
SPT Is Resistant to GA-Mediated Degradation and Does Not Directly Interact with the GID1A GA Receptor
(A) STP-HA is stable upon GA3 treatment in Arabidopsis fruits. Immunoblot showing the stability of SPT-HA upon GA3 treatment in stage 12/15 fruits. Coomassie blue staining of protein gel is shown below (arrowheads: position of SPT-HA at 42 kD).
(B) Yeast two-hybrid interaction using GAI, RGA, and SPT as baits and GID1A as prey. Yeast cells were plated on selective medium (left panel) and control medium (right) in both cases in the presence of 100 µM GA3. Ø indicates empty vector used as negative control.
The DELLA domain of DELLA proteins is essential not only for GA-induced degradation, but also for interaction with the GID1 receptor (Dill and Sun, 2001; Willige et al., 2007). Although SPT protein lacks a DELLA-like domain, the possible interaction between SPT and GID1A receptor was assayed using yeast two-hybrid analysis (Figure 7B). GAI and RGA proteins were used as positive controls for GA-dependent interaction with GID1A (Figure 7B; Willige et al., 2007). However, in the case of SPT, no interaction with GID1A could be detected (Figure 7B). In the absence of GA, DELLA proteins did not interact with GID1 in agreement with previous results, and this was also the case for SPT (Willige et al., 2007; data not shown). Taken together, the lack of SPT–GID1A interaction and the lack of GA-mediated SPT degradation demonstrate that the mode of action of SPT in GA signaling is different from that of the DELLA proteins. These data also suggest that additional molecular components are involved in the DELLA-independent GA signaling pathway.
DISCUSSION
It has previously been shown that DELLA proteins are important regulators of floral organ growth and patterning (Cheng et al., 2004; Tyler et al., 2004; Dorcey et al., 2009; Arnaud et al., 2010). In this article, we further characterize the relative importance of individual DELLA proteins in fruit development. We demonstrate that, although DELLA proteins act as major growth repressors, a DELLA-independent GA response during Arabidopsis fruit development also exists. This newly discovered DELLA-independent GA pathway shares several characteristics with the DELLA-dependent pathway. However, we show that in this new pathway, SPT is a key growth-repressing factor and is regulated differently from DELLA proteins during GA signaling.
DELLA Proteins Repress Gynoecium, Style, and Stigma Growth in Arabidopsis
To investigate the role of DELLA proteins during gynoecium and fruit development in Arabidopsis, global and ga1 global mutants lacking all five DELLA proteins were analyzed throughout the different stages of fruit development. We included both of these mutants in our studies in case the results we obtained in the global mutant were due to abnormal GA levels. However, similar results were obtained with these two mutants throughout.
Detailed characterization of stage 12 gynoecia demonstrated that lack of DELLA proteins results in enlarged style and stigma tissue development (Figure 1). Previous studies have shown that silencing of DELLA activity in tomato also promotes style growth (Martí et al., 2007), and the results described here for Arabidopsis demonstrate that this effect is not limited to fleshy fruit development. Furthermore, style elongation could be phenocopied in wild-type Arabidopsis pistils upon GA treatment (see Supplemental Figure 1 online), supporting the hypothesis that style development is under the control of DELLA-dependent GA signaling.
The importance of DELLA-dependent GA signaling in gynoecium and fruit development is further supported by the observation that emasculation of global and ga1 global pistils promotes parthenocarpic fruit development (Figure 2). It has previously been reported that emasculation of a quadruple-DELLA Arabidopsis mutant also results in fertilization-independent fruit growth (Dorcey et al., 2009). However, despite the numerous studies underlining the functional specialization of individual DELLA proteins (Peng et al., 1997; Silverstone et al., 1998; Dill and Sun, 2001; King et al., 2001; Lee et al., 2002; Tyler et al., 2004; Cao et al., 2005), the role that individual DELLA genes may play during fruit development remains poorly understood.
Transcript level analysis showed that their expression profiles follow three different patterns during fruit development (Figures 2A to 2C), suggesting that different DELLA genes may be important at different stages of fruit growth. For example, RGL3 expression decreases after fertilization but increases when the maximum fruit length is achieved at stage 17b (Figure 2C). Although the rgl3 single mutant has no detectable defect in restricting fruit growth, it is possible that RGL3 functions redundantly with other factors at this stage to determine final fruit length. DELLA activity is subjected to regulation at many different levels (Achard and Genschik, 2009); however, this expression analysis is in accordance with previous studies, which emphasize the relevance of the transcriptional regulation of DELLA genes (Tyler et al., 2004; Oh et al., 2007).
A detailed analysis of the parthenocarpic capacity of different della mutant combinations was also performed in order to understand the relative importance of individual DELLA proteins in the control of fruit initiation (Figure 2; see Supplemental Figure 2 online). After emasculation of single mutant flowers, only rgl1 exhibited significant fruit growth promotion and additional lack of DELLA proteins is required to enhance this effect (Figure 2; see Supplemental Figure 2 online). However, no difference was observed between parthenocarpic quadruple-DELLA and global mutants (Figure 2), suggesting at most a very minor contribution from RGL3 in fruit growth repression. Thus, GAI, RGA, RGL2, and particularly RGL1 are likely to play the main role in the regulation of fruit development.
At the tissue level, initial analysis showed that lack of DELLA proteins in parthenocarpic global and ga1 global fruits resulted in significant cell elongation across all tissue layers (Figure 3). However, only inner tissue layers of parthenocarpic global and ga1 global fruits showed a greater cell number (Figure 3C). Although GA action has traditionally been associated with cell length promotion (Srivastava et al., 1975; Davidonis, 1990; Inada and Shimmen, 2000; Cheng et al., 2004; Ubeda-Tomás et al., 2008), more recently it has also been shown that DELLA growth-repressing action involves the reduction of both cell proliferation and expansion rates in Arabidopsis roots (Achard et al., 2009; Ubeda-Tomás et al., 2009). Based on our observations alone, we are not able to conclude whether lack of DELLA proteins affects cell elongation and division to a similar extent in the different tissue layers or whether there is a primary GA-responsive tissue during fruit development. The use of cell layer–specific attenuation of the GA response could undoubtedly provide the means to answer this question as shown by Ubeda-Tomás et al. (2008), who proved that DELLA-dependent GA signaling in the endodermis is the rate-limiting factor during root elongation.
Auxin Functions Upstream of GA and DELLAs to Induce Fruit Elongation
It has been known for decades that parthenocarpic fruit development can be induced by manipulating hormone levels in fruits, either through direct application or by genetic engineering (Gustafson, 1936; Wittwer et al., 1957; Gillaspy et al., 1993; Vivian-Smith and Koltunow, 1999). One explanation for this effect could be that the added hormone compensates for the hormone normally produced by the seeds. Indeed, elevated levels of GA and auxin have been measured in fruits from plants that exhibit parthenocarpy in nature (Talon et al., 1990). Moreover, ectopic biosynthesis of auxin in seeds through genetic engineering has proven a powerful method to obtain seedless fruits in a number of plant species (Rotino et al., 1997; Ficcadenti et al., 1999; Mezzetti et al., 2004).
The direct measurement of IAA in wild-type, global, and ga1 global fruits reported here shows no elevation of auxin levels in parthenocarpic global and ga1 global fruits. This suggests that the mechanism inducing parthenocarpic fruit growth in della mutants functions downstream of the ovule-derived auxin signal. It has previously been proposed that auxin distribution from the ovule upon fertilization is required to induce GA biosynthesis in the pericarp (Dorcey et al., 2009; Ozga et al., 2009), which in turn would promote DELLA degradation to relieve growth restraint. The data obtained here support this hypothesis.
Since we found that ectopic addition of auxin did not further induce growth in the global and ga1 global mutant backgrounds, it is unlikely that a parallel auxin-promoted route to parthenocarpy exists in Arabidopsis. We therefore propose that Arabidopsis fruit development induced by auxin occurs solely through activation of GA signaling.
The lower levels of IAA recorded in parthenocarpic global fruits compared with emasculated wild-type pistils suggests that DELLAs may be involved in a positive feedback loop to promote auxin biosynthesis. Such a mechanism is plausible, as DELLAs are also known to induce expression of GA biosynthesis genes (Dill and Sun, 2001; Olszewski et al., 2002).
Evidence for a DELLA-Independent GA Response during Fruit Development
Although emasculation of global and ga1 global pistils results in fruit growth promotion, the parthenocarpic fruits do not grow to the same length as seed-bearing siliques (Figures 2D to 2F). This observation suggests that pathways other than DELLA-dependent GA signaling may be involved in the regulation of Arabidopsis fruit development. The generation of a complete della knockout mutant, such as global and ga1 global, allows the identification of DELLA-independent responses. Indeed, application of GA3 or GA4 to emasculated global and ga1 global pistils resulted in significant growth promotion, revealing the existence of a DELLA-independent GA response.
Application of GA3 to dominant gai-1 mutant pistils also promotes elongation, supporting the DELLA independence of the newly discovered response. Although we cannot discard the possibility that the growth promotion observed in gai-1 pistils could be partially mediated by degradation of the remaining functional DELLA proteins, this is unlikely since a GA response in gai-1 has not previously been reported.
The DELLA-Independent GA Response Shares Some Common Components with the DELLA-Dependent Pathway
Degradation of proteins through ubiquitination and relocation to the 26S proteasome is a common feature in most hormone-signaling pathways (Santner and Estelle, 2009). This mechanism requires interaction between specific protein domains often mediated by direct interactions of the hormone with its receptor(s). In agreement with this, our analyses demonstrate that the DELLA-independent GA pathway, similarly to the DELLA-dependent pathway, requires both the 26S proteasome and most likely the GID1 receptors to exert its effect.
It is interesting to notice that application of the 26S proteasome inhibitor MG132 alone to emasculated global and ga1 global pistils also resulted in growth reduction when compared with control-treated pistils (Figure 6B). It has previously been shown that unpollinated gynoecia continue to grow after emasculation and increase in length up to one-half of their original anthesis length (Vivian-Smith and Koltunow, 1999; Carbonell-Bejerano et al., 2010). Thus, our results suggest that blocking the 26S proteasome is likely to affect both the postanthesis growth of unpollinated pistils and the DELLA-independent GA response.
Overall, we can conclude that GID1 receptors and the 26S proteasome are components shared between the DELLA-dependent and DELLA-independent GA pathways. However, we found that the two pathways most likely branch out at the level of SCF complex specificity, since the DELLA-independent pathway did not rely on functional SLY1 F-box protein.
SPT as a Growth Repressor in the DELLA-Independent GA Pathway
Studies of SPT in fruit development have revealed its role in gynoecium patterning and valve margin formation (Alvarez and Smyth, 1999, 2002; Heisler et al., 2001; Girin et al., 2011). Several lines of evidence show that SPT is also involved in GA signaling: SPT represses GA biosynthesis during etiolated hypocotyl growth (Penfield et al., 2005), SPT and DELLA proteins can interact in yeast two-hybrid experiments (Gallego-Bartolomé et al., 2010), and SPT and DELLAs have dual action in the regulation of cotyledon growth (Josse et al., 2011). In this study we show that in contrast with quad-DELLA and global mutants, application of GA3 to emasculated quad-DELLA spt-2 pistils does not result in growth promotion, confirming that SPT also acts as a growth repressor in the DELLA-independent GA pathway. This is further supported by our data that spt loss-of-function mutants are partially parthenocarpic and fail to elongate to the same extent as emasculated wild-type pistils upon GA treatment.
The role of SPT in fruit growth repression is in accordance with recent reports showing that SPT functions as a repressor of leaf and cotyledon growth (Ichihashi et al., 2010; Sidaway-Lee et al., 2010; Josse et al., 2011). Based on its growth-repressing capacity, we hypothesized that the role of SPT in the DELLA-independent GA pathway may be comparable to that of DELLAs in the DELLA-dependent GA pathway. However, in contrast with DELLA proteins, no GA-mediated SPT degradation was observed in pistils. Furthermore, GA3 did not promote GID1–SPT interaction in yeast, suggesting that the DELLA-independent signaling pathway is likely to rely on additional uncharacterized molecular players.
GA Signaling during Fruit Development
The data presented here show that DELLA proteins are vital for appropriate gynoecium and fruit development in Arabidopsis and that fruit growth requires DELLA degradation. This is in accordance with the relief of restraint model, where activation of GA responses requires GA-mediated DELLA protein degradation by the 26S proteasome (Figure 8; Harberd, 2003). Furthermore, our results reveal that auxin-mediated parthenocarpy in Arabidopsis occurs entirely through stimulation of GA signaling.
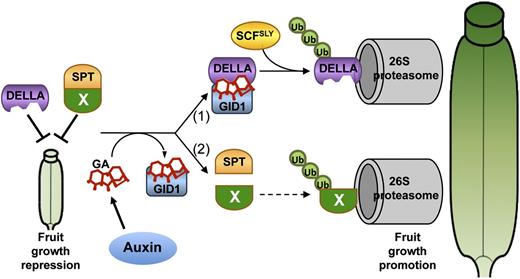
Model for GA Signaling during Fruit Development.
DELLA and SPT (together with an unidentified factor X) act as fruit growth repressors prior to fertilization. Upon fertilization, an ovule-derived auxin signal induces GA biosynthesis, which promotes two pathways: (1) The DELLA-GA-GID1 complex is formed, resulting in fruit growth promotion through 26S proteasome–dependent DELLA degradation; (2) GA perception enables the fine-tuning of fruit growth through a DELLA-independent pathway that relieves SPT repression possibly by dissociation from X, which is then targeted for degradation in the 26S proteasome.
In addition, we identified a GA signaling pathway that is independent of DELLA inactivation but that still requires the GID1 receptors and a functional 26S proteasome. We have also shown that SPT is a key factor in this pathway. However, since SPT itself is not degraded upon GA treatment, we speculate that one or more other factors are involved. As presented in the model in Figure 8, we hypothesize that SPT interacts with an unidentified factor X in the absence of GA to repress growth of the gynoecium. In the presence of GA, this interaction is disrupted and X is degraded in the 26S proteasome similar to DELLAs, allowing the repression to be relieved.
Our results clearly identify the classical DELLA-dependent GA pathway as having the dominant effect on Arabidopsis fruit elongation. However, in analogy with the 26S proteasome-independent mechanism of DELLA inactivation (Ariizumi et al., 2008), the DELLA-independent pathway identified here provides additional opportunities for fine-tuning fruit growth. Fruit development relies on careful coordination of growth between distinct tissue types to allow protection of the fertilized seeds and their timely dispersal. Additional layers of regulatory complexity in this organ may therefore be particularly important to optimize the conditions for successful reproduction.
METHODS
Plant Material and Growth Conditions
The global-DELLA mutant (lacking all five DELLA genes) was obtained as described by Koini et al. (2009). The ga1-3 global-DELLA mutant was isolated from a cross between a ga1-3 quadruple-DELLA mutant (Cheng et al., 2004) and a gai rga rgl1*/−rgl2 rgl3*/− mutant plant. Seeds from both mutants were kindly provided by Liz Alvey. Seeds from quadruple-DELLA spt-2 mutant (Josse et al., 2011) and 35S:SPT-HA (Sidaway-Lee et al., 2010) were kindly provided by Steve Penfield, and we received the gid1a-1 gid1b-1 gid1c-1 seeds (Griffiths et al., 2006) as a kind gift from Stephen Thomas.
The global-DELLA mutant consists of a combination of the gai-t6, rga-t2, rgl1-1, rgl2-1, and rgl3-4 alleles (Koini et al., 2009). Isolation and characterization of the first four alleles are described by Peng et al. (1997 2002), Lee et al. (2002), and Cheng et al. (2004).
In the rgl3-4 allele, the T-DNA insertion is located 1203 nucleotides downstream from the ATG start codon of the RGL3 gene, reducing the length of the predicted translational product by 121 residues. In a transcriptional analysis we were unable to detect a full-length transcript in rgl3-4 RNA isolated from floral tissue, whereas a reduced level of a truncated rgl3-4 was detected (see Supplemental Figure 6 online). This product is terminated in the middle of the conserved PFYRE domain and the SAW domain is missing completely. Based on conservation within the GRAS protein family, this truncated rgl3-4 transcript is unlikely to be translated into a functional DELLA protein (Bolle, 2004). Furthermore, mutant alleles of the barley DELLA gene SLENDER1 that encode proteins lacking the last few amino acids of the full-length protein confer the slender (loss-of-function) phenotype (Chandler et al., 2002). As there is high level of sequence similarity and functional homology between DELLA proteins across angiosperms, it is reasonable to assume that Arabidopsis thaliana DELLA proteins (including RGL3) also require a full-length protein to function.
Plants were grown in a controlled environment room at 20°C under 16-h-light/8-h-dark photoperiod prior to which seeds were stratified at 4°C in darkness for 4 d.
For all emasculation experiments, the first three stage 12 flowers were emasculated. Flowers at this stage were also used for the style and stigma measurements in Figures 1G and 1H. Hand-pollinations were performed after emasculations throughout.
Pistil Emasculation and Hormone Treatments
Pistil emasculations and hormone treatments were performed as described by Vivian-Smith and Koltunow (1999), and dose–response experiments were performed to further determine the optimal hormone concentration. A 1-μL droplet containing 0.1, 1, or 10 mM of GA3 or IAA with 0.01% (v/v) Triton X-100 buffered to pH 7.0 was used to coat emasculated pistils at stage 13 uniformly. Triton X-100 buffer solution (0.01% [v/v]) was used as the control solution. During 26S proteasome inhibition experiments, a 1-μL droplet containing 0.01% DMSO or 100 µM MG132 was applied and let to dry prior to control or GA3 treatment. At least five individual plants were used in each of the hormone treatment experiments.
Final pistil length following pollination, hormone, or control treatment was measured 7 d postanthesis (DPA) once pistils had fully elongated. Pistils were examined under light microscopy using an MZ 16 stereomicroscope (Leica), and the images were captured with a DFC 280 digital camera (Leica). Measurements were performed on captured images using ImageJ software.
Quantitative Real-Time PCR Analysis
Total RNA was extracted from homogenized samples of stage 11-12, 15, 17a, and 17b flowers and fruits using the RNeasy Plant Mini Kit (Qiagen) according to manufacturer's protocol. Three micrograms of total RNA was used to synthesize first-strand cDNA, using M-MLV reverse transcriptase (Invitrogen) and oligo(dT) (18) primers. Gene expression was determined by quantitative real-time PCR (Chromo4 real-time PCR detector; Bio-Rad) using the SYBR Green JumpStart Taq ReadyMix PCR Master Mix (Sigma-Aldrich). Expression levels of DELLA genes were calculated relative to the average expression level of TUBULIN8, UBIQUITIN-CONJUGATING ENZYME9, and UBIQUITIN-CONJUGATING ENZYME10 genes. The gene-specific primer sequences used in this study are shown in Supplemental Table 1 online.
Endogenous IAA Quantification
IAA quantification was performed as described by Sorefan et al. (2009). For each measurement, three independent pooled samples (10 to 20 mg fresh weight) of emasculated and self-pollinated Ler and global mutant gynoecia 7 DPA were collected.
Scanning Electron Microscopy
Fruits were fixed for ∼4 h at 25°C in FAA (50% ethanol, 5% glacial acetic acid, and 3.7% formaldehyde). After critical point drying, tissue was coated with gold and examined in a Philips XL30 FEG microscope using an acceleration voltage of 3 kV.
Histological Analysis
Pistils treated with the control solution, 10 mM GA3, or self-pollinated pistils were collected 7 DPA for histological analysis. Tissue fixation was performed by vacuum infiltration with FAA solution (3.7% formaldehyde, 5% acetic acid, and 50% ethanol) followed by a series of 30-min ethanol washes (50, 60, 70, 80, and 90%). Tissue was stored overnight in 95% ethanol at 4°C. A second round of 30-min washes was performed the following day (3× 100% ethanol, 75% ethanol/25% Histoclear, 50% ethanol/50% Histoclear, 25% ethanol/75% Histoclear, and 3× 100% Histoclear). Tissue was stored overnight in 50% Histoclear/50% Paraplast at 60°C and subsequently embedded through six changes of Paraplast at 60°C. An RM 2255 rotary microtome (Leica) was used to make 8-μm thin longitudinal carpel sections.
To visualize the different tissue layers in the longitudinal carpel sections, tissue staining with Alcian blue 8Gx (staining unlignified tissue blue) and Safranin-O (staining lignified tissue red) was performed. After deparaffinization, sections were treated with Alcian Blue 8Gx/Safranin-O solution (0.05% Alcian Blue 8Gx and 0.01% Safranin-O in 0.1 M acetate buffer, pH 5.0) for 20 min. Longitudinal carpel sections were examined under light microscopy using a MZ16 stereomicroscope (Leica), and the images were captured with a DFC 280 digital camera (Leica). Cell length parallel to the plane of silique elongation was measured on captured images using Image J software (10 to 27 cells per tissue layer per section each from n ≥ 4 sections). The middle part of the fruit was chosen, and measurements were always done in the same area. These data were used to calculate the total number of cells normal to the pistil elongation axis by dividing the mean silique length by the mean cell length normal to the plane of elongation.
Immunoblotting
Approximately 300 mg of plant tissue from flowers at stages 12 to 15 was collected and grinded. One milliliter of protein extraction buffer (50 mM Tris HCl, pH 7.5, 400 mM NaCl, and 1 mM EDTA) with 10 μL Sigma-Aldrich complete protease inhibitor cocktail and 0.5 μL of 200 µM MG132 was mixed thoroughly with the grinded tissue. The debris was removed by centrifugation at 13,000g at 4°C for 10 min. The supernatant was transferred to a fresh Eppendorf tube and stored at −80°C.
After protein extraction and quantification by Bradford assay (Bio-Rad), sample running buffer and reducing agent were added to samples according to the RunBlue protocol (http://www.expedeon.com/TECHNICALRESOURCES/PRODUCTMANUALS/tabid/125/Default.aspx), and this mixture was heated for 10 min at 70°C. Protein extracts were separated by SDS-PAGE (12%) and transferred to a nitrocellulose membrane. A rabbit anti-HA polyclonal Chip-grade antibody (Abcam) was applied, and a goat anti-rabbit IgG antibody (InmunoPure Antibody; Thermo) was used as a secondary antibody to enable immunoreactive polypeptide visualization after film development with Supersignal West Pico Chemiluminescent substrate (Thermo).
Yeast Two-Hybrid Assays
For the yeast two-hybrid assays, the GID1A-BD vector (Willige et al., 2007) used as bait in this study was kindly provided by Claus Schwechheimer. For the activation-domain vectors, the GAI, RGA, and SPT coding regions were amplified by PCR with primers containing SmaI and PstI restriction sites (see Supplemental Table 1 online). The amplicons were cloned in frame between SmaI and PstI sites of pGAD424 to form the prey vectors. After confirming the plasmid quality by sequencing, prey plasmids were transformed into yeast Y187 (-Leu) and bait plasmids into AH109 (-Trp). Prey transformants were selected in SD-L medium (SD broth supplemented with 2% Glc [Formedium], complete supplement mixture -Leu [Formedium], pH 5.8, and 10% agar) while bait transformants were selected in SD-T medium (SD broth supplemented with 2% Glc [Formedium], complete supplement mixture -Trp [Formedium], pH 5.8, and 10% agar).
Yeast transformation was performed as described in the Clontech Laboratories Yeast Protocols Handbook, and the desired transformants were selected on SD-L (prey transformants) or SD-T (bait transformants) media. For each mating combination, a 20-μL aliquot of each of the plasmids was mixed into 160 μL of YPD medium and incubated overnight at 30°C with shaking at 200 rpm. To confirm mating efficiency and protein–protein interactions, 10 μL of each mating culture was plated on SD-LT (growth confirms mating) (SD broth supplemented with 2% Glc [Formedium], complete supplement mixture -Leu -Trp [Formedium], pH 5.8, and 10% agar) and SD-LTHA plates (growth confirms interaction) (SD broth supplemented with 2% Glc [Formedium], complete supplement mixture -Ade -His -Leu -Trp [Formedium], pH 5.8, and10% agar) and incubated upside-down at 30°C.
Accession Numbers
Sequence data from this article can be found in the Arabidopsis Genome Initiative or GenBank/EMBL databases under the following accession numbers: GAI (AT1G14920), RGA (AT2G01570), RGL1 (AT1G66350), RGL2 (AT3G03450), RGL3 (AT5G17490), GA1 (AT4G02780), SPT (AT4G36930), GID1A (AT3G05120), GID1B (AT3G63010), GID1C (AT5G27320), SLY1 (AT4G24210), TUBULIN8 (AT5G23860), UBIQUITIN-CONJUGATING ENZYME9 (AT4G27960), and UBIQUITIN-CONJUGATING ENZYME10 (AT5G5330).
Supplemental Data
The following materials are available in the online version of this article.
Supplemental Figure 1. Style Elongation Is Mediated by GA.
Supplemental Figure 2. della Mutants That Do Not Show Significant Parthenocarpy upon Emasculation.
Supplemental Figure 3. Statistical Analysis of the Tissue Sections of Ler, global Mutant, and ga1 global Mutant Pistils as Shown in Figure 3.
Supplemental Figure 4. Function of 26S Proteasome in the DELLA-Independent GA Response in the ga1-3 global-DELLA Mutant Background.
Supplemental Figure 5. SLY1 Is Not Required for the DELLA-Independent GA Response.
Supplemental Figure 6. Characterization of the rgl3-4 Allele.
Supplemental Table 1. Oligonucleotide Sequences.
Acknowledgments
We thank Steve Penfield for giving us access to quad-DELLA spt-2 mutant seeds prior to publication of these lines and Claus Schwechheimer for kindly providing the GID1A-BD vector used as bait in this study. We also thank Andrew Davis from the John Innes Centre for assistance with photography. We thank Nicolas Arnaud, Thomas Girin, and Susana Sauret-Gueto for critically reading the article, Jan Lohmann for providing useful comments, and Philip Martin for statistical advice. This work was supported by the GRO ISP grant (BB/J004588/1) from the Biotechnology and Biological Sciences Research Council, the John Innes Foundation and by a Marie Curie Fellowship on the Early Stage Training Programme MEST-CT-2005-019727 to S.F.
Glossary
- GA
gibberellin
- Ler
Landsberg erecta
- IAA
indole-3-acetic acid
- HA
hemagglutinin
- DPA
days postanthesis
- CI
confidence interval
AUTHOR CONTRIBUTIONS
S.F. and L.Ø. designed the research. S.F., K.L., K.S., E.A., and L.Ø. performed the research. S.F., K.L., K.S., E.A., N.P.H., and L.Ø. analyzed the data. S.F. and L.Ø. wrote the article.
References
Author notes
Current address: Department of Genetics, Evolution, and Environment, University College London, Gower Street, London WC1E 6 BT, United Kingdom.
Current address: School of Biological Sciences, University of East Anglia, Norwich NR4 7TJ, United Kingdom.
Current address: Department of Plant Sciences, University of Cambridge, Cambridge CB2 3EA, United Kingdom.
Address correspondence to [email protected].
The author responsible for distribution of materials integral to the findings presented in this article in accordance with the policy described in the Instructions for Authors (www.plantcell.org) is: Lars Østergaard ([email protected]).

Online version contains Web-only data.

Open Access articles can be viewed online without a subscription.