-
PDF
- Split View
-
Views
-
Cite
Cite
Ian R Jenkinson, Elisa Berdalet, Wei-Chun Chin, Michel Denis, Haibing Ding, Jizhou Duan, Florence Elias, Igor Emri, Santosh K Karn, Zhuo Li, Alenka Malej, Xavier Mari, Laurent Seuront, Jun Sun, Tim Wyatt, Wuchang Zhang, Oliver Wurl, The rôles of plankton and neuston microbial organic matter in climate regulation, Journal of Plankton Research, Volume 43, Issue 6, November/December 2021, Pages 801–821, https://doi.org/10.1093/plankt/fbab067
- Share Icon Share
Abstract
Plankton and neuston microbes produce organic matter (OM), which accumulates in the sea surface microlayer (SML). Fluxes of heat and momentum exchange across the sea-air interface, as do fluxes of matter, including greenhouse gases, aerosols, microbes (algae, bacteria sensu lato and viruses) and other substances. At least at calm to moderate windspeeds, microbial OM (MOM) in the SML reduces these fluxes. Another MOM fraction, foam, covers a part of the ocean surface. Ocean foam increases mean ocean albedo because it reflects solar radiation, thus cooling the ocean and the Earth. The rheological properties of MOM and the reduction of sea-air fluxes depend on microbial abundance and taxonomic composition, as do the formation and persistence of foam. Genomic regulation of MOM secretion may thus be helping to regulate air-sea fluxes and climate. Unpredictable changes in abundance and taxonomic composition of these microbial communities may be adding uncertainty to global and more local climate. Some of this uncertainty could be mitigated by studying the ecology and genomics of the surface microbial community together with chemical and rheological properties of their secreted MOM and its effects on sea-air fluxes and foam coverage, to incorporate into climate models.
INTRODUCTION
What this review does not cover: existing consensual paradigms
This review does not cover directly existing paradigms about the effects of plankton and neuston microbes on climate regulation.
By current consensus, plankton and neuston microbes have roles in climate regulation by affecting the following properties and processes in the ocean and the atmosphere.
Absorbing heat from solar radiation by their opacity, and transferring it to the water in seconds or less, thus altering vertical temperature distribution, density stratification and mixing in the photic zone (Wu et al., 2007).
Mainly photosynthetic fixation of CO2, carbonates and bicarbonates to organic matter (OM), thereby ultimately diminishing CO2 in the atmosphere and increasing acidity in the ocean; respiration produces the reverse process. OM sinking > OM rising (Mari et al., 2017; but time scales, days to billions of years, are crucial), leading to burial and OM sequestration, thus ultimately removing CO2 from the atmosphere and mitigating ocean acidification (Verdugo et al., 2004; Blain et al., 2007) and partially mitigating anthropogenic CO2 emissions.
While this review covers the effects of marine microbes and microbially produced OM (MOM) on modulating diffusional fluxes of volatile organic carbon (VOC) through the surface microlayer (SML), it does not cover the fate of this VOC in the atmosphere nor any effects on weather or climate.
Some photosynthetic microbes produce abundant dimethylsulphoniopropionate (DMSP), part of which diffuses to the atmosphere as a component of VOC, converts to dimethyl sulphide (DMS) and incorporates into cloud condensation nuclei (CCN). According to the CLAW hypothesis (Charlson et al., 1987), these CCNs increase cloud albedo, thus cooling the Earth and making DMS a negative greenhouse gas (GHG). Whether subsequent work has soundly validated CLAW is hotly debated, but the evidence may be stronger in Polar regions than in the Tropics (Green and Hatton, 2014; Qu et al., 2021; Yang, 2021).
Confusion persists about algorithms required to correct remote sensing estimates of ocean foam coverage and ocean albedo. Therefore, a “ground-truth” set of data on worldwide ocean foam coverage is required independent of atmospheric interference. By excluding atmospheric albedo and aerosols cloud cover and haze from this review, we aim to promote low-altitude surveys of ocean foam and albedo independent of atmospheric effects. This review does not address the effects of MOM on clouds and aerosols.
CH4 is produced from OM, mainly in anaerobic zones, and ultimate releases to the atmosphere (Li et al., 2018). This review does not cover CH4 production or its effect on climate.
Marine microbes participate in regulating climate by fixation and liberation of the GHGs CO2 and CH4 (Shiermeier, 2011). They also produce DMSP, which converts to DMS and other possible climate-changing VOC molecules. Our review concerns how MOM modifies air-sea fluxes in general, but not the subsequent fate of these substances in the atmosphere, nor whether or how they modify climate.
Artificial enhancement of ocean-foam albedo as a climate engineering tool to counter global warming is also not covered. We simply cite some modeling data to help our assessment of existing cooling from natural foam, and we warn against the risks of increasing ocean foam coverage.
What this review covers
While this review does directly cover the existing paradigms in the last subsection, it adds the two following effects modulated by the rheology of ocean MOM.
Rheological effects of MOM on reducing turbulent and molecular diffusion of substances including GHGs across the sea-air interface and through the SML.
Rheological effects of MOM on reducing drainage between the bubbles in ocean surface foams (including whitecaps), stabilizing the foam, increasing ocean-surface albedo and thus reflecting back into Space more solar radiant heat.
Background
By harvesting solar light, plankton and neuston microbes fix the GHG, CO2, reducing it to OM of which they secrete much as dissolved OM (DOM), most of it in the form of carbohydrates, proteins, lipids and nucleic acids (Kurata et al., 2016; Howe et al., 2018). Much of this DOM occurs as complex polymers, which add elasticity and non-Newtonian viscosity to the Newtonian viscosity of the water with its salt (Jenkinson and Sun, 2010). DOM generally, but not always, becomes enriched within the sea-SML, which includes a roughly 60- to 100-μm-thick layer lying just below the sea-air interface (Mustaffa et al., 2020). As well as the abrupt discontinuity at the sea-air interface itself, the SML shows sharp gradients in physical and chemical properties, including viscosity (Zhang, Liu, et al., 2003), surface tension and pH, as well as concentrations of DOM, chlorophyll a, nutrients and dissolved trace metals (Zhang, Liu, et al., 2003). Jenkinson and Biddanda (1995) found that the ratio 3D viscoelastic thickening/chlorophyll a was greater in the SML than in the underlying water (ULW) under calm conditions. These gradients are generally sharpest in the SML (Harvey and Burzell, 1972; Rahlff et al., 2018, 2019), although Rahlff et al. (2019) found sharp change in O2 concentration also as deep as 1.5 mm.
DOM, furthermore, imparts 2D viscoelasticity, in horizontal compression-dilation (Dragčević et al., 1979; Dragčević and Pravdić, 1981) and shearing (Kuhnhenn et al., 2006) of the sea-air surface. Surface viscoelasticity smoothes ripples, for example in oil slicks (Franklin et al., 1774) and in natural slicks (Carlson, 1987). Jenkinson et al. (2015) introduced the term “Thalassorheology” from the Greek “θάλασσα” meaning “sea” and the science of Rheology, itself derived from the Greek, “ρέω,” “to flow,” which is the study of flow and deformation in materials, such as slime, which are neither ideal (Newtonian) liquids nor ideal (Hookean) solids. As part of these thalassorheological changes (Jenkinson et al., 2015), DOM reduces diffusivity near the surface and changes sea-air exchange of matter and energy (Calleja et al., 2009), which is crucial in regulating weather and climate (Veron, 2015). The ocean has absorbed through the SML ~25% of the anthropogenic CO2 produced (Le Quéré et al., 2018), thus mitigating warming in the atmosphere and on land while increasing ocean acidity (Gattuso et al., 2018).
Associated with its rheological properties, biologically produced OM makes the ocean foamier by stabilizing ocean foam (Kesaulya et al., 2008), including whitecaps (Callaghan, 2018). This foam, mostly white in color and thus of high albedo (Dierssen and Garaba, 2020), reflects much more incident solar radiant energy than does the foam-free part of the ocean surface.
We present evidence that microbial blooming variability and the composition and abundance of near-surface microbes therefore alter sea-air exchange of energy and matter by modification of SML viscoelasticity and by altering ocean albedo through foam stabilization. Both these types of modification by OM are still lacking from environmental frameworks of data acquisition and models (Brévière et al., 2015). The Surface Ocean—Lower Atmosphere Study (SOLAS; Brévière et al., 2015) addresses at least three of the nine “planetary boundaries” identified by the International Geosphere Biosphere Programme (IGBP; Steffen et al., 2015) as critical for maintaining the Earth system in a resilient state (Steffen et al., 2015). These three IGBP boundaries are climate change, biogeochemical flows and ocean acidification, which constrain the SOLAS themes: (i) GHGs and the oceans; (ii) air-sea interface and fluxes of mass and energy; (iii) atmospheric deposition and ocean biogeochemistry; (iv) interconnections between aerosols, clouds and marine ecosystems and (v) ocean biogeochemical control on atmospheric chemistry (Brévière et al., 2015). We treat all these themes in the section Albedo values and remote sensing. The syntheses and future work proposed here may be useful to SOLAS, IGBP and the Intergovernmental Panel on Climate Change (IPCC, 2018) to help model, predict and, if necessary, regulate climate dynamics.
Table 1 provides a glossary.
DISSOLVED OM
Ocean OM originates mostly from primary production by planktonic microbes (Eppley and Peterson, 1997; Neu, 2000). This OM consists of particulate OM (POM), consisting of the living bodies of plankton and their detrital remains, and dissolved organic matter (DOM). DOM is distinguished functionally from POM by whether it passes or is retained by a filter of pore size ~0.2–1.0 μm (Supplementary Table 1). Through photosynthesis, CO2 is reduced to organic carbon and thus enters the highly diverse metabolic pathways synthesizing key biological macromolecules, particularly carbohydrates, proteins and lipids (Thornton, 2014). These molecules can be directly excreted (Wyatt and Ribera d'Alcalà, 2006) or released by grazing (sloppy feeding, leakage from fecal pellets; Møller et al., 2003), bacterial (Grossart and Simon, 2007) or viral (Sharoni et al., 2015) lysis, and contribute to the pool of DOM.
Autotrophic micro-, nano- and pico-plankton and -neuston interact with their mixotrophic and heterotrophic equivalents forming microbial ecosystems. They form more-or-less closely interacting networks of metabolically and genomically complementary microbes, termed “interactomes” (Przytycka et al., 2010; Enke et al., 2019; Cook et al., 2020; Takebe et al., 2020; Chaffron et al., 2021). Cutting through its wondrous and still poorly understood biodiversity, we will refer to this interactome as plankton and neuston “microbes.” This review focuses on the effects of this microbial interactome on those mechanical properties and physical processes at the sea-air surface known or believed to regulate climate. Interactions within the interactome are largely outside our scope.
The different molecules of OM produced by microbes are largely produced by specific amplified genes. Amplified genes may produce these molecules directly, but more often, they craft specific enzymes that themselves catalyze the production of other molecules including lipids and polysaccharides. Consortia of genes have evolved to cooperate in the production of many complex molecules and structures. These consortia may co-occur within the same organism or may be placed in interacting organisms of different species or even kingdoms (e.g. eukaryotes, bacteria, archaea and viruses). Such interaction implies communication, which may allow coordination. Planktonic microorganisms that are members of such interactomes may be always spatially separated (e.g. communicating by diffusing molecules), intermittently touching (predation, parasitism), touching throughout their lifetimes (in aggregates or biofilms) or even with microorganisms living inside other microorganisms (eukaryotic, bacterial and viral infections) (Lima-Mendez et al., 2015; Bjorbækmo et al., 2019; D'Alelio et al., 2019). Chaffron et al., (2021) have, moreover, revealed that plankton lives in a complex of interactomes at world-ocean scale, and have investigated how this complex reacts to abiotic forcing as well as to shifts caused by processes within the interactome.
Production and abundance of certain MOM molecules by plankton organisms depend on their abundance, taxonomic composition and physiological state. One example is the polymeric material that increases seawater viscosity and enhances foam production in blooms dominated by the haptophyte alga Phaeocystis globosa (Jenkinson and Biddanda, 1995; Seuront et al., 2006; Kang et al., 2020).
Carbohydrate production by marine prokaryotic communities were investigated by Enke et al. (2019), who found colony-formation times of about 3 days to a week, while communities dominated by the freshwater planktonic cyanobacterium Microcystis showed a formation time from about 2 days to a week (Cook et al., 2020).
Major DOM components and their effects on SML properties
In both the SML and the ULW, the major components of DOM are, in order of abundance, carbohydrates, proteins and lipids (Williams et al., 1986). Apart from nucleic acids, lipids generally comprise the smallest component but their surfactant and 2D rheological activities are greatest relative to their concentration (Frka et al., 2009).
While carbohydrates tend to be polar (hydrophilic), and lipids are mostly non-polar (hydrophobic), proteins tend to be either predominantly non-polar or with some hydrophobic groups present on a hydrophilic surface. This amphiphilic nature allows complex and diverse stereo-interactions of proteins with surrounding molecules and ions (Chen et al., 2015). The 2D compression-dilation rheometry of the sea-air interface, mentioned above, can also be considered as measurement of dynamic surface tension. In this respect, Elliott et al. (2018) reviewed horizontal distribution of air-sea surface tension at scales sub-mm to hundreds of km and suggested ways to integrate such information with data on ocean–atmosphere fluxes in relation to living biological material in the SML. The potential for biological control of climate by altering sea-air surface rheology has been described by Elliott et al. (2019) as “super-Gaian,” where Gaia is described by Lenton et al. (2020) as “Life plus its effects on [the Earth’s] habitability.” Biological feedback through its influence on climate and vice versa may also be subject to evolution by natural selection of species and genes (Darwin, 2003; Dawkins, 2016).
Chemical surfactants and environmental “surfactants”
The three main components of OM (carbohydrates, lipids and proteins) occur sometimes as separate molecules and sometimes as part of macromolecular complexes and aggregates. These aggregates generally bear both polar and non-polar groups and so are often amphiphilic and act as surfactants in the chemical sense, i.e. being surface active (SA). Dissolved and aggregated carbohydrates were classified by Zhou et al. (1998) into three categories, highly SA, “less SA” and “residual” carbohydrates. “Highly SA” carbohydrates were those most readily scavenged by rising bubbles and thus injected into seawater. “Less highly SA” was less readily scavenged, and “residual” carbohydrates were those remaining in seawater after scavenging. Zhou et al. (1998) found highly SA carbohydrates to be enriched in deoxysugars (fucose and rhamnose), whereas less SA and residual carbohydrates were enriched in glucose. Additionally, the highly SA fraction was strongly enriched in covalently bound sulphate.
Such chemical surfactants adsorb from water on to hydrophobic surfaces, such as the sea-air surface, and the measured surfactant activity of OM complexes and aggregates near the sea surface varies markedly in time and space (Boniewicz-Szmyt and Pogorzelski, 2016).
The definition of “surfactant,” which we term “environmental surfactant,” as it applies to the natural environment, has also been widely discussed, both by environmental chemists (Brüggemann et al., 2018) and in the context of accumulation in and near quasi-horizontal surfaces, including the SML (Goldman et al., 1988; Gašparović et al., 2007; Frka et al., 2009; Wurl et al., 2016; Mustaffa et al., 2020).
OM enrichment in the SML
Some components of the OM produced by ocean microbes (Kurata et al., 2016; Howe et al., 2018) become enriched in the SML relative to that in the ULW, taken generally from a depth of 0.1–30 m or even 200 m (Supplementary Table 1).
Even OM that is not chemical surfactant can modify the properties of the ocean-air interface and thus the processes that take place in it (Williams et al., 1986; Frew et al., 1990), because it is buoyant or associated with buoyant organic particles (Mari et al., 2017) or gas bubbles (Woolf, 1993; Asher et al., 1996; Robinson et al., 2019). Other non-polar OM is believed to float by hydrophobic repulsion from the water at or just above the ocean-air interface (van Oss et al., 2005). OM may float abundantly on the sea surface (Wurl and Holmes, 2008), exceptionally as rheologically thick mare sporco (Stachowitsch et al., 1990) or “sea snot” (Balkis-Özdelice et al., 2021). More frequently, OM in and near the SML has been observed to form a more or less broken-up gel (Wurl et al., 2016), increasing the water’s viscous and elastic moduli (Van Vleet and Williams, 1983; Jenkinson and Biddanda, 1995; Zhang, et al., 2003), and thereby reducing turbulent mixing and molecular diffusion, particularly near the sea-air surface (Calleja et al., 2009).
The rheological (visco-elastic) properties and measurement of whole seawater have been reported by (Jenkinson, 1993) for 3D shearing geometry, by Dragčević et al. (1979) for 2D dilation-compression geometry and reviewed by Jenkinson and Sun (2010). Seawater OM also reduces processes such as wave and ripple formation and propagation (Creamer and Wright, 1992); it reduces exchange of gases including O2 (Goldman et al., 1988; Rahlff et al., 2019; Ribas-Ribas et al., 2019) and GHGs such as CO2 (Ribas-Ribas et al., 2018; Mustaffa et al., 2020) and possibly DMS (Calleja et al., 2009; Green and Hatton, 2014). It can also reduce evaporation of water vapor (Wurl et al., 2018) and direct convective exchange of sensible heat (Minnett et al., 2011).
Various adsorbable substances (such as those in a mixture of OM molecules) tend to accumulate in the SML (Ćosović and Vojvodić, 1998) and modify its surface and rheological properties in a competitive manner, each according to its activity [affinity × concentration]. The different molecules and molecular groups each has a different affinity for adsorption to different types of surface (e.g. seawater-atmosphere; seawater-suspended particle). Each molecule and group also has its particular activity for modification of each rheological and surface-associated property and process, such as reduction and damping of gas exchange, waves, ripples or turbulence (Jenkinson et al., 2018; Jenkinson and Sun, 2010). Since the SML is enriched by a more-or-less broken-up gel or biofilm (Wurl et al., 2016), adsorptive interaction among these gel particles, molecules and groups may furthermore interact and therefore complicate their respective activities at the sea–air interface.
Ćosović and Vojvodić (1998) developed a method for estimating surfactant activity in seawater. This method is based on the degree to which OM, by accumulating at the surface between seawater and on an electrode, reduces the alternating current that flows in response to an alternating voltage between the seawater and the electrode under standard conditions. This method has been adopted as a standard protocol for quantifying seawater surfactant activity (Rickard et al., 2019) and is calibrated against the concentration of Triton-X-100 (Teq), a monoionic chemical surfactant (Wurl et al., 2011). Frka et al. (2009) modified the method by using reduction of Cd(II) as a proxy for electric current. Little if any work, however, has yet been reported on inter-calibration of activity coefficients (Ćosović and Vojvodić, 1998) of each OM component on each sea-surface process. Nevertheless, it is mandatory to understand and characterize the activity of these different sea-surface processes (including exchanges) because each process may play a specific role in atmosphere–ocean interactions, and thus each influences the climate in a specific way, as we explain below. If key sea-surface surfactants, whether chemical or environmental, can be sufficiently monitored and managed, they could be potentially powerful tools to regulate the Earth’s climate.
To understand in detail the mechanisms of “surfactant” action on processes that influence climate, the SML rheological properties should be measured as completely as possible over a 5D matrix of the scales, time, mechanical stress and the three dimensions of length (Jenkinson, 2017).
EFFECTS OF BIOLOGICAL OM ON SEA-AIR FLUXES
Figure 1 shows a sketch of gas concentration close the sea-air surface. Surface-associated OM has been shown to reduce sea-air exchange of O2, CO2 and other GHGs (Calleja et al., 2009; Goldman et al., 1988). It is unclear how much of this exchange reduction is due to damping of turbulent diffusion by rheological effects (Goldman et al., 1988) and how much is by OM molecular structure hindering molecular diffusion, particularly if it forms horizontal layers. This OM also reduces exchange of salts, humidity, aerosols and both thermal and mechanical energy, which are all important inputs to storm formation (Veron, 2015). OM in the surface film furthermore damps ripples, gravity waves (Alpers and Hühnerfuss, 1989) and may even affect low-frequency ocean swell (Henderson and Segur 2013). In ocean cyanobacterial slicks, associated increase in temperature and reduction in salinity have been measured during daytime in and just below the SML (Wurl et al., 2018), indicating reduction in evaporation and convective heat exchange over slick areas.

Conceptual view of boundary layer concentration profiles. Resistance to gas transfer is concentrated in the diffusive sublayers. On the left (blue line) is the activity profile for an insoluble gas with the resistance in the aqueous-side diffusive sublayer. On the right (red line) is the profile for a soluble gas with resistance in the air-side diffusive sublayer. Soluble gases have an Ostwald solubility > 100. While an enriched layer of OM is suggested (in red) below the interface, it is unclear what effect this would have on the distribution of gas concentration in the SML, so the contours are left unchanged. C indicates gas concentration; H, Henry coefficient of solubility; subscript a, in air and subscript w, in seawater. Redrawn and reconceptualised from Wanninkhof et al. (2009) and from Jenkinson et al. (2018).
As mentioned in the previous section, each surface exchange process is modified by each component of OM in the SML.
Formalizing, each OM component, OMi = 1,…, m, changes each sea-surface process, Pj = 0,…, n, to give a matrix of modified processes P’i,j. The value i = 0 is reserved for the absence of OM.
The matrix of sea-surface processes modified by the combined activities of all active OMi.
This concept provides a 2D matrix of m × (n + 1) elements for laboratory or in situ empirical testing of the activity of each OM component in modifying each exchange process (Jenkinson, 2017). For simplicity, interactions among molecules and among processes have been ignored here. A major aim of such testing would be to find those elements of the matrix that markedly influence oceanic and atmospheric climates worldwide.
Until recently, measurements of air-sea gas fluxes in relation to OM have been made under calm to moderate winds (0–13 m s−1), but not in heavy weather (Calleja et al., 2009; Goldman et al., 1988; Mari et al., 2017). First in-situ measurements in different oceanic regimes have now resolved that the SML reduces global CO2 fluxes by ~19% (Mustaffa et al., 2020), and that slicks, a sea surface phenomenon characterized by wave-damping and biofilm-like properties, exhibit the strongest suppression.
However, such studies rarely report the tertiary polymeric structure, such as molecular mass or hydrophobicity/hydrophilicity, or the rheological properties of the main components of OM in the SML. MOM, however, shows huge inter- and intra-taxon variations in its rheological properties (Jenkinson, 1986; Petkov and Bratkova, 1996; Kesaulya et al., 2008; Jenkinson et al., 2018; Kang et al., 2020). This suggests that shifts in the taxon composition of microbial assemblages, produced by environmental change (Cheung et al., 2009) or physiological changes during their blooms, could lead to corresponding shifts in some exchange processes and thus of how the ocean affects climate.
Hamed et al. (2020), working on the surface of mucus from pigs’ lungs, have shown that strong air currents (coughing) produce aerosols. The authors measured surface viscosity and surface elasticity of this mucus. Addition of different artificial surfactants enhanced the fluidity of the surface, notably reducing the surface shear elastic modulus G’. The authors found that a balance was needed between surface elastic and surface viscous behaviors to produce the most aerosol production. Comparison of structure and functions of the ocean surface to those of the mucous lining of the mammalian lung may therefore not be entirely fanciful. Both are liquid–air interfaces controlling essential exchange of respiratory gases, and both can produce pathogen-containing aerosols. Both contain mucus as well as surfactants sensu stricto.
OCEAN FOAM
Formation and decay of foams in general
When liquids are agitated strongly enough in the presence of a gas, gas is generally entrained into the liquid and forms bubbles. These bubbles generally rise to the surface. If enough bubbles are produced, and do not burst too quickly, they aggregate to produce a foam of many bubbles. An isolated bubble has two surfaces: between the surrounding liquid and the gas inside the bubble, and another between the surrounding liquid and the gas outside the bubble. Bursting occurs when the liquid drains from the space between these two surfaces. The surfaces touch, and the bubble bursts. The decay of foams is similar but proceeds partly by progressive coalescence of its bubbles into fewer larger bubbles. If the liquid is stabilized, for example by a surfactant sensu stricto, the surfactant binds the liquid to the liquid–gas surfaces and retards drainage of the liquid, this stabilizing the foam. A non-surfactant but inherently more viscous liquid, or one rendered more viscous by DOM, will also drain more slowly and thus also stabilize the foam. See Cantat et al. (2013), particularly Chapter 3.
MOM-enhanced ocean foam increases planetary ocean albedo
Waves spilling or breaking on a water–air interface entrain air bubbles into the water. These bubbles tend to rise and accumulate at the surface, producing patches of foam (whitecaps). Whitecaps start with a growth phase, frequently known as a Stage A. After the breaking or spilling has locally ceased, the whitecap passes into Stage B during which it decays mainly by bursting of the bubbles. This scenario of formation followed by decay occurs both in the laboratory and at sea even in the absence of OM. The presence of at least some types of OM, however, enhances the intensity and duration of the foam produced, particularly during Stage B (Callaghan et al., 2013, 2017).
Callaghan et al. (2013) found that 204 μg L−1 of the surfactant detergent, Triton-X-100 (Teq), prolonged the life of Stage B whitecaps in the laboratory by a factor of 3 compared with surfactant-free filtered seawater. This corresponds approximately to the effect of natural surface-associated OM (i.e. “surfactant”) in an ocean area considered to be of medium productivity according to the criteria of Wurl et al. (2011).
Callaghan (2018) reanalyzed data on individual whitecap coverage C vs. time t at a frequency of 3 or 6 Hz for 552 videos of breaking wave events previously videoed by Callaghan et al. (2012) over 3 days in the coastal Western Atlantic in October. Figure 2 shows a representative plot of C vs. t for a wave-produced whitecap without a right-hand “tail,” characteristic of “clean” water, called a universal curve, compared with one in which a tail dominates values of C at higher values of t. In this “typical” case, the “surfactant” prolongs the duration of the Stage B whitecap, with negligible effect on C vs. t during the Stage A or at the beginning of Stage B. This “surfactant” then prolongs Stage B by stabilizing the foam and, perhaps to some extent, by stabilizing and prolonging the bubble plume that generally underlies each whitecap and tends to feed it with further bubbles (Monahan, 1993, 2020). Figure 2 shows natural prolongation of the whitecap duration from 4 to ~10 s. Bubble plumes and longer periods of foam stabilization are further considered, respectively, in the following sections, A comment on subsurface bubble plumes, and Dramatic examples of sea foam stabilization.
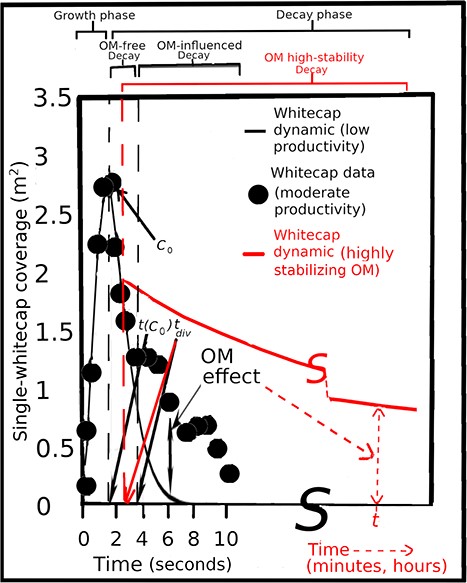
Plot of whitecap coverage Ct vs. time t. The filled circles show the measured foam area time series of an oceanic whitecap from the SPACE08 data set (Callaghan et al., 2012). The black curve is estimated from evolution in the absence of OM effects, which was found following the method described in Callaghan et al., (2017). The left-hand vertical dashed line marks the time t(C0) of the transition from the growth to the decay phases of whitecap foam evolution, when the maximum foam coverage C0 is reached. The right-hand dashed line marks the time tdiv of the transition from OM-free (“universal”) foam decay to OM-influenced foam decay, when OM effects are present and start to become important. The data on OM-influenced foam decay are from observations by Callaghan et al. (2012) under moderate biological productivity. The red curve is intended as an embryonic framework to plan future measurement and modeling of foam decay in the presence of highly stabilizing OM, such as that produced during blooms of the rhaphidophyte Phaeocystis globosa, which is often observed to give foam lifetime of tstab (minutes to hours). In the growth phase, the slope of Ct vs. t is positive until it reaches a maximum, designated C0. The decay phase then begins and the slope of Ct vs. t becomes negative. The value of this slope is most negative for OM-free, less negative for OM-influenced and least negative for highly OM-stabilized foam. Examples of stabilized foam are shown in Fig. 2 and in Supplementary Video 1. Note the discontinuity in the time axis and in the red curve. Inspired by Fig. 1 in Callaghan (2018), with modifications and additions.
MOM, through its rheological, chemical and surface-active properties (Jenkinson et al., 2015, 2018), interacts with turbulence produced by wind, waves and other processes to enhance ocean coverage by whitecaps (Andreas and Monahan, 2000; Callaghan, 2020) and more persistent (>~1 h) foam (Seuront et al., 2006; Kesaulya et al., 2008; Jenkinson et al., 2015; Shetye et al., 2021).
From a study of total water-surface albedo under ranges of different radiation angles, windspeeds and wavelengths in the visible, Gordon and Jacobs (1977) calculated that the foam on the sea surface is at least as important in determining planetary albedo as the optical properties of the other material in the water. Supporting this contention, and based on their measurements of foam coverage and albedo at different wavelengths, Frouin et al. (1996) concluded that the then-existing imprecision in modeling of sea-surface reflectance caused by foam (foam coverage × foam albedo) exceeded 1%, 10 times more than the precision required for biological models to calibrate remote sensing measurements. Even though Frouin et al.’s dramatic conclusions may have been influenced by their bias toward observing coastal water, we suggest that an important part of this unacceptable imprecision is contributed by variability in MOM and hence in marine microbes.
Anguelova and Webster (2006) published remote sensing estimates of worldwide whitecap coverage compared with values modeled from daily fields of windspeed, recently cited by Jenkinson et al. (2018). Anguelova and Webster’s satellite observations showed much more foam coverage in the Trade Wind zones than values based on previous models, while in subpolar regions, notably the Southern Ocean, satellite-observed values were lower than modeled ones. We (Jenkinson et al., 2020) suggested that the higher values of DOM in the surface layer (particularly the SML) in the tropics (Hansell et al., 2009) might have explained the high measured foam coverage in the Trade Wind zones despite lower wind speeds there, with a possible supplementary explanation being different molecular composition of the DOM in different sea areas, reflecting production by taxonomically different blooming microbes as well as different OM histories after production. More recent research on estimating whitecap coverage and whitecap-related planetary albedo, however, has explained the difficulty in separating the effects of atmospheric aerosols, clouds, whitecaps, ocean spray, subsurface bubble plumes and non-whitecap ocean color (Anguelova, 2020; Cifuentes-Lorenzen and Randolph, 2020; Dierssen and Garaba, 2020; Scanlon et al., 2020), all of which are influenced by microbial abundance, taxonomic composition and physiological state, along with physical and chemical properties of the OM they produce and their efficacy as natural foaming and foam-stabilizing agents. As discussed in the section Albedo values and remote sensing, estimates of worldwide ocean foam coverage and its effect on albedo are still subject to much debate (Anguelova, 2020).
Upward flux and albedo at high windspeeds
As mentioned in the previous subsection, strong winds affect albedo by increasing foam coverage. They also increase sea-air fluxes by lifting foam and spray into the atmosphere, along with the dissolved and particulate material they contain.
Breaking waves eject small water droplets from the ocean surface. These droplets can be transported over large distances and remain in the atmosphere for several days, where they enhance atmospheric scatter of radiation (not treated in this paper), when they evaporate they leave behind sea salt and act as CCN. Large ejected drops mostly remain close to the sea surface and affect sea-air fluxes of momentum and enthalpy, thus enhancing the intensity of tropical cyclones (Veron, 2015).
Many types of non-living and living material are enriched in the SML relative to ULW (Section on OM enrichment in the SML), and some of these are enriched in sea foam even more than in the SML (Rahlff et al., 2021). Wind-blown spray and foam, moreover, inject living organisms from the ocean into the atmosphere. Such microbes include: viruses, bacteria, cyanobacteria and their spores (Hamilton and Lenton, 1998; Tesson et al., 2016; Wiśiewska et al., 2020; Shetye et al., 2021) and fragments (Casabianca et al., 2013) including DNA and toxins of dinoflagellates, such as Ostreopsis c.f. ovata and Karenia breve (Fleming et al., 2007; Dotzek et al., 2010; Ciminiello et al., 2014; Michaud et al., 2018; Rahlff, 2019; Medina-Pérez et al., 2020). Aerosolization of organisms blown in drops from the SML and in wind-blown foam may provide a significant means of dispersal for some species (Thornton, 1999). Hamilton and Lenton (1998), moreover, suggested that hydrophobic surfaces of marine plankton cells could favor ejection in droplets. Indeed, Joux et al. (2006) found that, particularly in high-productivity water, both autotrophic and heterotrophic flagellates became enriched in the SML relative to ULW much more than the corresponding pico cell size fraction. Michaud et al. (2018) showed as well that during an experimental phytoplankton bloom in a 13-m3 tank with breaking waves, marine viruses and bacteria with hydrophobic coatings, including species pathogenic to humans, tended to become more enriched in aerosols than those with hydrophilic coatings. Viruses generally were aerosolized less efficiently than bacteria, and their tendency to be aerosolized was also more sensitive to chemical and biological water compositional changes.
Pathogenic bacteria and proteobacteria have been found in coastal diatom-associated stabilized sea foam (Shetye et al., 2021). These have included the bacteria—Vibrio harveyi and Vibrio rotiferianus that are pathogenic to marine animals, as well as Enterobacter cancerogenus, Vibrio parahaemolyticus, Salmonella enterica, Shigella sonnei and the proteobacterium, Pantoeica sp., that are pathogenic to humans as well. Wind-blown sea foam and its derived aerosols can thus potentially expose coastal human populations to bacterial and proteobacterial pathogens.
Ma et al. (2020) measured in-situ spray flux during a Tropical cyclone. This flux was largely generated by foam disruption at high (10–40 m s−1) windspeeds. During their observations, the relationship between windspeed and both spray quantity and the spectrum of drop size varied markedly. The authors linked this variation with Anguelova and Webster’s (2006) report that at a given windspeed, whitecap cover can vary by a factor of 10 or more. While physical and chemical factors undoubtedly cause a major part of this variation, Callaghan et al. (2012, 2013) and Callaghan (2018) showed that biological OM also causes part of this variation.
A comment on subsurface bubble plumes
Subsurface bubble plumes, already mentioned in the section on Microbially enhanced foam increases planetary ocean albedo, are formed by entrainment of air by breaking waves. Such plumes of bubbles rising mainly by buoyancy, occur beneath ocean whitecaps and participate in whitecap formation. Some bubble plumes, however, occur separately from associated whitecaps, or after their disappearance (Monahan, 1993). The dynamics of bubble plumes are thus an important additional element in whitecap formation and decay (Callaghan, 2018). High water temperature decreases the solubility of gas in the bubbles within plumes produced by breaking waves, thus tending to increase the duration of bubbles and foam coverage (Monahan and Woolf, 1989; Callaghan, 2018). Higher temperature also decreases the Newtonian viscosity of the aquatic component of seawater and thus tends to increase the rising speed of bubbles, reducing the residence times of bubbles in plumes, as well as their volume fraction, thereby reducing the albedo caused by subsurface plumes (Callaghan et al., 2013). It is unclear which of these opposing effects is greater.
Albedo of sea foam
Ocean foam reflects and scatters back upward a proportion of incident solar visible radiation (wavelength approx. from 380 to 750 or 800 nm). Because most foam is white, the proportion reflected or scattered back, known as albedo, is roughly constant over this visible range. Measured albedo values from 0.4 to 0.6 in the visible (here from 400 to 1000 nm, e.g. 0.55 at 400 nm) were reported by Whitlock et al. (1982), while Koepke (1982), reviewing the literature, gave albedo values over the wavelength range from 460 to 1100 nm of 0.55 for foam on “very clear water,” but only ~0.44–0.48 for foam on water rich in suspended sediment. Koepke (1982) also reported albedo of only 0.1 in the infrared at 2000 nm.
Developing from Cifuentes-Lorenzen and Randolph (2020), at a given time t and 2D place (pixel) x within an area of ocean, upwelling radiance from the ocean surface, Lx,t(θ,ϕ,λ) is the spectral distribution of light, or other radiant energy, per unit area, wavelength and solid angle (W m−2 nm−1 sr−1), emerging from the ocean in polar and azimuthal directions θ and ϕ, respectively. Radiance reflectance, Rx,t (sr−1), the ratio of Lx,t(θ,ϕ,λ) to the downwelling spectral irradiance incident on the ocean surface Ex,t(θ,ϕ,λ) (W m−2 nm−1), accounts for changes in the illumination and produces a stable measurement of the ratio of surface reflection to downwelling skylight by the wavy surface including any foam. A Lambertian Equivalent Reflectance can be estimated by multiplying by π and the measurement becomes similar to a dimensionless albedo, hereafter Rx,t,λ.
For each place x, time t and wavelength λ, the difference in albedo of foam compared with that of foam-free ocean can be represented by ΔRx,t,λ (dimensionless). Since, as mentioned above, the albedo of most sea foam changes little over the visible and near infrared (480–1000 nm), differences in λ over this spectral range can be simplified to give ΔRx,t, or ΔR.
As already mentioned in the subsection on Microbially enhanced foam increases planetary ocean albedo, the life of a typical whitecap is divided into two stages, A and B (Monahan, 2020). Stage A represents whitecaps actively being formed by spilling or tumbling waves. When this process ends, the whitecap is in Stage B, during which it progressively decays (Fig. 2).
For foam over the whole spectrum of its thicknesses, (Stages A and B taken together), produced by near-coast breakers, Sinnett and Feddersen (2016) gave albedo values of ≤0.465 over a spectrum passing well into the infrared, 305–2800 nm. Stabeno and Monahan (1986) report albedo values in foam of different thicknesses. They found albedo values of only 0.1 for foam one-bubble thick to 0.5–0.6 for foam 25 bubbles thick, roughly corresponding to whitecaps of Stage B and Stage A, respectively (Monahan and Woolf, 1989; Monahan and Lu, 1990; Anguelova, 2020; Callaghan, 2020; Cifuentes-Lorenzen and Randolph, 2020; Hooker et al., 2020; Monahan, 2020).
While ocean foam is usually white, exceptionally yellow and yellow–green foam has been noted associated with blooms of the dinoflagellates Cochlodinium polykrikoides (Vargas-Montero et al., 2006) and Akashiwo sanguinea (Jessup et al., 2009), respectively, while brown foam has been observed associated with blooms of the diatoms Anaulis australis and Asterionella glacialis (Du Preez et al., 1989). It is not clear to what extent, if any, introduction of microbes, whether colored or not, into sea foam is linked to the ability of laboratory foam to trap micro-swimmers in the films between its bubbles (Roveillo et al., 2020).
Dramatic examples of sea foam stabilization
Given that large inter- and intra-taxon differences occur in the rheological properties of the MOM (Jenkinson, 1986), such inter- and intra-taxon differences may be expected also in the foaming properties of such MOM. Indeed, Seuront et al. (2006) found differences in both seawater rheology and foaming properties characteristic of different stages in the blooms of P. globosa.
Examples of long-lived foam from the North Sea, Atlantic and Mediterranean coasts are shown in Fig 3a–c, respectively. The Suppplementary Video 1 also shows North Sea foam. Other things being equal, microbes and the OM they produce may thus increase ocean albedo through increasing coverage by Stage B whitecaps, at scales up to those of the global-ocean. Figure 3 shows large amounts of foam, the equivalent of Stage B whitecaps, which can persist for several hours at least. Research is needed on the extent, the formation and the duration effect of such foam, as well as its effect on ocean albedo, particularly in the open ocean. Research on the rheology and mechanical behavior of sea foam is also required over a wide range of weather conditions.
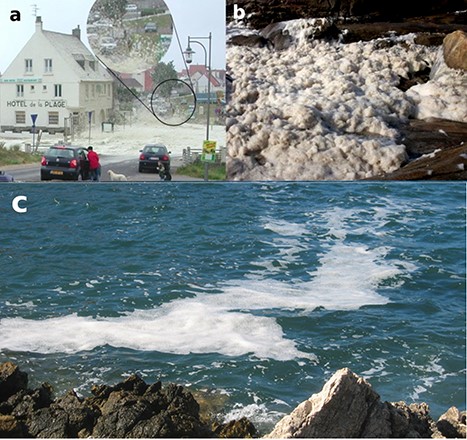
Coastal foam events. (a) Massive foam event at Audresselles, Pas de Calais, France, associated with bloom of P. globosa. Note the flying foam to the right of the hotel, and also that the hotel roof is partly white from wind-blown and sticky foam (insert is enlarged to show better some wind-blown foam aggregates); (b) foam at Cape Silleiro, Galicia, Spain, about a fortnight after gales in early February 2009. Such foam is produced by the action of breaking waves, entraining air into seawater, itself containing polymeric OM produced mainly by plankton and neuston microbes; (c) stabilized floating foam off the Mediterranean coast of Menorca, Spain, 20 August 2013. Photos by Laurent Seuront (a) and Tim Wyatt (b), as in Jenkinson et al. (2018). Photo (c) by Elisa Berdalet. All these foams are believed to have been free of anthropogenic pollution.
Albedo values measured by remote sensing
As mentioned in the Introduction, estimation of albedo by satellite observations falls under SOLAS Theme 4. Satellite observations through the atmosphere have been used to conclude that the Trade Winds areas are associated with coverage of whitecaps W of ~ 3–8%, compared with only ~ 2–4% in the Southern Ocean, where winds are much stronger (Anguelova and Webster, 2006). High levels of near-surface DOM in the Trade Winds zone (Hansell et al., 2009) provide a possible explanation for this, as we suggested previously (Jenkinson et al., 2018), but an alternative explanation may be the difficulty in correcting W for variations in atmospheric haze (Anguelova, 2020).
Albedo of planet Earth is required to understand the thermal balance between the Earth and Space (including the Sun). Maps of remotely sensed ocean albedo estimates of whitecap coverage have been published (Anguelova and Webster, 2006; Salisbury et al., 2013, 2014; Albert et al., 2016; Anguelova and Bettenhausen, 2019). These estimates of sea-surface albedo have been corrected algorithmically for clouds and haze in the atmosphere. Over the years, however, quantitative aspects of these algorithms have had difficulty achieving a stable consensus (Green and Hatton, 2014; Anguelova, 2020), perhaps related to the fact that many of the atmospheric optical properties are themselves influenced by aerosolized sea salt (Hendrickson et al., 2021) and perhaps by the influence of sea-surface microbes on the production and sea-air fluxes of aerosols and cloud condensation substances such as DMS and sulphates. Rigorously separating very low-altitude ocean albedo measurements made from ships (Anguelova, 2020; Cifuentes-Lorenzen and Randolph, 2020; Dierssen and Garaba, 2020), towers (Toba and Koga, 1986; Callaghan et al., 2012), low-flying aircraft (Monahan, 1971) or drones from the confounding effects of the atmosphere may be necessary to understand and estimate both foam coverage and the ocean microbial effects on top-of-the-atmosphere albedo.
In addition to the net upward sea-air flux of DMS, other VOC, including isoprene iodine, are released from the sea-air surface, largely by the action of solar (particularly ultra-violet) radiation on MOM in the SML. Brüggemann et al. (2018) indicated that 23.2–91.9 Tg C year−1 is released from the ocean’s surface as VOC due to interfacial photochemistry within the SML, while Novak and Bertram (2020) estimated this fluxes as between 17.5 and 87.3 Tg C year−1. While the influence of VOC on secondary aerosol formation and its effect on climate regulation is complex and uncertain, the rheological properties of the sea surface are likely themselves to influence this flux. Since this VOC is largely derived from sea-surface MOM, its dynamics may furthermore be linked to those of the ocean microbial interactome, and these dynamics need to be investigated and included in future climate models.
MOM, sea-surface slicks and sunshine reflectivity
In addition to foam, sea-surface slicks damp ripples and thus affect the reflection of solar radiation from the sea surface. Like foam, slicks are formed from concentrations of MOM at the sea surface. Their degree of ripples damping shown in slicks is positively related to their 3D- (Carlson, 1987) and 2D-measured rheological properties. Slicks represent a thickening (units: mm) of the SML Mustaffa et al. (2020) found that CO2 diffusivity was reduced 62% in slicks relative to non-slick surface. In the Mediterranean, Romano (1996) observed slick coverage of 10 and 30% in coastal and offshore waters, respectively. Mustaffa et al. (2020), moreover, reviewing their own observations and those of Takahashi et al. (2009), reported observed slick coverage of 11% in all the following offshore areas: Pacific Ocean, [Tropical] West Pacific, the Atlantic, the North Atlantic [off southern Norway], but 30% in coastal waters in Norwegian fjords. Slick coverage thus reduced CO2 diffusivity overall by 7% in the same offshore areas and by 19% in the coastal area. Since slicks are formed from MOM, detailed properties of their diffusivity may reflect the physico–chemical properties of the molecules and hence of the microbial interactomes that produced them.
Quantitative ocean albedo and conservation of the planetary thermal balance
Apart from suspended sediment in localized areas, such as coastal zones (Dierssen et al., 2006), the dominant effects on ocean color depend on the near-surface concentrations of chlorophyll a (Frouin and Iacobellis, 2002; Jin et al., 2004) and accessory pigments (Dierssen et al., 2006; Sun et al., 2011) in plankton and neuston microbes, as well as backscatter from their intracellular components and external cell coverings such as coccoliths (Kopelevich et al., 2020). The species composition and the physiological state of these microbes (Sonntag and Hense, 2011) are thus dominant in determining foam-free ocean color and albedo as well as foam coverage (detected just above the surface). Anthropogenic detritus, particularly floating, light-colored plastic is also playing an increasing role (Dierssen and Garaba, 2020). Table 2 shows significant components involved in estimating ocean albedo (Monahan, 2020).
Term . | Definition . | References . |
---|---|---|
Surfactant [sensu stricto] | Surfactants are compounds that lower the surface tension (or interfacial tension) between two liquids, between a gas and a liquid or between a liquid and a solid. Surfactants may act as detergents, wetting agents, emulsifiers, foaming agents and dispersants. | (Wikipedia contributors, 2021a) |
“Environmental surfactant,” also known as “surfactant” or surfactant sensu lato | Material, not necessary surfactant sensu stricto, that associates with the air-sea surface and may alter exchange of energy and/or matter. | (Jenkinson et al., 2018) |
Foam | A foam is a dispersion of gas in a liquid. (However, in the present work, we distinguish “foam,” which floats on top of the primary sea-air surface and consist of >50% (v/v) gas, from “bubble plumes,” defined below.) | (Cantat et al., 2013) |
Whitecap | The subset of ocean foam formed by, or having been formed by breaking waves. | |
Stage A whitecap (1) | Actively forming whitecaps | (Bondur and Sharkov, 1982) |
Stage B whitecap (1) | Decaying whitecaps, previously formed as Stage A whitecaps | (Bondur and Sharkov, 1982) |
Bubble plume | A subset of a subsurface 3D patch of “foam” in which the gas phase <50% (v/v). α-plumes are those forming under Stage A whitecaps; β-plumes are those already formed, and possibly decaying, under Stage B whitecaps; γ-plumes are those persisting in the water after their corresponding whitecap has decayed and disappeared. Bubble plumes not associated with wave breaking and their whitecaps also occur, for example those associated with intense turbulence and with Langmuir circulation (Osborn et al., 1992). Near-surface gas supersaturation caused by exceptionally intense photosynthesis in algal blooms has also been observed to produce bubble suspensions, stabilized by OM (Jenkinson and Connors, 1980; Jenkinson, 1986). | (Osborn et al., 1992; Monahan, 1993) |
Albedo | The proportion of the diffuse reflection (backscatter) of solar radiation out of the total solar radiation and measured on a scale from 0, corresponding to a black body that absorbs all incident radiation, to 1, corresponding to a body that reflects all incident radiation. | (Wikipedia contributors, 2021b) |
Term . | Definition . | References . |
---|---|---|
Surfactant [sensu stricto] | Surfactants are compounds that lower the surface tension (or interfacial tension) between two liquids, between a gas and a liquid or between a liquid and a solid. Surfactants may act as detergents, wetting agents, emulsifiers, foaming agents and dispersants. | (Wikipedia contributors, 2021a) |
“Environmental surfactant,” also known as “surfactant” or surfactant sensu lato | Material, not necessary surfactant sensu stricto, that associates with the air-sea surface and may alter exchange of energy and/or matter. | (Jenkinson et al., 2018) |
Foam | A foam is a dispersion of gas in a liquid. (However, in the present work, we distinguish “foam,” which floats on top of the primary sea-air surface and consist of >50% (v/v) gas, from “bubble plumes,” defined below.) | (Cantat et al., 2013) |
Whitecap | The subset of ocean foam formed by, or having been formed by breaking waves. | |
Stage A whitecap (1) | Actively forming whitecaps | (Bondur and Sharkov, 1982) |
Stage B whitecap (1) | Decaying whitecaps, previously formed as Stage A whitecaps | (Bondur and Sharkov, 1982) |
Bubble plume | A subset of a subsurface 3D patch of “foam” in which the gas phase <50% (v/v). α-plumes are those forming under Stage A whitecaps; β-plumes are those already formed, and possibly decaying, under Stage B whitecaps; γ-plumes are those persisting in the water after their corresponding whitecap has decayed and disappeared. Bubble plumes not associated with wave breaking and their whitecaps also occur, for example those associated with intense turbulence and with Langmuir circulation (Osborn et al., 1992). Near-surface gas supersaturation caused by exceptionally intense photosynthesis in algal blooms has also been observed to produce bubble suspensions, stabilized by OM (Jenkinson and Connors, 1980; Jenkinson, 1986). | (Osborn et al., 1992; Monahan, 1993) |
Albedo | The proportion of the diffuse reflection (backscatter) of solar radiation out of the total solar radiation and measured on a scale from 0, corresponding to a black body that absorbs all incident radiation, to 1, corresponding to a body that reflects all incident radiation. | (Wikipedia contributors, 2021b) |
Term . | Definition . | References . |
---|---|---|
Surfactant [sensu stricto] | Surfactants are compounds that lower the surface tension (or interfacial tension) between two liquids, between a gas and a liquid or between a liquid and a solid. Surfactants may act as detergents, wetting agents, emulsifiers, foaming agents and dispersants. | (Wikipedia contributors, 2021a) |
“Environmental surfactant,” also known as “surfactant” or surfactant sensu lato | Material, not necessary surfactant sensu stricto, that associates with the air-sea surface and may alter exchange of energy and/or matter. | (Jenkinson et al., 2018) |
Foam | A foam is a dispersion of gas in a liquid. (However, in the present work, we distinguish “foam,” which floats on top of the primary sea-air surface and consist of >50% (v/v) gas, from “bubble plumes,” defined below.) | (Cantat et al., 2013) |
Whitecap | The subset of ocean foam formed by, or having been formed by breaking waves. | |
Stage A whitecap (1) | Actively forming whitecaps | (Bondur and Sharkov, 1982) |
Stage B whitecap (1) | Decaying whitecaps, previously formed as Stage A whitecaps | (Bondur and Sharkov, 1982) |
Bubble plume | A subset of a subsurface 3D patch of “foam” in which the gas phase <50% (v/v). α-plumes are those forming under Stage A whitecaps; β-plumes are those already formed, and possibly decaying, under Stage B whitecaps; γ-plumes are those persisting in the water after their corresponding whitecap has decayed and disappeared. Bubble plumes not associated with wave breaking and their whitecaps also occur, for example those associated with intense turbulence and with Langmuir circulation (Osborn et al., 1992). Near-surface gas supersaturation caused by exceptionally intense photosynthesis in algal blooms has also been observed to produce bubble suspensions, stabilized by OM (Jenkinson and Connors, 1980; Jenkinson, 1986). | (Osborn et al., 1992; Monahan, 1993) |
Albedo | The proportion of the diffuse reflection (backscatter) of solar radiation out of the total solar radiation and measured on a scale from 0, corresponding to a black body that absorbs all incident radiation, to 1, corresponding to a body that reflects all incident radiation. | (Wikipedia contributors, 2021b) |
Term . | Definition . | References . |
---|---|---|
Surfactant [sensu stricto] | Surfactants are compounds that lower the surface tension (or interfacial tension) between two liquids, between a gas and a liquid or between a liquid and a solid. Surfactants may act as detergents, wetting agents, emulsifiers, foaming agents and dispersants. | (Wikipedia contributors, 2021a) |
“Environmental surfactant,” also known as “surfactant” or surfactant sensu lato | Material, not necessary surfactant sensu stricto, that associates with the air-sea surface and may alter exchange of energy and/or matter. | (Jenkinson et al., 2018) |
Foam | A foam is a dispersion of gas in a liquid. (However, in the present work, we distinguish “foam,” which floats on top of the primary sea-air surface and consist of >50% (v/v) gas, from “bubble plumes,” defined below.) | (Cantat et al., 2013) |
Whitecap | The subset of ocean foam formed by, or having been formed by breaking waves. | |
Stage A whitecap (1) | Actively forming whitecaps | (Bondur and Sharkov, 1982) |
Stage B whitecap (1) | Decaying whitecaps, previously formed as Stage A whitecaps | (Bondur and Sharkov, 1982) |
Bubble plume | A subset of a subsurface 3D patch of “foam” in which the gas phase <50% (v/v). α-plumes are those forming under Stage A whitecaps; β-plumes are those already formed, and possibly decaying, under Stage B whitecaps; γ-plumes are those persisting in the water after their corresponding whitecap has decayed and disappeared. Bubble plumes not associated with wave breaking and their whitecaps also occur, for example those associated with intense turbulence and with Langmuir circulation (Osborn et al., 1992). Near-surface gas supersaturation caused by exceptionally intense photosynthesis in algal blooms has also been observed to produce bubble suspensions, stabilized by OM (Jenkinson and Connors, 1980; Jenkinson, 1986). | (Osborn et al., 1992; Monahan, 1993) |
Albedo | The proportion of the diffuse reflection (backscatter) of solar radiation out of the total solar radiation and measured on a scale from 0, corresponding to a black body that absorbs all incident radiation, to 1, corresponding to a body that reflects all incident radiation. | (Wikipedia contributors, 2021b) |
Components of ocean albedo and some physical and biological factors that affect them
Source . | Physical . | Biological . | References . |
---|---|---|---|
Ocean color | Color of water and salts, suspended sediment | Plankton, principally abundance, taxonomic composition and physiological state of plankton microalgae | (Callaghan, 2018; Cifuentes-Lorenzen and Randolph, 2020) |
Floating foam (whitecaps) | Modulated by windspeed, salinity, water temperature, atmospheric stability, (sea-air temperature difference), fetch, wind duration, wave height, wave age, current velocity. | OM (“surfactant”) concentration; chemical composition including secondary and tertiary polymeric structure; OM vertical near-surface vertical distribution. It originates largely from phytoplankton, and thus depends on phytoplankton abundance, taxonomic composition and physiological state in the recent past. It depends also on biological transformation and utilization, largely by bacteria. | (Koepke, 1984), (Callaghan et al., 2012; Frouin et al., 2001; Scanlon et al., 2020; Sinnett and Feddersen, 2016; Stabeno and Monahan, 1986) |
Near-surface bubble plumes | Qualitatively modulated by the same factors as floating whitecaps. | Qualitatively as for floating whitecaps. Closely related to whitecap formation. | (Monahan, 1993, 2020; Cifuentes-Lorenzen and Randolph, 2020) |
Neuston, notably floating cyanobacteria. | Modified by winds and currents | Abundance, taxonomic composition and physiological state. | (Sieburth and Connover, 1965; Sonntag and Hense, 2011) |
Floating macroalgae (green and golden tides). | Modified by winds and currents | Abundance, taxonomic composition and physiological state. | (Liu et al., 2018; Sissini et al., 2017; Smetacek and Zingone, 2013; Zhou et al., 2015) |
Floating anthropogenic materials, notably light-colored plastic | Modified by winds and currents, as well as mechanical, chemical and photo-degradation. | Anthropogenic pollution, modified by biofouling and biodegradation | (Dierssen and Garaba, 2020) |
Ocean spray | Qualitatively as for floating whitecaps, mainly at high windspeed. | Qualitatively as for floating whitecaps. | (Veron, 2015) |
Ocean-derived atmospheric aerosols | Qualitatively as for floating whitecaps, particularly after history of high windspeed Possible effects of the production of cloud-condensation nuclei through the CLAW hypothesis. | Qualitatively as for floating whitecaps. Production of DMSP by plankton and neuston microbes may also be important. | (Green and Hatton, 2014; Ianora et al., 2011; Meskhidze and Nenes, 2006) |
Source . | Physical . | Biological . | References . |
---|---|---|---|
Ocean color | Color of water and salts, suspended sediment | Plankton, principally abundance, taxonomic composition and physiological state of plankton microalgae | (Callaghan, 2018; Cifuentes-Lorenzen and Randolph, 2020) |
Floating foam (whitecaps) | Modulated by windspeed, salinity, water temperature, atmospheric stability, (sea-air temperature difference), fetch, wind duration, wave height, wave age, current velocity. | OM (“surfactant”) concentration; chemical composition including secondary and tertiary polymeric structure; OM vertical near-surface vertical distribution. It originates largely from phytoplankton, and thus depends on phytoplankton abundance, taxonomic composition and physiological state in the recent past. It depends also on biological transformation and utilization, largely by bacteria. | (Koepke, 1984), (Callaghan et al., 2012; Frouin et al., 2001; Scanlon et al., 2020; Sinnett and Feddersen, 2016; Stabeno and Monahan, 1986) |
Near-surface bubble plumes | Qualitatively modulated by the same factors as floating whitecaps. | Qualitatively as for floating whitecaps. Closely related to whitecap formation. | (Monahan, 1993, 2020; Cifuentes-Lorenzen and Randolph, 2020) |
Neuston, notably floating cyanobacteria. | Modified by winds and currents | Abundance, taxonomic composition and physiological state. | (Sieburth and Connover, 1965; Sonntag and Hense, 2011) |
Floating macroalgae (green and golden tides). | Modified by winds and currents | Abundance, taxonomic composition and physiological state. | (Liu et al., 2018; Sissini et al., 2017; Smetacek and Zingone, 2013; Zhou et al., 2015) |
Floating anthropogenic materials, notably light-colored plastic | Modified by winds and currents, as well as mechanical, chemical and photo-degradation. | Anthropogenic pollution, modified by biofouling and biodegradation | (Dierssen and Garaba, 2020) |
Ocean spray | Qualitatively as for floating whitecaps, mainly at high windspeed. | Qualitatively as for floating whitecaps. | (Veron, 2015) |
Ocean-derived atmospheric aerosols | Qualitatively as for floating whitecaps, particularly after history of high windspeed Possible effects of the production of cloud-condensation nuclei through the CLAW hypothesis. | Qualitatively as for floating whitecaps. Production of DMSP by plankton and neuston microbes may also be important. | (Green and Hatton, 2014; Ianora et al., 2011; Meskhidze and Nenes, 2006) |
Components of ocean albedo and some physical and biological factors that affect them
Source . | Physical . | Biological . | References . |
---|---|---|---|
Ocean color | Color of water and salts, suspended sediment | Plankton, principally abundance, taxonomic composition and physiological state of plankton microalgae | (Callaghan, 2018; Cifuentes-Lorenzen and Randolph, 2020) |
Floating foam (whitecaps) | Modulated by windspeed, salinity, water temperature, atmospheric stability, (sea-air temperature difference), fetch, wind duration, wave height, wave age, current velocity. | OM (“surfactant”) concentration; chemical composition including secondary and tertiary polymeric structure; OM vertical near-surface vertical distribution. It originates largely from phytoplankton, and thus depends on phytoplankton abundance, taxonomic composition and physiological state in the recent past. It depends also on biological transformation and utilization, largely by bacteria. | (Koepke, 1984), (Callaghan et al., 2012; Frouin et al., 2001; Scanlon et al., 2020; Sinnett and Feddersen, 2016; Stabeno and Monahan, 1986) |
Near-surface bubble plumes | Qualitatively modulated by the same factors as floating whitecaps. | Qualitatively as for floating whitecaps. Closely related to whitecap formation. | (Monahan, 1993, 2020; Cifuentes-Lorenzen and Randolph, 2020) |
Neuston, notably floating cyanobacteria. | Modified by winds and currents | Abundance, taxonomic composition and physiological state. | (Sieburth and Connover, 1965; Sonntag and Hense, 2011) |
Floating macroalgae (green and golden tides). | Modified by winds and currents | Abundance, taxonomic composition and physiological state. | (Liu et al., 2018; Sissini et al., 2017; Smetacek and Zingone, 2013; Zhou et al., 2015) |
Floating anthropogenic materials, notably light-colored plastic | Modified by winds and currents, as well as mechanical, chemical and photo-degradation. | Anthropogenic pollution, modified by biofouling and biodegradation | (Dierssen and Garaba, 2020) |
Ocean spray | Qualitatively as for floating whitecaps, mainly at high windspeed. | Qualitatively as for floating whitecaps. | (Veron, 2015) |
Ocean-derived atmospheric aerosols | Qualitatively as for floating whitecaps, particularly after history of high windspeed Possible effects of the production of cloud-condensation nuclei through the CLAW hypothesis. | Qualitatively as for floating whitecaps. Production of DMSP by plankton and neuston microbes may also be important. | (Green and Hatton, 2014; Ianora et al., 2011; Meskhidze and Nenes, 2006) |
Source . | Physical . | Biological . | References . |
---|---|---|---|
Ocean color | Color of water and salts, suspended sediment | Plankton, principally abundance, taxonomic composition and physiological state of plankton microalgae | (Callaghan, 2018; Cifuentes-Lorenzen and Randolph, 2020) |
Floating foam (whitecaps) | Modulated by windspeed, salinity, water temperature, atmospheric stability, (sea-air temperature difference), fetch, wind duration, wave height, wave age, current velocity. | OM (“surfactant”) concentration; chemical composition including secondary and tertiary polymeric structure; OM vertical near-surface vertical distribution. It originates largely from phytoplankton, and thus depends on phytoplankton abundance, taxonomic composition and physiological state in the recent past. It depends also on biological transformation and utilization, largely by bacteria. | (Koepke, 1984), (Callaghan et al., 2012; Frouin et al., 2001; Scanlon et al., 2020; Sinnett and Feddersen, 2016; Stabeno and Monahan, 1986) |
Near-surface bubble plumes | Qualitatively modulated by the same factors as floating whitecaps. | Qualitatively as for floating whitecaps. Closely related to whitecap formation. | (Monahan, 1993, 2020; Cifuentes-Lorenzen and Randolph, 2020) |
Neuston, notably floating cyanobacteria. | Modified by winds and currents | Abundance, taxonomic composition and physiological state. | (Sieburth and Connover, 1965; Sonntag and Hense, 2011) |
Floating macroalgae (green and golden tides). | Modified by winds and currents | Abundance, taxonomic composition and physiological state. | (Liu et al., 2018; Sissini et al., 2017; Smetacek and Zingone, 2013; Zhou et al., 2015) |
Floating anthropogenic materials, notably light-colored plastic | Modified by winds and currents, as well as mechanical, chemical and photo-degradation. | Anthropogenic pollution, modified by biofouling and biodegradation | (Dierssen and Garaba, 2020) |
Ocean spray | Qualitatively as for floating whitecaps, mainly at high windspeed. | Qualitatively as for floating whitecaps. | (Veron, 2015) |
Ocean-derived atmospheric aerosols | Qualitatively as for floating whitecaps, particularly after history of high windspeed Possible effects of the production of cloud-condensation nuclei through the CLAW hypothesis. | Qualitatively as for floating whitecaps. Production of DMSP by plankton and neuston microbes may also be important. | (Green and Hatton, 2014; Ianora et al., 2011; Meskhidze and Nenes, 2006) |
Concern is increasing worldwide about global warming. Political and economic pressure is being exerted to put in place global engineering solutions, in case GHG reduction measures prove insufficient (Gattuso et al., 2018). Recent research (Callaghan et al., 2013; Callaghan, 2018, 2020; Anguelova, 2020) is now building on older findings about direct, close-up measurements of whitecap coverage (Monahan, 1971; Monahan and Ó Muircheartaigh, 1980; Monahan et al., 1983) and whitecap albedo (Koepke, 1984), allowing clearer separation of the effects of atmospheric haze, aerosols and albedo from low-altitude measurements and estimates of whitecap coverage and the ocean foam’s contribution to albedo (Gordon and Wang, 1994; Anguelova, 2020).
According to Callaghan et al. (2008), at the average wind speed over the ocean, ~6.6 m s−1, the ocean is covered by 0.8% with whitecaps. This coverage exists continuously and can exceed 1.5% in regions with prevailing high wind speeds and frequent storms, e.g. in the Southern Ocean. Combining the estimates for global ocean foam coverage by Callaghan et al. (2008) and for the higher estimates by Anguelova and Webster (2006), for the purpose of this review, we assume a global coverage of 1.5%.
The albedo of natural ocean foam is ~0.5 (Stabeno and Monahan, 1986) and the present ocean-wide average foam coverage is ~1.5% (Section on Quantitative ocean albedo and conservation of the planetary thermal balance). Thus, the foam-contributed albedo is about 1.5% |$\times$| 0.5 = 0.75%. This therefore represents enough albedo to counter a 15% of current GHG perturbation, but it is not taken into account by models of global warming (IPCC, 2021) because they neglect marine planktonic microbial production of DOM and foam.
Sometimes, generally associated with phytoplankton blooms, huge amounts of foam, the equivalent of Stage B whitecaps, can persist for several hours or more, with the potential to increase albedo much more (Supplementary Video 1).
The need to separate measurements of ocean foam albedo from those of atmospheric albedo and from interference by clouds and haze
The effects of MOM on atmospheric aerosols are confusing and controversial. Atmospheric aerosols confound modeling of both sea-surface foam and albedo and their measurement by remote sensing, because aerosols change atmospheric albedo and haze, as well as adding albedo of their own. An extensive body of literature exists on the calibration of the necessary corrections of ocean color caused by atmospheric interference, largely using assumptions about the CLAW (Charlson et al., 1987) hypothesis (Anguelova and Webster, 2006; Lee et al., 2009; Salisbury et al., 2013, 2014; Green and Hatton, 2014; Liss and Johnson, 2014; Brévière et al., 2015; Albert et al., 2016). Anguelova (2020) has recently pointed out the difficulty in separating measurement of ocean surface albedo from interference by atmospheric aerosols and clouds. These three factors need to be studied separately with a view to incorporating into algorithms of Earth-space heat dynamics: (i) ocean foam coverage-linked albedo; (ii) marine cloud-linked atmospheric albedo and (iii) interference by marine haze and clouds on the effects of both sea-surface albedo and albedo of low clouds. All these three effects are influenced by marine phytoplankton, probably to different degrees by different types of phytoplankton, so research needs to treat these effects separately as much as possible. In view of the current climate crisis, this needs to be done urgently, and the present review is intended to guide the way forward.
Effect of foam coverage on ocean albedo and the Earth’s temperature
The contribution by ocean foam to the overall ocean albedo, is (Afoam × W), where W is the proportion covered by foam and Afoam is the albedo of the foam. Correspondingly, the contribution by the surface not covered by foam is [Awater × (1 − W)]. Overall, ocean albedo is thus
A = (Afoam × W) + [Awater × (1 − W)].
Foam albedo depends partly on the spectral reflectance in relation to the distribution of bubble sizes (Kokhanovsky, 2004) and on its thickness in terms of number of bubbles (Koepke, 1984). Subsurface bubble plumes also contribute some reflectance (Randolph et al., 2017). The need for simplification, however, has led to “good agreement” for Afoam of 0.4–0.6 and for Awater of 0.03–0.05 (Stabeno and Monahan, 1986; Evans et al., 2010).
Gabriel et al. (2017) studied the effect of adding artificial foam to about a quarter of the world ocean to reduce the earth’s temperature, using the G4FOAM modeled scenario. They assumed a mean background value of A of 0.06. If we assume values of 0.5 and 0.05 for Afoam and Awater, respectively, solving the equation above gives an existing background value of W of 0.022. When A in a quarter of the Earth’s ocean is tripled to 0.18, as in Gabriel et al.’s model, then W in the modified ocean areas would correspond to a value of 0.287. Although this enhanced value of albedo was imposed on a limited part of the ocean, the model predicted it reduced whole-Earth mean temperature by 0.6°C.
Associated with some phytoplankton, and at some stages of their blooms, huge amounts of foam, the equivalent of persistent Stage B whitecaps (Fig. 2), can persist for several hours or more (Fig. 3), with the potential to increase W many-fold (Supplementary Video 1). Changes in abundance and composition of microplankton, whether or not in response to climate change or species introduction, may thus have the potential to change ocean foam coverage and hence the Earth’s thermal dynamics. Foam coverage in relation to microplankton needs to be further investigated, and the associated current uncertainty taken into account in climate models (IPCC, 2021). We, the co-authors, do not support addition of artificial foam to the oceans.
CONCLUSIONS
Different types of MOM in and close to the SML are formed from primary and secondary production mainly by plankton and neuston microbes. MOM differentially affects air-sea exchange of matter and energy and thereby impacts weather and climate. MOM also slows the decay of ocean whitecaps and other ocean foam. This foam is white and reflects solar radiation more than the non-foam water. It thus helps to cool the Earth, and it also depends on the dynamics of marine microbial plankton. To understand these impacts better, the tertiary chemical structures of this MOM in the SML need to be characterized in relation to their rheological and surface properties, as well as to the taxa and the genomes of the plankton and neuston microbial community that produces them, throughout the oceans and at all seasons and in all weathers. Such characterization should be combined with measurements on the exchange of key components of mechanical and thermal energy, of non-living and living matter, as well as both foam production and foam longevity. Uncertainty in microbial blooming may thus be adding uncertainty to models and predictions of weather and climate. Such climate models need to be populated with validated information on the ocean microbial community and how the MOM it produces affects both sea-air exchange processes and the production and longevity of ocean foam.
FUNDING
Chinese Academy of Science Research Fellowship for Senior International Scientists (2009S1-36 to I.R.J.); National Natural Science Foundation of China grant (41876134 to J.S.); Changjiang Scholar Program of Chinese Ministry of Education.
ACKNOWLEDGEMENTS
All authors of this publication participate in the International Working Group on Rheology micro/nanoFluidics and bioFouling in the Ocean and other natural waters (RheFFO WG). The authors have no conflict of interest in respect of this article. We thank two anonymous referees for their meticulous and inspiring comments.
References
Chaffron, S., Delage, E., Budinich, M., Vintache, D., Henry, N. Nef, C., Ardyna, M., Zayed, A. A. et al. (
Jenkinson, I. R. and Connors, P. P. (
Osborn, T., Farmer, D. M., Vagle, S., Thorpe, S. A. and Cure, M. (