-
PDF
- Split View
-
Views
-
Cite
Cite
E W Sawyer, Petrogenesis of Secondary Diatexites and the Melt Budget for Crustal Reworking, Journal of Petrology, Volume 61, Issue 3, March 2020, egaa039, https://doi.org/10.1093/petrology/egaa039
- Share Icon Share
Abstract
This study investigates the petrogenesis of diatexite migmatites and leucogranites in a granulite facies terrain and quantifies the melt budget for it. The anatectic rock types in the Ashuanipi Subprovince are: (1) melt-depleted orthopyroxene metatexite migmatite, (2) secondary diatexite migmatite formed where anatectic melt intruded, entrained and accumulated in the metatexite, and (3) leucogranite. The FeO, MgO, TiO2, Cr, Co and Sc contents of the diatexites are controlled by the fraction of entrained metatextite. However, most diatexites and many leucogranites are richer in (Na2O+CaO) but depleted in K2O relative to an anatectic melt + metatexite mixture. This, and the predominance of plagioclase + orthopyroxene frameworks in the diatexites, indicates loss of fractionated melt. Mass-balance models using the metatexite and compositions of fractionated melts and crystallised solids obtained from simulated crystallisation of the anatectic melt indicate that ‘typical’ diatexite formed by mixing ∼40% metatexite with ∼60% anatectic melt, and then when 8 to 30% crystallised, most (>73%) of the remaining melt was expelled, likely by shear-enhanced compaction. The processes making the diatexites and leucogranites expelled ∼50% of the initial amount of melt; some formed the K2O-rich leucodiatexites and leucogranites in the terrain, but most escaped. A melt budget for the present Ashuanipi surface made by combining mass-balance calculations and the area of each rock type reveals that it once held 3.05 times more melt than was generated there. The adjacent Opinaca Subprovince contains 10 times more leucogranite than partial melting there produced; moreover, its leucogranites are compositionally similar to fractionated melts expelled from the Ashuanipi. Combining these crustal levels and assuming a gradient of 30oC km-1, then ∼400 000 km3 of melt representing >68% of the total generated during crustal reworking in the Ashuanipi remained in the middle crust where temperatures were above the solidus.
INTRODUCTION
New crust is created by the addition of magma from the mantle (Hawkesworth et al., 2010). However, melts from the mantle are mafic, whereas the continental crust is andesitic in bulk composition (Taylor & McLennan, 1985; Rudnick & Gao, 2003). New crust must therefore be modified to become continental crust; this is accomplished through two processes. One changes the bulk composition by the return of high-density, mafic/ultramafic cumulate, or residual, material to the mantle in a delamination process (Tatsumi, 2000; Turcotte & Schubert, 2002). The other process produces a compositionally differentiated continental crust from this, perhaps at a much later date, with the upper part enriched in SiO2, K2O, Na2O, Ba, Cs, Li, Rb, Th, U and Zr relative to the lower (Taylor & McLennan, 1985; Rudnick & Gao, 2003). This step, called crustal reworking, is accomplished by partial melting in the lower crust and the ascent of the felsic melt to higher levels, although erosion and sedimentation also contribute (Hawkesworth et al., 2010).
Crustal reworking requires that newly formed, weak, low-density felsic melt initially dispersed in the stronger, denser solid residue moves from the intergranular pore space, created as the volume of reactants decreases as they melt-back, into a network of channels. These channels must then coalesce upwards in order to maintain a sufficient flux of melt through cooler, subsolidus crust. Granitic melt may eventually ascend sufficiently to form plutons in the upper crust, typically at the brittle to ductile transition. A common conceptual view of this process (e.g. Weinberg, 1999) is of a self-organised, possibly scale-invariant (Brown, 2013) network of linked channels that Bons et al. (2010) termed the rivulets-feeding-rivers (RFR) model. A hierarchical pattern from pores to progressively fewer, but wider, channels is indeed found at the outcrop scale in melt-depleted migmatites (e.g. Sawyer, 2001), but remains to be demonstrated at crustal length-scales (∼20 km). Many representations of crustal reworking (e.g. fig. 5 in Brown, 2013; Hawkesworth et al., 2016) join a lower crustal melt source to an upper crustal repository by a dyke. Such depictions imply that the middle crust is not involved in the crustal reworking process: it is by-passed. This ‘direct passage’ view is challenged by recent studies (Morfin et al., 2013, 2014; Yakymchuk et al., 2013; Diener et al., 2014; Hall & Kisters, 2016; Kruger & Kisters, 2016; Carvalho et al., 2016), which show a significant volume of anatectic melt trapped as injection complexes of innumerable thin sills, dykes and stocks of leucogranite in supra-solidus (T>750oC), typically granulite or uppermost amphibolite facies, middle crust. Morfin et al. (2014) noted that most dykes and sills of leucogranite in their injection complex have evolved compositions, and hence the anatectic melt began to crystallise deeper in the crust, near to where it segregated.
Many details of partial melting and crustal reworking are now reasonably well understood (see summary in Brown, 2013), but some overall aspects are not. This study addresses two of these: (1) what do the compositional and microstructural variations in the rocks derived from anatectic melt (e.g. diatexites and leucogranites) tell us about the processes that occurred as the anatectic melt crystallised in a cooling orogenic crust; and (2) what fraction of the melt made during orogen-scale anatexis and crustal reworking might actually reach the upper crust, and, if a large fraction does not, where is it in the crust?
The rocks studied are from the Ashuanipi and Opinaca subprovinces, two large mid-crustal granulite facies terrains in northeastern Canada. The field relations, microstructure and whole-rock compositions of the migmatites and leucogranites enable the melt-depleted and melt-rich rocks to be identified. Mass-balance models using the compositions of fractionated melts and crystallised solids obtained from the simulated crystallisation of a parent anatectic melt are used in order to investigate the petrogenesis of the diatexites and leucogranites, and in particular to estimate the fraction of crystallised solid, fractionated melt (i.e. ‘melt product’) and entrained wall rock in each. These mass-balance models also indicate how much fractionated melt was expelled as the diatexites and leucogranites formed. Coupling this information to the area fraction of the melt-derived rocks at the present surface obtained by regional mapping yields the 2-D melt budget for the whole terrain. Extending this to three dimensions and comparing how much anatectic melt was produced with how much was retained in the diatexites and leucogranites then constrains how much melt might have reached the upper crust. This allows the overall efficacy of the melt transfer and segregation processes that comprise crustal reworking to be estimated.
REGIONAL CONTEXT
The Ashuanipi Subprovince (Fig. 1) is the largest late Archaean metasedimentary belt in the northeastern Superior Province and connects to the Opinaca Subprovince (Simard, 2008). Both underwent deformation and granulite facies metamorphism at about the same time: 2682 to 2636 Ma (Simard, 2008) and 2666 to 2637 Ma (Morfin et al., 2013), respectively. However, the Ashuanipi represents a slightly deeper, hotter crustal level (P ∼ 7 kbar, T ∼ 850 to 900oC; Guernina & Sawyer, 2003) than the Opinaca (P ∼ 6 kbar, T ∼ 810oC; Morfin et al., 2013). The combination of high temperature and long period (20–30 Ma) at suprasolidus temperatures is characteristic of radioactive heating in a thickened, ‘hot’ orogen (Beaumont et al., 2006; Cavalcante et al., 2018).
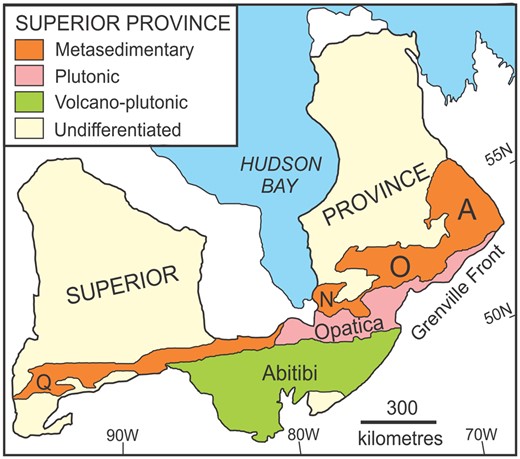
Simplified map of the Superior Province showing the location of the Ashuanipi (A), Opinaca (O), Nemiscau (N) and Quetico (Q) metasedimentary subprovinces.
Siliciclastic rocks dominate in the Ashuanipi Subprovince, with greywacke bulk compositions 10 times more abundant than pelitic. Layers of banded iron formation (BIF) occur, but are volumetrically minor. Mafic rocks were extruded onto, and intruded into, the sediments and are now Hbl + Pl + Cpx ± Opx metamafites (abbreviations for minerals from Whitney & Evans, 2010) and typically occur as transposed layers. Medium- to coarse-grained, mesocratic rocks ranging from Pl + Qz + Bt + Opx metatonalite to Pl + Opx + Cpx + Qz + Bt metadiorite occur sparsely throughout the Ashuanipi and comprise the Desliens Igneous Suite (DIS). The DIS intruded the sediments before regional deformation and granulite facies metamorphism; some of the tonalitic bulk compositions have partially melted.
After metamorphism, the migmatites were intruded by three suites of (<2636 Ma) plutonic rocks, not discussed here (for details, see Simard 2008). Most of the Ashuanipi Subprovince is mapped, and the area percentages covered by the principal rock types obtained from Quebec Geological Survey maps (Lamothe et al., 1998, 2000; Leclair et al., 1998; Thériault & Chevé, 2001; Simard, 2008) are: metatexite migmatite 14.6%; diatexite migmatite 42.9%; anatectic leucogranite 18.4%; BIF + metamafites 4.0%; DIS 3.0%; post-anatectic plutons 17.1%. Anatectic rocks (metatextite, diatexite and leucogranite) predominate and range from 66 to 96% from map to map, with the main variation being in the proportion of post-anatectic intrusions.
MIGMATITES: MORPHOLOGY AND FIELD RELATIONS
Metatextite migmatites
The metatextite migmatites are fine-grained (∼0.7 mm) rocks with a foliation (S1) defined by biotite. Many outcrops preserve bedding (S0) as layers with different mineral assemblages, or modes (representative modes are shown in Table 1); S1 is sub-parallel to S0. The typical mineral assemblage is Pl + Qz + Bt + Opx + Ilm/Mag; minor Grt and Kfs occur in some rocks. The metatexite migmatites of this study are the melt-depleted metagreywackes of Guernina & Sawyer (2003). Leucosomes are generally not abundant (∼15%) in the metatexites (Fig. 2a). Thin (<2 cm) stromatic leucosomes are most common, but low-aspect-ratio forms occur in shear bands and between boudins. The typical mineral assemblage is Pl + Qz ± Kfs with minor Opx and Bt.
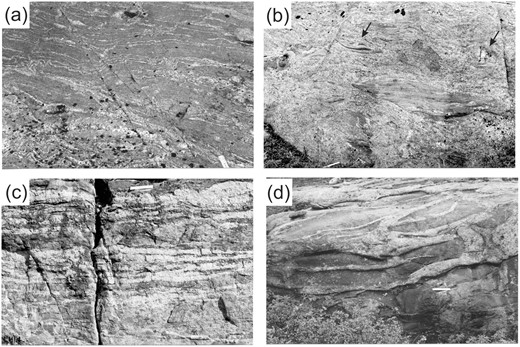
Outcrop appearance of the Ashuanipi anatectic rocks. (a) Greywacke-derived metatexite migmatite with ∼15% leucosome; most are stromatic, but some are located in shear bands or between boudins. (b) Mesocratic diatexite migmatite showing asymmetric schollen of metatexite and vein quartz (upper right corner). (c) Metatexite migmatite containing thin layer-parallel sheets of K-feldspar-rich leucogranite. (d) Metatexite migmatite intruded by anatectic leucogranodiorite. Ruler is 15 cm long.
Sample . | Rock type . | Pl . | Qz . | Opx . | Bt . | Kfs . | Opq . | Acc . | Framework . |
---|---|---|---|---|---|---|---|---|---|
1006A | Metatexite | 61.1 | 24.6 | 6.8 | 4.6 | 2.1 | 0.4 | 0.4 | |
5099 | Metatexite | 57.8 | 19.4 | 13 | 6.2 | 2.4 | 1 | tr | |
5186 | Metatexite | 48.1 | 27.5 | 10.1 | 14 | tr | 0.3 | tr | |
5223 | Metatexite | 61.8 | 18.9 | 5.7 | 7.8 | 4.6 | 1.1 | tr | |
5095 | Meladiatexite | 44 | 17 | 19 | 3 | 16 | 1 | tr | |
5079 | Meladiatexite | 50 | 24 | 13 | 8 | 5 | tr | tr | |
5020 | Mesodiatexite | 39.9 | 26.7 | 0.2 | 10.6 | 22.1 | tr | tr | Pl+Kfs |
5076 | Mesodiatexite | 42 | 40 | 0 | 8 | 10 | tr | tr | Pl |
5186A | Mesodiatexite | 44 | 29 | 0 | 13 | 14 | tr | tr | Pl+Kfs |
5224 | Mesodiatexite | 37 | 28 | 5 | 20 | 10 | tr | tr | Pl |
5110 | Mesodiatexite | 50 | 25 | 0 | 20 | 5 | tr | tr | Pl |
57 | Leucodiatexite | 25 | 30 | 0 | 5 | 40 | tr | tr | Kfs+Pl |
5074 | Leucodiatexite | 47 | 41 | 0 | 10 | 2 | tr | tr | Pl |
5105A | Leucodiatexite | 27 | 25 | 0 | 4 | 44 | tr | tr | Kfs+Pl |
5109A8 | Leucodiatexite | 27.3 | 34.1 | 1 | 1.6 | 35.5 | 0.5 | tr | Kfs+Pl |
Sample . | Rock type . | Pl . | Qz . | Opx . | Bt . | Kfs . | Opq . | Acc . | Framework . |
---|---|---|---|---|---|---|---|---|---|
1006A | Metatexite | 61.1 | 24.6 | 6.8 | 4.6 | 2.1 | 0.4 | 0.4 | |
5099 | Metatexite | 57.8 | 19.4 | 13 | 6.2 | 2.4 | 1 | tr | |
5186 | Metatexite | 48.1 | 27.5 | 10.1 | 14 | tr | 0.3 | tr | |
5223 | Metatexite | 61.8 | 18.9 | 5.7 | 7.8 | 4.6 | 1.1 | tr | |
5095 | Meladiatexite | 44 | 17 | 19 | 3 | 16 | 1 | tr | |
5079 | Meladiatexite | 50 | 24 | 13 | 8 | 5 | tr | tr | |
5020 | Mesodiatexite | 39.9 | 26.7 | 0.2 | 10.6 | 22.1 | tr | tr | Pl+Kfs |
5076 | Mesodiatexite | 42 | 40 | 0 | 8 | 10 | tr | tr | Pl |
5186A | Mesodiatexite | 44 | 29 | 0 | 13 | 14 | tr | tr | Pl+Kfs |
5224 | Mesodiatexite | 37 | 28 | 5 | 20 | 10 | tr | tr | Pl |
5110 | Mesodiatexite | 50 | 25 | 0 | 20 | 5 | tr | tr | Pl |
57 | Leucodiatexite | 25 | 30 | 0 | 5 | 40 | tr | tr | Kfs+Pl |
5074 | Leucodiatexite | 47 | 41 | 0 | 10 | 2 | tr | tr | Pl |
5105A | Leucodiatexite | 27 | 25 | 0 | 4 | 44 | tr | tr | Kfs+Pl |
5109A8 | Leucodiatexite | 27.3 | 34.1 | 1 | 1.6 | 35.5 | 0.5 | tr | Kfs+Pl |
Sample . | Rock type . | Pl . | Qz . | Opx . | Bt . | Kfs . | Opq . | Acc . | Framework . |
---|---|---|---|---|---|---|---|---|---|
1006A | Metatexite | 61.1 | 24.6 | 6.8 | 4.6 | 2.1 | 0.4 | 0.4 | |
5099 | Metatexite | 57.8 | 19.4 | 13 | 6.2 | 2.4 | 1 | tr | |
5186 | Metatexite | 48.1 | 27.5 | 10.1 | 14 | tr | 0.3 | tr | |
5223 | Metatexite | 61.8 | 18.9 | 5.7 | 7.8 | 4.6 | 1.1 | tr | |
5095 | Meladiatexite | 44 | 17 | 19 | 3 | 16 | 1 | tr | |
5079 | Meladiatexite | 50 | 24 | 13 | 8 | 5 | tr | tr | |
5020 | Mesodiatexite | 39.9 | 26.7 | 0.2 | 10.6 | 22.1 | tr | tr | Pl+Kfs |
5076 | Mesodiatexite | 42 | 40 | 0 | 8 | 10 | tr | tr | Pl |
5186A | Mesodiatexite | 44 | 29 | 0 | 13 | 14 | tr | tr | Pl+Kfs |
5224 | Mesodiatexite | 37 | 28 | 5 | 20 | 10 | tr | tr | Pl |
5110 | Mesodiatexite | 50 | 25 | 0 | 20 | 5 | tr | tr | Pl |
57 | Leucodiatexite | 25 | 30 | 0 | 5 | 40 | tr | tr | Kfs+Pl |
5074 | Leucodiatexite | 47 | 41 | 0 | 10 | 2 | tr | tr | Pl |
5105A | Leucodiatexite | 27 | 25 | 0 | 4 | 44 | tr | tr | Kfs+Pl |
5109A8 | Leucodiatexite | 27.3 | 34.1 | 1 | 1.6 | 35.5 | 0.5 | tr | Kfs+Pl |
Sample . | Rock type . | Pl . | Qz . | Opx . | Bt . | Kfs . | Opq . | Acc . | Framework . |
---|---|---|---|---|---|---|---|---|---|
1006A | Metatexite | 61.1 | 24.6 | 6.8 | 4.6 | 2.1 | 0.4 | 0.4 | |
5099 | Metatexite | 57.8 | 19.4 | 13 | 6.2 | 2.4 | 1 | tr | |
5186 | Metatexite | 48.1 | 27.5 | 10.1 | 14 | tr | 0.3 | tr | |
5223 | Metatexite | 61.8 | 18.9 | 5.7 | 7.8 | 4.6 | 1.1 | tr | |
5095 | Meladiatexite | 44 | 17 | 19 | 3 | 16 | 1 | tr | |
5079 | Meladiatexite | 50 | 24 | 13 | 8 | 5 | tr | tr | |
5020 | Mesodiatexite | 39.9 | 26.7 | 0.2 | 10.6 | 22.1 | tr | tr | Pl+Kfs |
5076 | Mesodiatexite | 42 | 40 | 0 | 8 | 10 | tr | tr | Pl |
5186A | Mesodiatexite | 44 | 29 | 0 | 13 | 14 | tr | tr | Pl+Kfs |
5224 | Mesodiatexite | 37 | 28 | 5 | 20 | 10 | tr | tr | Pl |
5110 | Mesodiatexite | 50 | 25 | 0 | 20 | 5 | tr | tr | Pl |
57 | Leucodiatexite | 25 | 30 | 0 | 5 | 40 | tr | tr | Kfs+Pl |
5074 | Leucodiatexite | 47 | 41 | 0 | 10 | 2 | tr | tr | Pl |
5105A | Leucodiatexite | 27 | 25 | 0 | 4 | 44 | tr | tr | Kfs+Pl |
5109A8 | Leucodiatexite | 27.3 | 34.1 | 1 | 1.6 | 35.5 | 0.5 | tr | Kfs+Pl |
Diatexite migmatites
The diatexite migmatites are petrologically diverse (Fig. 2b), but share the following features: (1) grain size from 1 to 20 mm; (2) an alignment of biotite, tabular feldspar and biotite-rich schlieren which defines an S2 fabric; and (3) the presence of schollen of metatexite, vein quartz, mafic rocks and DIS. The schlieren and schollen indicate that S2 is a syn-anatectic, magmatic flow-foliation and hence that the diatexites were melt-rich rocks. The S2 deformation is pervasive across the Ashuanipi, but there is some variation in its orientation. Schollen are generally aligned in the S2 foliation and typically have asymmetric shapes that indicate a consistent magmatic shear-sense over large areas. In the southern Ashuanipi this is reverse motion to the southeast, and in the north it is reverse motion to the northwest, i.e. the migmatites were transported over adjacent plutonic and volcano-plutonic subprovinces. However, in places the S2 foliation is subvertical and developed in dextral strike-slip shear zones. Furthermore, local northeast-trending, dextral strike-slip shear zones in the diatexites contain an S3 foliation and meso-scale asymmetric F3 folds that have undeformed leucosomes in the axial planes and hinges. Thus, many diatexites occupy, or became, crustal-scale melt-bearing shear zones during late Archean regional dextral transpression. Local, late leucosome-filled normal-sense shear bands occur in the diatexites and suggest that exhumation of the orogen began with melt still present.
The common mineral assemblage in diatexites is Pl + Qz + Kfs + Bt + Opx + Ilm/Mag; Grt is rare (Table 1); zircon and apatite are common accessory phases, but monazite and rutile also occur. The main compositional variation evident in the field is the proportion of biotite + orthopyroxene, and thus diatexites are divided into melanocratic (>20% Opx + Bt), mesocratic (10 to 20%) and leucocratic (<10%) varieties. A few diatexites contain minor Cpx and/or Hbl.
Melanocratic diatexites have the smallest grain size (1 to 5 mm), and some contain vestiges of S0, S1 and leucosomes, like the metatexites. However, most diatexites are mesocratic with a grain size between 5 and 15 mm. Some mesocratic diatexites are comparatively uniform (Figs 2b, 3a, b), but others are very heterogeneous (Fig. 3c, d) with centimetre-scale variations in modal mineralogy, grain size, and the proportions of schollen and schlieren they contain. Leucocratic diatexites have the largest grain size (5 to 20 mm), fewest schollen, or schlieren, and typically occur as metre-scale diffuse patches and layers, or vein-like bodies in the mesocratic diatexites.
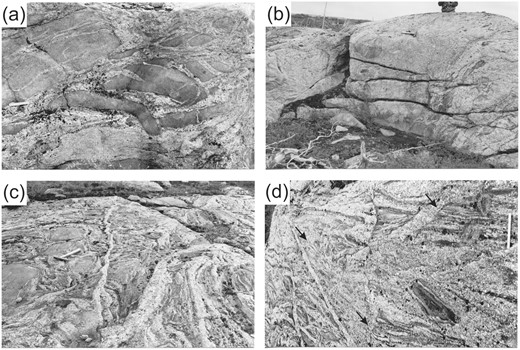
Field aspect of the diatexite migmatites. The upper images show the sequence across an abrupt metatexite to diatexite transition. (a) At the contact, the metatexite host is disrupted into tabular schollen by the injection of anatectic leucogranite. The schollen become smaller and rounded within a short distance into the diatexite body (upper right of the image). (b) The interior of the diatexite ∼100m from the contact; note fewer and more rounded schollen of metatexite, scattered biotite-rich schlieren and that the diatexite is mesocratic and uniform. Images (c) and (d) show a more gradational transition from metatexite to heterogeneous diatexite. (c) Metatexite (to the left) progressively injected by leucogranitic veins; the youngest are the most leucocratic and K-feldspar-rich and widen into the diatexite, suggesting that they root there. (d) Complex internal structure in the diatexite ∼10m from the contact. Cross-cutting, thin layers and units of diatexite each with a different grain size, proportion of ferromagnesian minerals, metatexite schollen, schlieren and internal flow structures, all cut by thin, leucocratic veins rich in K-feldspar. Arrows indicate some truncations of the compositional banding in the diatexite. Ruler in (a) is 15 cm long, and that in (d) is 30 cm.
Leucosome in the diatexite
In this study, centimetre-sized leucocratic patches and veins are considered to be locally derived if they have diffuse, or partly diffuse, borders with the host diatexite, and are called leucosome. They are distinguished from leucocratic diatexites by the absence of schollen and schlieren. Orthopyroxene is a minor phase in some leucosomes and, typically, is replaced by biotite.
Diatexites that have a strong S2 magmatic foliation locally contain leucosomes oriented in shear bands that are oriented between 30o and 60o to S2, between S2 foliation boudins, or along S2 (i.e. stromatic leucosomes). Locally the leucosomes form a weak net pattern, which indicates that melt was segregated from the diatexite as it crystallised during non-coaxial deformation.
Anatectic leucogranites in the migmatites
Bodies of leucogranite intrude the metatexite and diatexite migmatites throughout the Ashuanipi Subprovince. Most are thin (<30 cm) subhorizontal (<35o) sheets with sharp contacts that extend ∼100 m; however, the largest reported by Thériault & Chevé (2001) is >100 km long. The sheets of leucogranite are generally parallel to the main fabric in the host metatexite and diatexite (Fig. 2c, d). Some sheets consist of multiple layers, each with a different microstructure, and are the aggregate of multiple injections of small batches of melt (Morfin et al., 2014; Hall & Kisters, 2016). Small stocks and plutons of leucogranite occur mostly in the diatexite.
Modal analyses and mesonorms indicate that the leucogranites range from monzogranite to granodiorite with trace amounts of biotite and orthopyroxene. Most are of medium grain size, but some are coarse (>5 cm). Thériault & Chevé (2001) argue that the age (2647± 2Ma; Parent, 1998) and field relations indicate that the leucogranites and diatexite migmatites are genetically related.
Field relationship between metatexite and diatexite
The morphology of partially melted rocks in many contact aureoles changes from metatexite to diatexite as grade increases. Perhaps, once a temperature-dependent threshold fraction of melt is attained, bulk flow begins and primary diatexites develop (Sawyer, 2008). However, in the regional Ashuanipi Subprovince, metatexite and diatexite occur together, which suggests a different relationship.
The transition from metatexite to diatexite typically occurs over 0.5 to 10 m. In the narrow transition shown in Fig. 3, metatexite is injected by leucocratic veins, and the detached pieces become schollen in the diatexite. The schollen become smaller by fracture and rounded by erosion in the diatexite (Fig. 3a right). Hence this diatexite results from an injected felsic melt becoming contaminated by its metatexite wall rocks; it is a secondary diatexite (Sawyer, 2008; Vernon & Clarke, 2008). About 150 m from its margin, the diatexite is mesocratic and rather uniform with few schollen and schlieren (Fig. 3b). Its size (>1 km wide) and internal uniformity suggests that this diatexite formed where a large volume of leucocratic magma was able to inject the metatexite, and, since that is melt-depleted residuum, the melt became contaminated by residuum and peritectic phases (i.e. orthopyroxene).
Elsewhere, diatexite is more heterogeneous. Fig. 3c shows metatexite (left) intruded by a progression of veins; the oldest is mesocratic and plagioclase-rich with biotite schlieren and elongate schollen detached from the walls, whereas the youngest are K-feldspar-rich, highly leucocratic and root in the diatexite. Moreover, this diatexite is internally complex (Fig. 3d). Diatexite with a pronounced magmatic foliation and many schlieren is cut by narrow bands of more uniform diatexite that are truncated by a wider band of diatexite containing diffuse patches and wispy melanocratic and leucocratic parts. Overall, one flow-banded schlieric diatexite may truncate another slightly different one. This pattern suggests that the diatexite body formed in a tectonically active environment from many small batches of magma in which the more mobile parts flowed and truncated the less mobile; that is, it was deformed whilst still partially molten. Naturally, the mobile fraction decreased as crystallisation progressed, and the last increments form the narrowest veins of K-feldspar-rich leucocratic diatexite that roots in mesocratic diatexite and intrudes the metatexite wallrock.
MICROSTRUCTURE IN THE MIGMATITES
Metatexite migmatites
The metatexites have a granoblastic-polygonal microstructure and a grain size of ∼0.7mm, but scattered clusters of larger (∼1 mm), polygonal plagioclase (An29-32) grains occur within this microstructure (see figures in Sawyer 2001, 2008; Guernina & Sawyer 2003). Metatexites also contain domains in which small (>0.1 mm) rounded grains of biotite, plagioclase and quartz are separated by thin films of K-feldspar, or occur within large interstitial grains of K-feldspar. In places, orthopyroxene has straight crystal faces against the interstitial K-feldspar. K-feldspar in these rocks occurs only as thin films, or as irregularly shaped, cuspate interstitial grains that occupy former melt-filled pores. Sawyer (2001) considered these microstructures to be evidence for the melting reaction Pl + Bt + Qz = Opx + Melt + Ilm/Mag, and therefore the films of K-feldspar represent a crystallisation product of the melt.
Melanocratic diatexite
Most melanocratic diatexites have the assemblage Pl + Qz + Opx + Bt ± Kfs ± Grt and two microstructural components. The first comprises large (5 to 10 mm) domains of polygonal (∼1 mm) plagioclase (An29-32) in which small rounded grains of biotite and quartz together with rare, cuspate crystals of K-feldspar occur between the plagioclase. This part of the microstructure is identical to that in the metatexites. The second comprises narrow veins containing large (2–3 mm), equant, subhedral crystals of plagioclase (An23) with interstitial quartz and minor K-feldspar and indicates crystallisation from a granitic melt. These domains enclose fragments with metatexite-type microstructure. Some melanocratic diatexites have another microstructural component comprising thin (5 to 10 mm) discordant veins in which K-feldspar and plagioclase form a framework with interstitial quartz and K-feldspar. The microstructural features suggest that melanocratic diatexites formed where metatexite was injected by anatectic melt that left behind veins of Pl + Qz; in some cases, injection by a second melt left Pl + Kfs + Qz veins.
Mesocratic and leucocratic diatexites
The principal microstructure in these diatexites is a framework of large, subhedral crystals of feldspar and orthopyroxene. The interstices are filled by large anhedral crystals of quartz and/or K-feldspar, or by aggregates of small crystals. The frameworks range from open to densely packed. In open frameworks (Fig. 4a), feldspar crystals touch at the corners, whereas in dense frameworks, crystals touch along their faces (Fig. 4b) and consequently had less pore space. The dense frameworks are interpreted to result from tectonic compaction of a crystal mush, and so imply the segregation of melt. Most diatexites have a framework structure between loose- and densely packed varieties.
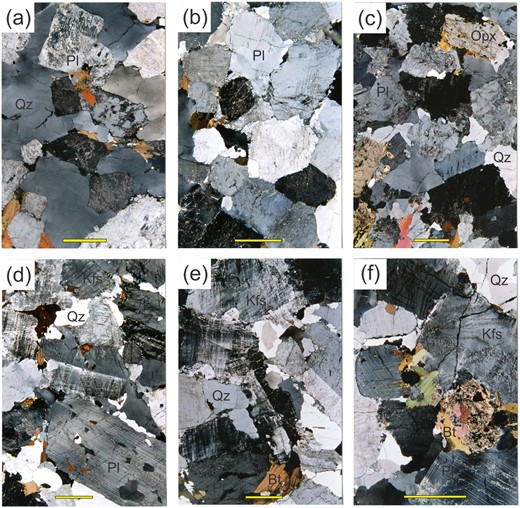
Microstructures in the diatexite migmatites. (a) Loose-packed framework of subhedral to euhedral plagioclase with interstitial quartz (with minor undulose extinction) and biotite. (b) Densely packed plagioclase framework from a tonalitic diatexite with interstitial K-feldspar + quartz + biotite. (c) Framework from a typical tonalitic mesocratic diatexite comprising subhedral to euhedral plagioclase and orthopyroxene with interstitial quartz; note that the orthopyroxene shows little alteration to biotite. (d) Framework in a granodioritic diatexite comprising subhedral crystals of plagioclase + K-feldspar with interstitial quartz. (e) Framework dominated by K-feldspar but also with plagioclase from a leucocratic diatexite; quartz is interstitial. (f) Subhedral crystal of orthopyroxene largely replaced by biotite in a K-feldspar + plagioclase framework. Scale bar represents 2 mm.
Feldspar frameworks are primary microstructures formed as granitic magmas crystallise (Byron et al., 1994); those in the Ashuanipi diatexites were modified by deformation whilst melt was still present. There are three types of framework in the Ashuanipi diatexites and leucogranites. The most common is dominated by plagioclase and is found in most mesocratic and some leucocratic diatexites. These frameworks comprise plagioclase (An23-25) and a few modal % large euhedral crystals of orthopyroxene (Fig. 4c); the interstices are filled by quartz, plagioclase and K-feldspar. Some leucogranites also have plagioclase-dominated frameworks. The second type, found in some mesocratic diatexites and leucogranites, has both plagioclase (rim ∼An22) and K-feldspar forming the framework (Fig. 4d) and interstices filled by anhedral K-feldspar, plagioclase and quartz. Rarely, K-feldspar coats the interstices and quartz fills the interior, but the inverse also occurs. In the third type, found in some leucodiatexites, leucosomes and leucogranites, the framework is dominated by large subhedral crystals of K-feldspar (Fig. 4e); quartz, K-feldspar and sodic plagioclase (An15) fill the interstices. In a few leucodiatexites, the framework consists of K-feldspar and a sodic plagioclase (∼An15). Some K-feldspar-dominated frameworks are densely packed and have been compacted. Framework plagioclase is consistently more sodic (An23-25) than residual plagioclase (An29-32) in the metatexites and has weak normal zoning, consistent with crystallisation from melt. Furthermore, some framework plagioclase contains rounded, calcic (∼An32) patches of similar size (∼1 mm) and composition to the polygonal plagioclase in metatexites. Thus, magmatic plagioclase may have nucleated on crystals of residual plagioclase entrained in the diatexite magma (Carvalho et al., 2016).
The order of crystallisation for the anatectic melt deduced from the frameworks is orthopyroxene, plagioclase, quartz, then K-feldspar, but in some, K-feldspar crystallised before quartz. Framework-forming plagioclase becomes more sodic through the three types, consistent with fractionating melt. Orthopyroxene is a minor constituent in all types of framework but is progressively replaced by biotite + plagioclase symplectite, which recrystallises to large grains of biotite (Fig. 4f), as modal K-feldspar increases. Thus, after K-feldspar began to crystallise, orthopyroxene reacted with the remaining H2O-rich melt to produce biotite. However, orthopyroxene in metatexites just centimetres away remains unaltered.
WHOLE-ROCK COMPOSITIONS
Analytical methods
The samples of diatexite and leucogranite collected for this study were between 1 and 3 kg, depending upon grain size. None of the diatexites contained schollen of metatextite, although some contained schlieren. The major oxides and trace elements Ba, Nb, Rb, Sr, Y and Zr were determined on 58 diatexites and 21 leucogranites from the Ashuanipi Subprovince by standard XRF technique using fused glass discs and pressed powder pellets; Cr, Cs, Hf, Sc, Th, U and the rare earth elements (REE) were determined using instrumental neutron activation analysis (INAA) at the analytical laboratories of the Ministère des Ressources Naturelles du Québec (MRNQ). For the 22 diatexites and 10 leucogranites in which the Tb and Lu contents were close to, or below, the detection limit of the MRNQ laboratories, the REE, NaO2, FeO, Ba, Co, Cr, Cs, Hf, Rb, Sc, Ta, Th and U were determined by INAA at the Université du Québec à Chicoutimi (UQAC) following irradiation in the SLOWPOKE II reactor at École Polytechnique in Montreal; Bédard & Barnes (1990) give details of the INAA procedure at UQAC. The major (XRF) and trace element (ICPMS) contents of two metatexite samples were determined at Geoscience Laboratories (GeoLabs) Ontario Geological Survey, Sudbury. A representative set of the analytical results is given in Table 2, and the complete data set in the Supplementary Data Electronic Appendix 1 (all the Electronic Appendices may be downloaded from http://www.petrology.oupjournals.org/.).
Representative whole-rock compositions of Ashuanipi metatexites, diatexites and leucogranites
Sample . | EA8 . | 5095A . | 5186 . | 2047 . | 5020 . | 5224 . | 57 . | 5074 . | 5105A . | 17119 . | 17131 . | 17145 . |
---|---|---|---|---|---|---|---|---|---|---|---|---|
Rock . | Meta . | Mela . | Meso . | Meso . | Meso . | Meso . | Leuco . | Leuco . | Leuco . | Leuco . | Leuco . | Leuco . |
type . | textite . | diatexite . | diatexite . | diatexite . | diatexite . | diatexite . | diatexite . | diatexite . | diatexite . | granite . | granite . | granite . |
SiO2wt% | 67.11 | 66.2 | 69 | 67.8 | 67.3 | 69.5 | 75.1 | 73.1 | 73.3 | 68.3 | 72.2 | 74.1 |
TiO2 | 0.51 | 0.46 | 0.22 | 0.59 | 0.47 | 0.35 | 0.11 | 0.27 | 0.14 | 0.28 | 0.22 | 0.07 |
Al2O3 | 16.07 | 14.6 | 15.4 | 14.7 | 15 | 15.7 | 13.3 | 13.8 | 14 | 15.1 | 14.5 | 14.0 |
FeO | 4.11 | 5.26 | 3.24 | 4.28 | 3.55 | 3.46 | 1.07 | 2.28 | 1.08 | 2.99 | 1.59 | 0.68 |
MnO | 0.02 | 0.06 | 0.04 | 0.04 | 0.02 | 0.02 | 0.01 | 0.01 | 0.01 | 0.04 | 0.01 | 0.01 |
MgO | 2.29 | 2.86 | 1.75 | 1.2 | 1.66 | 1.5 | 0.5 | 0.96 | 0.56 | 1.56 | 0.54 | 0.14 |
CaO | 2.36 | 2.49 | 2.6 | 2.09 | 1.9 | 1.96 | 0.93 | 2.69 | 0.83 | 2.5 | 1.46 | 0.59 |
Na2O | 4.3 | 3.72 | 3.61 | 3.55 | 3.44 | 3.34 | 2.59 | 4.06 | 2.98 | 4.24 | 3.9 | 3.17 |
K2O | 2.24 | 3.09 | 3.04 | 3.85 | 4.32 | 3.89 | 6.3 | 1.21 | 6.77 | 3.87 | 4.6 | 6.45 |
P2O5 | 0.05 | 0.11 | 0.2 | 0.24 | 0.06 | 0.07 | 0.06 | 0.05 | 0.11 | 0.17 | 0.1 | 0.04 |
LOI | 0.57 | 0.44 | 0.43 | 0.62 | 0.88 | 0.79 | 0.33 | 0.88 | 0.43 | 0.62 | 0.3 | 0.43 |
Cr ppm | 206 | 150 | 88 | 46 | 106 | 94 | 21 | 89 | 20 | 46 | 15 | 5 |
Co | 20 | 21 | 10 | 8 | 12 | 10 | 3 | 9 | 5 | 8 | 3 | 2 |
Sc | 8 | 15 | 8 | 7 | 9 | 7 | 2 | 5 | 3 | 4 | 1 | bdl |
Rb | 79 | 67 | 90 | 126 | 192 | 139 | 147 | 39 | 160 | 103 | 117 | 151 |
Cs | 0.99 | 0.5 | 0.75 | 0.58 | 1.16 | 0.61 | 2.46 | bdl | 0.5 | 1.12 | 0.83 | 0.88 |
Ba | 558 | 930 | 950 | 640 | 1000 | 910 | 1800 | 260 | 930 | 1000 | 1100 | 1400 |
Sr | 459 | 449 | 430 | 328 | 402 | 391 | 506 | 402 | 213 | 605 | 461 | 427 |
Ga | 17 | 17 | 17 | 20 | 17 | 18 | 13 | 16 | 13 | 17 | 16 | 13 |
Ta | 0.31 | bdl | 0.11 | 0.19 | 0.22 | 0.17 | 0.06 | bdl | bdl | 0.15 | 0.07 | 0.04 |
Nb | 5.7 | bdl | 3 | 10 | 4 | 5 | 2 | 2 | bdl | 2 | 2 | 2 |
Hf | 6.2 | 4.4 | 5.64 | 12.63 | 6.93 | 3.11 | 0.71 | 15.17 | 7.2 | 2.96 | 3.1 | 2.39 |
Zr | 238 | 143 | 180 | 432 | 196 | 117 | 39 | 443 | 206 | 110 | 105 | 81 |
Y | 6 | 11 | 19 | 15 | 3 | 3 | 3 | 4 | 7 | 7 | 3 | 3 |
Th | 5.33 | 7 | 17.05 | 25.7 | 59.22 | 11.4 | 0.95 | 6.56 | 13 | 3.27 | 7.57 | 8.24 |
U | 0.78 | bdl | 0.81 | 0.71 | 0.83 | 0.39 | 0.21 | 0.99 | 1.1 | 0.76 | 0.38 | 0.31 |
La | 31.4 | 32 | 45.05 | 72.36 | 92.42 | 30.21 | 7.1 | 21.91 | 27 | 26.5 | 32.41 | 24.29 |
Ce | 58.5 | 56 | 83.81 | 144.9 | 181.4 | 51.9 | 12 | 40.19 | 51 | 51.49 | 58.77 | 38.78 |
Pr | 6.16 | na | na | na | 19.87 | na | 1.31 | na | na | na | na | na |
Nd | 21.1 | 26 | 35.53 | 61.78 | 69.15 | 19.97 | 5.01 | 13.8 | 22 | 20.95 | 19.99 | 14.38 |
Sm | 3.07 | 4.1 | 5.44 | 9.06 | 8.72 | 2.57 | 0.93 | 2.05 | 3.2 | 3.05 | 2.27 | 1.6 |
Eu | 1.14 | 1.2 | 1.05 | 1.45 | 1.18 | 0.93 | 0.83 | 1.05 | 0.4 | 1.17 | 0.88 | 0.67 |
Gd | 1.85 | na | na | na | 5.2 | na | 0.72 | na | na | na | na | na |
Tb | 0.22 | 0.5 | 0.71 | 0.78 | 0.53 | 0.24 | 0.1 | 0.19 | 0.2 | 0.28 | 0.19 | 0.09 |
Dy | 1.2 | na | na | na | 1.7 | na | 0.6 | na | na | na | na | na |
Ho | 0.22 | 0.6 | na | 0.68 | 0.26 | na | 0.12 | 0.18 | na | na | na | na |
Er | 0.62 | na | na | na | 0.53 | na | 0.31 | na | na | na | na | na |
Tm | 0.09 | na | na | na | 0.07 | na | 0.04 | na | na | na | na | na |
Yb | 0.62 | 1.5 | 1.39 | 1.04 | 0.46 | 0.26 | 0.23 | 0.68 | 0.7 | 0.64 | 0.26 | 0.13 |
Lu | 0.11 | 0.2 | 0.21 | 0.14 | 0.08 | 0.04 | 0.03 | 0.11 | bdl | 0.09 | 0.03 | 0.02 |
Sample . | EA8 . | 5095A . | 5186 . | 2047 . | 5020 . | 5224 . | 57 . | 5074 . | 5105A . | 17119 . | 17131 . | 17145 . |
---|---|---|---|---|---|---|---|---|---|---|---|---|
Rock . | Meta . | Mela . | Meso . | Meso . | Meso . | Meso . | Leuco . | Leuco . | Leuco . | Leuco . | Leuco . | Leuco . |
type . | textite . | diatexite . | diatexite . | diatexite . | diatexite . | diatexite . | diatexite . | diatexite . | diatexite . | granite . | granite . | granite . |
SiO2wt% | 67.11 | 66.2 | 69 | 67.8 | 67.3 | 69.5 | 75.1 | 73.1 | 73.3 | 68.3 | 72.2 | 74.1 |
TiO2 | 0.51 | 0.46 | 0.22 | 0.59 | 0.47 | 0.35 | 0.11 | 0.27 | 0.14 | 0.28 | 0.22 | 0.07 |
Al2O3 | 16.07 | 14.6 | 15.4 | 14.7 | 15 | 15.7 | 13.3 | 13.8 | 14 | 15.1 | 14.5 | 14.0 |
FeO | 4.11 | 5.26 | 3.24 | 4.28 | 3.55 | 3.46 | 1.07 | 2.28 | 1.08 | 2.99 | 1.59 | 0.68 |
MnO | 0.02 | 0.06 | 0.04 | 0.04 | 0.02 | 0.02 | 0.01 | 0.01 | 0.01 | 0.04 | 0.01 | 0.01 |
MgO | 2.29 | 2.86 | 1.75 | 1.2 | 1.66 | 1.5 | 0.5 | 0.96 | 0.56 | 1.56 | 0.54 | 0.14 |
CaO | 2.36 | 2.49 | 2.6 | 2.09 | 1.9 | 1.96 | 0.93 | 2.69 | 0.83 | 2.5 | 1.46 | 0.59 |
Na2O | 4.3 | 3.72 | 3.61 | 3.55 | 3.44 | 3.34 | 2.59 | 4.06 | 2.98 | 4.24 | 3.9 | 3.17 |
K2O | 2.24 | 3.09 | 3.04 | 3.85 | 4.32 | 3.89 | 6.3 | 1.21 | 6.77 | 3.87 | 4.6 | 6.45 |
P2O5 | 0.05 | 0.11 | 0.2 | 0.24 | 0.06 | 0.07 | 0.06 | 0.05 | 0.11 | 0.17 | 0.1 | 0.04 |
LOI | 0.57 | 0.44 | 0.43 | 0.62 | 0.88 | 0.79 | 0.33 | 0.88 | 0.43 | 0.62 | 0.3 | 0.43 |
Cr ppm | 206 | 150 | 88 | 46 | 106 | 94 | 21 | 89 | 20 | 46 | 15 | 5 |
Co | 20 | 21 | 10 | 8 | 12 | 10 | 3 | 9 | 5 | 8 | 3 | 2 |
Sc | 8 | 15 | 8 | 7 | 9 | 7 | 2 | 5 | 3 | 4 | 1 | bdl |
Rb | 79 | 67 | 90 | 126 | 192 | 139 | 147 | 39 | 160 | 103 | 117 | 151 |
Cs | 0.99 | 0.5 | 0.75 | 0.58 | 1.16 | 0.61 | 2.46 | bdl | 0.5 | 1.12 | 0.83 | 0.88 |
Ba | 558 | 930 | 950 | 640 | 1000 | 910 | 1800 | 260 | 930 | 1000 | 1100 | 1400 |
Sr | 459 | 449 | 430 | 328 | 402 | 391 | 506 | 402 | 213 | 605 | 461 | 427 |
Ga | 17 | 17 | 17 | 20 | 17 | 18 | 13 | 16 | 13 | 17 | 16 | 13 |
Ta | 0.31 | bdl | 0.11 | 0.19 | 0.22 | 0.17 | 0.06 | bdl | bdl | 0.15 | 0.07 | 0.04 |
Nb | 5.7 | bdl | 3 | 10 | 4 | 5 | 2 | 2 | bdl | 2 | 2 | 2 |
Hf | 6.2 | 4.4 | 5.64 | 12.63 | 6.93 | 3.11 | 0.71 | 15.17 | 7.2 | 2.96 | 3.1 | 2.39 |
Zr | 238 | 143 | 180 | 432 | 196 | 117 | 39 | 443 | 206 | 110 | 105 | 81 |
Y | 6 | 11 | 19 | 15 | 3 | 3 | 3 | 4 | 7 | 7 | 3 | 3 |
Th | 5.33 | 7 | 17.05 | 25.7 | 59.22 | 11.4 | 0.95 | 6.56 | 13 | 3.27 | 7.57 | 8.24 |
U | 0.78 | bdl | 0.81 | 0.71 | 0.83 | 0.39 | 0.21 | 0.99 | 1.1 | 0.76 | 0.38 | 0.31 |
La | 31.4 | 32 | 45.05 | 72.36 | 92.42 | 30.21 | 7.1 | 21.91 | 27 | 26.5 | 32.41 | 24.29 |
Ce | 58.5 | 56 | 83.81 | 144.9 | 181.4 | 51.9 | 12 | 40.19 | 51 | 51.49 | 58.77 | 38.78 |
Pr | 6.16 | na | na | na | 19.87 | na | 1.31 | na | na | na | na | na |
Nd | 21.1 | 26 | 35.53 | 61.78 | 69.15 | 19.97 | 5.01 | 13.8 | 22 | 20.95 | 19.99 | 14.38 |
Sm | 3.07 | 4.1 | 5.44 | 9.06 | 8.72 | 2.57 | 0.93 | 2.05 | 3.2 | 3.05 | 2.27 | 1.6 |
Eu | 1.14 | 1.2 | 1.05 | 1.45 | 1.18 | 0.93 | 0.83 | 1.05 | 0.4 | 1.17 | 0.88 | 0.67 |
Gd | 1.85 | na | na | na | 5.2 | na | 0.72 | na | na | na | na | na |
Tb | 0.22 | 0.5 | 0.71 | 0.78 | 0.53 | 0.24 | 0.1 | 0.19 | 0.2 | 0.28 | 0.19 | 0.09 |
Dy | 1.2 | na | na | na | 1.7 | na | 0.6 | na | na | na | na | na |
Ho | 0.22 | 0.6 | na | 0.68 | 0.26 | na | 0.12 | 0.18 | na | na | na | na |
Er | 0.62 | na | na | na | 0.53 | na | 0.31 | na | na | na | na | na |
Tm | 0.09 | na | na | na | 0.07 | na | 0.04 | na | na | na | na | na |
Yb | 0.62 | 1.5 | 1.39 | 1.04 | 0.46 | 0.26 | 0.23 | 0.68 | 0.7 | 0.64 | 0.26 | 0.13 |
Lu | 0.11 | 0.2 | 0.21 | 0.14 | 0.08 | 0.04 | 0.03 | 0.11 | bdl | 0.09 | 0.03 | 0.02 |
bdl, below detection limit; na, not determined.
Representative whole-rock compositions of Ashuanipi metatexites, diatexites and leucogranites
Sample . | EA8 . | 5095A . | 5186 . | 2047 . | 5020 . | 5224 . | 57 . | 5074 . | 5105A . | 17119 . | 17131 . | 17145 . |
---|---|---|---|---|---|---|---|---|---|---|---|---|
Rock . | Meta . | Mela . | Meso . | Meso . | Meso . | Meso . | Leuco . | Leuco . | Leuco . | Leuco . | Leuco . | Leuco . |
type . | textite . | diatexite . | diatexite . | diatexite . | diatexite . | diatexite . | diatexite . | diatexite . | diatexite . | granite . | granite . | granite . |
SiO2wt% | 67.11 | 66.2 | 69 | 67.8 | 67.3 | 69.5 | 75.1 | 73.1 | 73.3 | 68.3 | 72.2 | 74.1 |
TiO2 | 0.51 | 0.46 | 0.22 | 0.59 | 0.47 | 0.35 | 0.11 | 0.27 | 0.14 | 0.28 | 0.22 | 0.07 |
Al2O3 | 16.07 | 14.6 | 15.4 | 14.7 | 15 | 15.7 | 13.3 | 13.8 | 14 | 15.1 | 14.5 | 14.0 |
FeO | 4.11 | 5.26 | 3.24 | 4.28 | 3.55 | 3.46 | 1.07 | 2.28 | 1.08 | 2.99 | 1.59 | 0.68 |
MnO | 0.02 | 0.06 | 0.04 | 0.04 | 0.02 | 0.02 | 0.01 | 0.01 | 0.01 | 0.04 | 0.01 | 0.01 |
MgO | 2.29 | 2.86 | 1.75 | 1.2 | 1.66 | 1.5 | 0.5 | 0.96 | 0.56 | 1.56 | 0.54 | 0.14 |
CaO | 2.36 | 2.49 | 2.6 | 2.09 | 1.9 | 1.96 | 0.93 | 2.69 | 0.83 | 2.5 | 1.46 | 0.59 |
Na2O | 4.3 | 3.72 | 3.61 | 3.55 | 3.44 | 3.34 | 2.59 | 4.06 | 2.98 | 4.24 | 3.9 | 3.17 |
K2O | 2.24 | 3.09 | 3.04 | 3.85 | 4.32 | 3.89 | 6.3 | 1.21 | 6.77 | 3.87 | 4.6 | 6.45 |
P2O5 | 0.05 | 0.11 | 0.2 | 0.24 | 0.06 | 0.07 | 0.06 | 0.05 | 0.11 | 0.17 | 0.1 | 0.04 |
LOI | 0.57 | 0.44 | 0.43 | 0.62 | 0.88 | 0.79 | 0.33 | 0.88 | 0.43 | 0.62 | 0.3 | 0.43 |
Cr ppm | 206 | 150 | 88 | 46 | 106 | 94 | 21 | 89 | 20 | 46 | 15 | 5 |
Co | 20 | 21 | 10 | 8 | 12 | 10 | 3 | 9 | 5 | 8 | 3 | 2 |
Sc | 8 | 15 | 8 | 7 | 9 | 7 | 2 | 5 | 3 | 4 | 1 | bdl |
Rb | 79 | 67 | 90 | 126 | 192 | 139 | 147 | 39 | 160 | 103 | 117 | 151 |
Cs | 0.99 | 0.5 | 0.75 | 0.58 | 1.16 | 0.61 | 2.46 | bdl | 0.5 | 1.12 | 0.83 | 0.88 |
Ba | 558 | 930 | 950 | 640 | 1000 | 910 | 1800 | 260 | 930 | 1000 | 1100 | 1400 |
Sr | 459 | 449 | 430 | 328 | 402 | 391 | 506 | 402 | 213 | 605 | 461 | 427 |
Ga | 17 | 17 | 17 | 20 | 17 | 18 | 13 | 16 | 13 | 17 | 16 | 13 |
Ta | 0.31 | bdl | 0.11 | 0.19 | 0.22 | 0.17 | 0.06 | bdl | bdl | 0.15 | 0.07 | 0.04 |
Nb | 5.7 | bdl | 3 | 10 | 4 | 5 | 2 | 2 | bdl | 2 | 2 | 2 |
Hf | 6.2 | 4.4 | 5.64 | 12.63 | 6.93 | 3.11 | 0.71 | 15.17 | 7.2 | 2.96 | 3.1 | 2.39 |
Zr | 238 | 143 | 180 | 432 | 196 | 117 | 39 | 443 | 206 | 110 | 105 | 81 |
Y | 6 | 11 | 19 | 15 | 3 | 3 | 3 | 4 | 7 | 7 | 3 | 3 |
Th | 5.33 | 7 | 17.05 | 25.7 | 59.22 | 11.4 | 0.95 | 6.56 | 13 | 3.27 | 7.57 | 8.24 |
U | 0.78 | bdl | 0.81 | 0.71 | 0.83 | 0.39 | 0.21 | 0.99 | 1.1 | 0.76 | 0.38 | 0.31 |
La | 31.4 | 32 | 45.05 | 72.36 | 92.42 | 30.21 | 7.1 | 21.91 | 27 | 26.5 | 32.41 | 24.29 |
Ce | 58.5 | 56 | 83.81 | 144.9 | 181.4 | 51.9 | 12 | 40.19 | 51 | 51.49 | 58.77 | 38.78 |
Pr | 6.16 | na | na | na | 19.87 | na | 1.31 | na | na | na | na | na |
Nd | 21.1 | 26 | 35.53 | 61.78 | 69.15 | 19.97 | 5.01 | 13.8 | 22 | 20.95 | 19.99 | 14.38 |
Sm | 3.07 | 4.1 | 5.44 | 9.06 | 8.72 | 2.57 | 0.93 | 2.05 | 3.2 | 3.05 | 2.27 | 1.6 |
Eu | 1.14 | 1.2 | 1.05 | 1.45 | 1.18 | 0.93 | 0.83 | 1.05 | 0.4 | 1.17 | 0.88 | 0.67 |
Gd | 1.85 | na | na | na | 5.2 | na | 0.72 | na | na | na | na | na |
Tb | 0.22 | 0.5 | 0.71 | 0.78 | 0.53 | 0.24 | 0.1 | 0.19 | 0.2 | 0.28 | 0.19 | 0.09 |
Dy | 1.2 | na | na | na | 1.7 | na | 0.6 | na | na | na | na | na |
Ho | 0.22 | 0.6 | na | 0.68 | 0.26 | na | 0.12 | 0.18 | na | na | na | na |
Er | 0.62 | na | na | na | 0.53 | na | 0.31 | na | na | na | na | na |
Tm | 0.09 | na | na | na | 0.07 | na | 0.04 | na | na | na | na | na |
Yb | 0.62 | 1.5 | 1.39 | 1.04 | 0.46 | 0.26 | 0.23 | 0.68 | 0.7 | 0.64 | 0.26 | 0.13 |
Lu | 0.11 | 0.2 | 0.21 | 0.14 | 0.08 | 0.04 | 0.03 | 0.11 | bdl | 0.09 | 0.03 | 0.02 |
Sample . | EA8 . | 5095A . | 5186 . | 2047 . | 5020 . | 5224 . | 57 . | 5074 . | 5105A . | 17119 . | 17131 . | 17145 . |
---|---|---|---|---|---|---|---|---|---|---|---|---|
Rock . | Meta . | Mela . | Meso . | Meso . | Meso . | Meso . | Leuco . | Leuco . | Leuco . | Leuco . | Leuco . | Leuco . |
type . | textite . | diatexite . | diatexite . | diatexite . | diatexite . | diatexite . | diatexite . | diatexite . | diatexite . | granite . | granite . | granite . |
SiO2wt% | 67.11 | 66.2 | 69 | 67.8 | 67.3 | 69.5 | 75.1 | 73.1 | 73.3 | 68.3 | 72.2 | 74.1 |
TiO2 | 0.51 | 0.46 | 0.22 | 0.59 | 0.47 | 0.35 | 0.11 | 0.27 | 0.14 | 0.28 | 0.22 | 0.07 |
Al2O3 | 16.07 | 14.6 | 15.4 | 14.7 | 15 | 15.7 | 13.3 | 13.8 | 14 | 15.1 | 14.5 | 14.0 |
FeO | 4.11 | 5.26 | 3.24 | 4.28 | 3.55 | 3.46 | 1.07 | 2.28 | 1.08 | 2.99 | 1.59 | 0.68 |
MnO | 0.02 | 0.06 | 0.04 | 0.04 | 0.02 | 0.02 | 0.01 | 0.01 | 0.01 | 0.04 | 0.01 | 0.01 |
MgO | 2.29 | 2.86 | 1.75 | 1.2 | 1.66 | 1.5 | 0.5 | 0.96 | 0.56 | 1.56 | 0.54 | 0.14 |
CaO | 2.36 | 2.49 | 2.6 | 2.09 | 1.9 | 1.96 | 0.93 | 2.69 | 0.83 | 2.5 | 1.46 | 0.59 |
Na2O | 4.3 | 3.72 | 3.61 | 3.55 | 3.44 | 3.34 | 2.59 | 4.06 | 2.98 | 4.24 | 3.9 | 3.17 |
K2O | 2.24 | 3.09 | 3.04 | 3.85 | 4.32 | 3.89 | 6.3 | 1.21 | 6.77 | 3.87 | 4.6 | 6.45 |
P2O5 | 0.05 | 0.11 | 0.2 | 0.24 | 0.06 | 0.07 | 0.06 | 0.05 | 0.11 | 0.17 | 0.1 | 0.04 |
LOI | 0.57 | 0.44 | 0.43 | 0.62 | 0.88 | 0.79 | 0.33 | 0.88 | 0.43 | 0.62 | 0.3 | 0.43 |
Cr ppm | 206 | 150 | 88 | 46 | 106 | 94 | 21 | 89 | 20 | 46 | 15 | 5 |
Co | 20 | 21 | 10 | 8 | 12 | 10 | 3 | 9 | 5 | 8 | 3 | 2 |
Sc | 8 | 15 | 8 | 7 | 9 | 7 | 2 | 5 | 3 | 4 | 1 | bdl |
Rb | 79 | 67 | 90 | 126 | 192 | 139 | 147 | 39 | 160 | 103 | 117 | 151 |
Cs | 0.99 | 0.5 | 0.75 | 0.58 | 1.16 | 0.61 | 2.46 | bdl | 0.5 | 1.12 | 0.83 | 0.88 |
Ba | 558 | 930 | 950 | 640 | 1000 | 910 | 1800 | 260 | 930 | 1000 | 1100 | 1400 |
Sr | 459 | 449 | 430 | 328 | 402 | 391 | 506 | 402 | 213 | 605 | 461 | 427 |
Ga | 17 | 17 | 17 | 20 | 17 | 18 | 13 | 16 | 13 | 17 | 16 | 13 |
Ta | 0.31 | bdl | 0.11 | 0.19 | 0.22 | 0.17 | 0.06 | bdl | bdl | 0.15 | 0.07 | 0.04 |
Nb | 5.7 | bdl | 3 | 10 | 4 | 5 | 2 | 2 | bdl | 2 | 2 | 2 |
Hf | 6.2 | 4.4 | 5.64 | 12.63 | 6.93 | 3.11 | 0.71 | 15.17 | 7.2 | 2.96 | 3.1 | 2.39 |
Zr | 238 | 143 | 180 | 432 | 196 | 117 | 39 | 443 | 206 | 110 | 105 | 81 |
Y | 6 | 11 | 19 | 15 | 3 | 3 | 3 | 4 | 7 | 7 | 3 | 3 |
Th | 5.33 | 7 | 17.05 | 25.7 | 59.22 | 11.4 | 0.95 | 6.56 | 13 | 3.27 | 7.57 | 8.24 |
U | 0.78 | bdl | 0.81 | 0.71 | 0.83 | 0.39 | 0.21 | 0.99 | 1.1 | 0.76 | 0.38 | 0.31 |
La | 31.4 | 32 | 45.05 | 72.36 | 92.42 | 30.21 | 7.1 | 21.91 | 27 | 26.5 | 32.41 | 24.29 |
Ce | 58.5 | 56 | 83.81 | 144.9 | 181.4 | 51.9 | 12 | 40.19 | 51 | 51.49 | 58.77 | 38.78 |
Pr | 6.16 | na | na | na | 19.87 | na | 1.31 | na | na | na | na | na |
Nd | 21.1 | 26 | 35.53 | 61.78 | 69.15 | 19.97 | 5.01 | 13.8 | 22 | 20.95 | 19.99 | 14.38 |
Sm | 3.07 | 4.1 | 5.44 | 9.06 | 8.72 | 2.57 | 0.93 | 2.05 | 3.2 | 3.05 | 2.27 | 1.6 |
Eu | 1.14 | 1.2 | 1.05 | 1.45 | 1.18 | 0.93 | 0.83 | 1.05 | 0.4 | 1.17 | 0.88 | 0.67 |
Gd | 1.85 | na | na | na | 5.2 | na | 0.72 | na | na | na | na | na |
Tb | 0.22 | 0.5 | 0.71 | 0.78 | 0.53 | 0.24 | 0.1 | 0.19 | 0.2 | 0.28 | 0.19 | 0.09 |
Dy | 1.2 | na | na | na | 1.7 | na | 0.6 | na | na | na | na | na |
Ho | 0.22 | 0.6 | na | 0.68 | 0.26 | na | 0.12 | 0.18 | na | na | na | na |
Er | 0.62 | na | na | na | 0.53 | na | 0.31 | na | na | na | na | na |
Tm | 0.09 | na | na | na | 0.07 | na | 0.04 | na | na | na | na | na |
Yb | 0.62 | 1.5 | 1.39 | 1.04 | 0.46 | 0.26 | 0.23 | 0.68 | 0.7 | 0.64 | 0.26 | 0.13 |
Lu | 0.11 | 0.2 | 0.21 | 0.14 | 0.08 | 0.04 | 0.03 | 0.11 | bdl | 0.09 | 0.03 | 0.02 |
bdl, below detection limit; na, not determined.
Starting-point compositions
Field relations show that diatexite formed where felsic melt injected and accumulated in metatexite. Therefore, the compositions of metatexite and anatectic melt are starting points for investigating the petrogenesis of the diatexites. The compositional field of metatexites (Fig. 5) is defined by 30 samples, namely 28 from Guernina & Sawyer (2003) plus the new analyses; the average composition of the metagreywacke metatexites (AMT) is shown on the plots. The field for anatectic melts in Fig. 5 is defined by quenched glasses from melting experiments conducted at P–T conditions appropriate to the Ashuanipi, in which Opx + Melt were reaction products (SBG, Patiño-Douce & Beard, 1995; CEV, Montel &Vielzeuf, 1997). The average of six CEV glasses (CEVG 8/851, 5/883, 8/942 and CEVP 5/883, 8/875, 8/919), but with CaO/(CaO+Na2O), adjusted from 0.17 to 0.34 so as to be compatible with a more calcic Archaean metagreywacke source that yields melt (like sample 1017) which crystallises ∼An20 plagioclase; the resulting model composition is shown as AMM. The major oxide compositions for AMT and AMM are given in Table 3. The compositions of the metatexites and experimental glasses plotted in the figures are given in Supplementary Data Electronic Appendices 2 and 3.
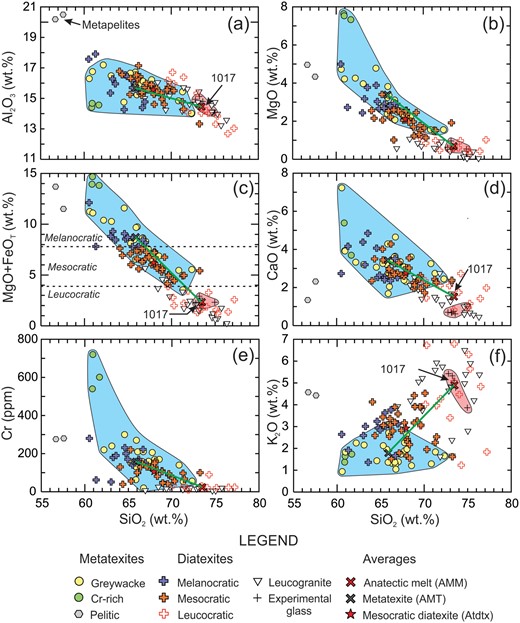
Harker variation diagrams for metatexites, diatexites and leucogranites from the Ashuanipi Subprovince. (a) Al2O3 vs SiO2, (b) MgO vs SiO2, (c) (MgO+FeOT) vs SiO2, (d) , CaO vs SiO2, (e) Cr vs SiO2 and (f) K2O vs SiO2. The field of greywacke-derived metatexites (i.e. residual compositions) is shown in blue, and that of experimental melts from metagreywacke bulk compositions is in pink.
Sample . | AMM . | AMT . | Atdtx . | Mix . | Amdtx . | Rmtx . |
---|---|---|---|---|---|---|
n . | 6 . | 28 . | 32 . | . | 10 . | 3 . |
SiO2 wt% | 73.47 | 65.89 | 67.95 | 68.62 | 64.53 | 62.08 |
TiO2 | 0.16 | 0.51 | 0.49 | 0.38 | 0.58 | 0.63 |
Al2O3 | 14.45 | 15.67 | 15.56 | 15.21 | 15.85 | 16.81 |
FeO | 1.65 | 5.34 | 4.1 | 4.01 | 5.81 | 6.51 |
MnO | 0.05 | 0.07 | 0.05 | 0.06 | 0.06 | 0.09 |
MgO | 0.69 | 3.39 | 2.1 | 2.42 | 3.13 | 4.14 |
CaO | 1.52 | 3.42 | 2.75 | 2.74 | 2.95 | 3.49 |
Na2O | 3.01 | 3.8 | 3.95 | 3.52 | 3.76 | 4.11 |
K2O | 4.89 | 1.79 | 2.92 | 2.88 | 3.18 | 2.08 |
P2O5 | 0.11 | 0.12 | 0.14 | 0.12 | 0.14 | 0.07 |
Cr ppm | 158 | 105 | 233 | |||
Co | 19 | 13.4 | 26.1 | |||
Sc | 15 | 10.1 |
Sample . | AMM . | AMT . | Atdtx . | Mix . | Amdtx . | Rmtx . |
---|---|---|---|---|---|---|
n . | 6 . | 28 . | 32 . | . | 10 . | 3 . |
SiO2 wt% | 73.47 | 65.89 | 67.95 | 68.62 | 64.53 | 62.08 |
TiO2 | 0.16 | 0.51 | 0.49 | 0.38 | 0.58 | 0.63 |
Al2O3 | 14.45 | 15.67 | 15.56 | 15.21 | 15.85 | 16.81 |
FeO | 1.65 | 5.34 | 4.1 | 4.01 | 5.81 | 6.51 |
MnO | 0.05 | 0.07 | 0.05 | 0.06 | 0.06 | 0.09 |
MgO | 0.69 | 3.39 | 2.1 | 2.42 | 3.13 | 4.14 |
CaO | 1.52 | 3.42 | 2.75 | 2.74 | 2.95 | 3.49 |
Na2O | 3.01 | 3.8 | 3.95 | 3.52 | 3.76 | 4.11 |
K2O | 4.89 | 1.79 | 2.92 | 2.88 | 3.18 | 2.08 |
P2O5 | 0.11 | 0.12 | 0.14 | 0.12 | 0.14 | 0.07 |
Cr ppm | 158 | 105 | 233 | |||
Co | 19 | 13.4 | 26.1 | |||
Sc | 15 | 10.1 |
AMM, anatectic melt; AMT, metatexite; Atdtx, typical diatexite; Amdtx, melanocratic diatexite; Rmtx, most residual metatexite.
Sample . | AMM . | AMT . | Atdtx . | Mix . | Amdtx . | Rmtx . |
---|---|---|---|---|---|---|
n . | 6 . | 28 . | 32 . | . | 10 . | 3 . |
SiO2 wt% | 73.47 | 65.89 | 67.95 | 68.62 | 64.53 | 62.08 |
TiO2 | 0.16 | 0.51 | 0.49 | 0.38 | 0.58 | 0.63 |
Al2O3 | 14.45 | 15.67 | 15.56 | 15.21 | 15.85 | 16.81 |
FeO | 1.65 | 5.34 | 4.1 | 4.01 | 5.81 | 6.51 |
MnO | 0.05 | 0.07 | 0.05 | 0.06 | 0.06 | 0.09 |
MgO | 0.69 | 3.39 | 2.1 | 2.42 | 3.13 | 4.14 |
CaO | 1.52 | 3.42 | 2.75 | 2.74 | 2.95 | 3.49 |
Na2O | 3.01 | 3.8 | 3.95 | 3.52 | 3.76 | 4.11 |
K2O | 4.89 | 1.79 | 2.92 | 2.88 | 3.18 | 2.08 |
P2O5 | 0.11 | 0.12 | 0.14 | 0.12 | 0.14 | 0.07 |
Cr ppm | 158 | 105 | 233 | |||
Co | 19 | 13.4 | 26.1 | |||
Sc | 15 | 10.1 |
Sample . | AMM . | AMT . | Atdtx . | Mix . | Amdtx . | Rmtx . |
---|---|---|---|---|---|---|
n . | 6 . | 28 . | 32 . | . | 10 . | 3 . |
SiO2 wt% | 73.47 | 65.89 | 67.95 | 68.62 | 64.53 | 62.08 |
TiO2 | 0.16 | 0.51 | 0.49 | 0.38 | 0.58 | 0.63 |
Al2O3 | 14.45 | 15.67 | 15.56 | 15.21 | 15.85 | 16.81 |
FeO | 1.65 | 5.34 | 4.1 | 4.01 | 5.81 | 6.51 |
MnO | 0.05 | 0.07 | 0.05 | 0.06 | 0.06 | 0.09 |
MgO | 0.69 | 3.39 | 2.1 | 2.42 | 3.13 | 4.14 |
CaO | 1.52 | 3.42 | 2.75 | 2.74 | 2.95 | 3.49 |
Na2O | 3.01 | 3.8 | 3.95 | 3.52 | 3.76 | 4.11 |
K2O | 4.89 | 1.79 | 2.92 | 2.88 | 3.18 | 2.08 |
P2O5 | 0.11 | 0.12 | 0.14 | 0.12 | 0.14 | 0.07 |
Cr ppm | 158 | 105 | 233 | |||
Co | 19 | 13.4 | 26.1 | |||
Sc | 15 | 10.1 |
AMM, anatectic melt; AMT, metatexite; Atdtx, typical diatexite; Amdtx, melanocratic diatexite; Rmtx, most residual metatexite.
Composition of diatexite and leucogranite relative to anatectic melt and metatexite
Metatexites define a field on the variation diagrams that extends from 60 to 72 wt % SiO2 and have high Al2O3, MgO, (MgO+FeOT), CaO, Cr (Fig. 5a– e), TiO2, FeOT, Co and Sc contents that correlate negatively with SiO2. The abundances of K2O (Fig. 5f) and Na2O are low and correlate poorly with SiO2. The pelitic metatexites have notably lower SiO2, CaO (and Na2O) but higher Al2O3 and K2O contents. Three metatexites have high MgO and Cr (average 628 ppm) contents, even higher than rocks from the DIS (Percival et al., 2003) at equivalent SiO2, and are probably metagreywackes with unusually high proportions of ultramafic detritus.
The field of experimental glass compositions extends from 72 to 76 wt % SiO2 (Fig. 5). The contents of TiO2, FeOT, MgO, CaO and K2O are all low and correlate negatively with SiO2. Diatexites form a large field, with most samples between the metatexite and anatectic melt fields (Fig. 5). However, some leucocratic diatexites have higher SiO2 and K2O, but lower MgO and (MgO+FeOT) contents than the anatectic melts; these have compositions more evolved than the anatectic melt. The Al2O3, FeOT, MgO, CaO and Cr contents of diatexites correlate negatively with SiO2, but K2O correlates positively (Fig. 5). Fig. 5c shows that the field divisions of melanocratic, mesocratic and leucocratic diatexite correspond to ∼7.8 and 3.9 wt % (MgO+FeOT) respectively.
Diatexites span four fields on a quartz–alkali-feldspar–plagioclase (QAP) mesonorm plot (Fig. 6); 25 in the tonalite field, 25 in the granodiorite field and seven in the monzogranite field. The sample in the quartz diorite field is hereafter grouped with those of tonalitic composition. Thirteen diatexites (10 tonalitic and three granodioritic) are melanocratic, 32 are mesocratic (15 tonalitic, 16 granodioritic, one monzogranitic) and 13 are leucocratic (one tonalitic, six granodioritic, six monzogranitic). Thus, the most common (32/58 samples), or ‘typical’ diatexite in the Ashuanipi is mesocratic and of granodioritic or tonalitic composition; the average of the ‘typical’ diatexites (Atdtx in Table 3) is shown (red star) on the composition plots.
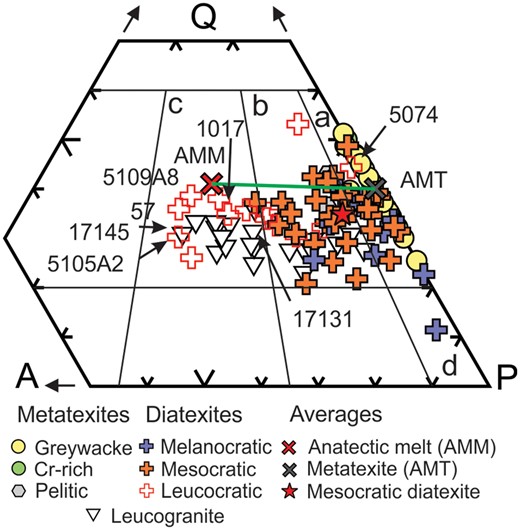
Quartz-alkali-feldspar-plagioclase (QAP) mesonorm plot. Metatexite migmatites plot along the Q-P join and into the tonalite field, diatexite migmatites extend from the tonalite to the monzogranite field, and leucogranites occupy the granodiorite and monzogranite fields. The average mesocratic diatexite lies to the right of the granodiorite field, and most mesocratic and melanocratic diatexites lie on the P side of the AMM–AMT tie-line. Lettered fields are: a, tonalite; b, granodiorite; c, monzogranite; and d, quartz diorite.
The greywacke-derived experimental glass compositions and the adjusted model melt composition (AMM) lie in the monzogranite field, whereas the anatectic leucogranites lie in the monzogranite and granodiorite fields below AMM. Leucodiatexite sample 1017 is closest to AMM, and from its major element composition may represent an initial Ashuanipi anatectic melt. Metatexites lie along, or close to, the Q–P join.
Composition of feldspars in the diatexites
Because plagioclase is the only CaO-bearing major phase in most Ashuanipi diatexites and metatexites, variation in the CaO and Na2O contents reflects changes in the abundance, or composition, of plagioclase. The Na2O/(Na2O+CaO) ratio reflects the albite content of the plagioclase, and the whole-rock (Na2O+CaO) content reflects the weight fraction of plagioclase. On a plot (Fig. 7) of Na2O vs (Na2O+CaO), the diatexites and leucogranites lie between ∼An10 and An30; AAM corresponds to ∼33% plagioclase of composition An23, and leucodiatexite 1017 to ∼34% plagioclase of composition An23.
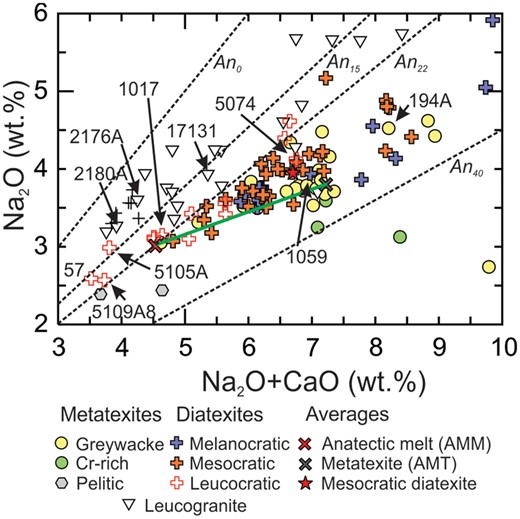
Plots of Na2O vs (Na2O+CaO) to show the range in plagioclase content and composition in the anatectic rocks from the Ashuanipi Subprovince.
Metatexites define a field (Fig. 7) in which the abundance and composition of plagioclase changes from left (∼35% plagioclase An23) to right (>60% plagioclase An35). This is consistent with an increasing degree of partial melting and extent of melt loss from the metatexites.
Most mesocratic diatexites define a trend between plagioclase An23 and An28, and contain from ∼40 to ∼53% plagioclase (Fig. 7), consistent with the modal analyses (Table 1). Leucocratic diatexites contain the lowest abundance (∼27%) of, and least calcic (An13 to An20), plagioclase. Conversely, melanocratic diatexites have the most calcic (up to An37) and highest abundances (>60%) of plagioclase.
Partial melting of greywacke produces K2O-rich melt and a plagioclase + ferromagnesian mineral-rich residuum (Montel & Vielzeuf, 1997). Consequently, residuum and melt are well separated on plots of (Na2O+CaO) vs K2O (Fig. 8a) and (MgO+FeOT) vs K2O (Fig. 8b). Because K2O is partitioned between biotite and K-feldspar, the high (MgO+FeOT) content of the most melanocratic diatexites means that biotite accounts for all their K2O. However, biotite accounts for <0.9 wt % K2O in leucocratic diatexites; they contain 30 to 50% K-feldspar. The most potassic diatexites (57, 5105A, 5109A8 and 3311A) have the highest Na2O/(Na2O+CaO) ratios (Figs 7, 8a) and represent the most evolved, K-feldspar-rich compositions.
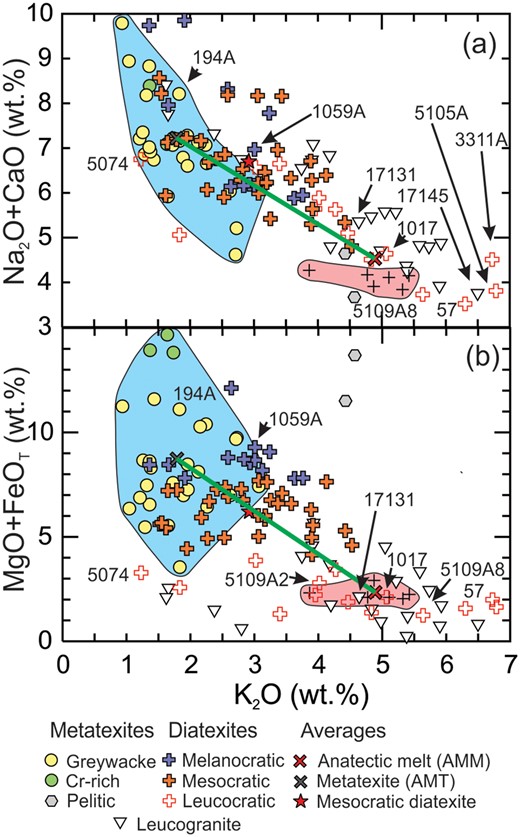
Variation diagrams showing components that partition into the residuum: (a) (Na2O+CaO) and (b) (MgO + FeOT) vs K2O, a component that partitions into anatectic melt. Note that some leucodiatexites and leucogranites contain more K2O than the field of anatectic melts. In (a) the AMM plots just above the pink field because its composition has been adjusted to be compatible with Archean metagreywacke (see text).
High-field-strength elements
The contents of Zr, Th, La, Y and Yb in the metatexites increase slightly as SiO2 decreases, indicating weak enrichment in the residuum (Fig. 9). Most diatexites follow this pattern but with more scatter; this may reflect the metatexite component in the diatexites. However, relative to the metatexites, 10 to 15% of diatexites are enriched in these trace elements between 67 and 74 wt % SiO2. Maximum values are between 70 and 71 wt % SiO2 and 4 or 5 times higher than the ‘background’ for Zr and Th, and 2 to 3 times higher for La, Y and Yb. This peak at ∼70 wt % SiO2 strongly resembles the saturation of felsic magmas with accessory phases during differentiation (Hoskin et al., 2000), and may reflect process occurring as the anatectic melt component in the diatexites crystallised. Alternatively, the enrichment could be the result of entrained phases from the residuum, but in that case the reason why the peak values should be at ∼70 wt % SiO2 is not evident.
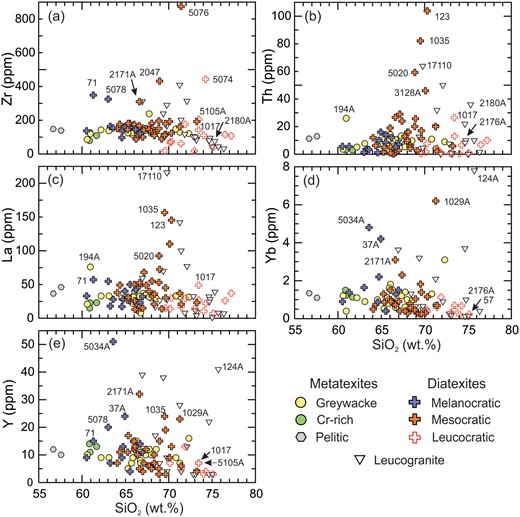
Harker diagrams for trace elements: (a) Zr, (b) Th, (c) La, (d) Yb and (e) Y. The metatexites show a slight decrease in the content of these trace elements as SiO2 increases. Diatexites show the same pattern, except that some mesocratic and leucocratic diatexites show a higher abundance of these trace elements between 68 and 74 wt % SiO2. Some leucogranites also show enrichment of these trace elements over the same SiO2 range.
Rare earth elements
The metatexites show a narrow range of REE contents (Fig. 10a) and have moderate slopes (average LaN/YbN = 19.5). Most have small positive Eu anomalies (Eu* 1.1 to 1.57), but some have smooth REE patterns (Eu* 1 ± 0.1), and one, a negative Eu anomaly. The diatexites (Figs 10b–d) display a greater range in REE content and steeper patterns (average LaN/YbN = 40.6) overall. About half have positive Eu anomalies (Eu* up to 3.4), ∼28% have negative Eu anomalies, and ∼20% have smooth patterns. Melanocratic diatexites show the least and leucodiatexites show the greatest range in REE content, shape of pattern and type of Eu anomaly. The range of EuN for the diatexites is much less than the range in the other REEs (e.g. La or Yb). Hence, variation in the levels of the REE patterns in the diatexites is due principally to the abundance and relative proportion of REE-bearing accessory phases, not to differences in the content of feldspar, biotite or orthopyroxene, phases that are not particularly rich in REE. This is consistent with crystal fractionation in the diatexites. Although leucodiatexite 1017 is similar to AMM in terms of major components (e.g. Fig. 6), its ΣREE content, negative Eu anomaly and steep pattern (LaN/YbN = 68.5) suggests derivation from a somewhat fractionated melt.

Chondrite-normalised rare earth element patterns (normalising factors from Sun & McDonough, 1989) for Ashuanipi anatectic rocks: (a) metatexites, (b) melanocratic diatexites, (c) mesocratic diatexites, (d) leucodiatexites and (e) leucogranites.
Compositional characteristics of the Ashuanipi leucogranites
The leucogranites are monzogranitic and granodioritic rocks that define a broad field (Figs 5, 6 and 8) that overlaps the anatectic melt field defined by the experimental glasses and broadly coincides with the leucodiatexites. There are two subgroups. One has high (5 to 6.5 wt %) K2O and low (Na2O+CaO) contents corresponding to ∼45 modal % K-feldspar and ∼30% plagioclase. The other has lower K2O (<3 wt %) but high Na2O, and thus a higher content (>50%) of sodic plagioclase (An8-20).
The highest Zr, Th, La, Y and Yb contents in the leucogranites (Fig. 9) are between 67 and 73 wt % SiO2 and decrease at higher SiO2, a pattern characteristic of saturation and subsequent fractionation of accessory phases from the melt. Leucogranites with high Th also have high La consistent with the presence of monazite, but a few samples have high levels of Zr, Th, La, Y and Yb consistent with monazite + zircon and/or xenotime. Epidote is not present.
DISCUSSION
The field evidence (e.g. Fig. 3a) indicates that Ashuanipi diatexites develop where anatectic melt intruded metatexite wall rock and mixed. A mass-balance calculation indicates that the average ‘typical’ diatexite (Atdtx) corresponds approximately to 0.64AMT + 0.36AMM (see ‘mix’ in Table 3). However, the following features all suggest that processes other than simple mixing played a part in the formation of the diatexites: (1) the displacement of many diatexites from the AMT–AMM tie-line (Fig. 5) and towards the A-P join in Fig. 6; (2) the extension of the diatexite field well beyond the melt field (Figs 5 and 8) to higher K2O; (3) the systematic change in plagioclase composition across the entire diatexite group (Fig. 7); (4) the increase in the content of high-field-strength elements at ∼70 wt % SiO2 (Fig. 9); and (5) the range of REE patterns (Fig. 10).
Bea et al. (2005) compared the natural compositional variants in a granite to the modelled fractionation of a starting melt and related progressively more evolved melts to episodic, deformation-driven tapping of fractionated melt as the pluton crystallised. This study uses a similar approach and compares the composition of the various diatexites and leucogranites with the modelled evolution of an initial anatectic melt to understand their development and to constrain the melt budget for the terrain.
Crystallisation of the anatectic melt
Assuming AMM as the initial anatectic melt composition, equilibrium crystallisation was modelled using the rhyolite melts (Asimov & Ghiorso 1998) and Pele 7.07 (Boudreau, 1999) software to determine the composition of the crystallised solid (CS1) and complementary fractionated liquid (FL1) from it. The results from Pele 7.07 are preferred because the composition of the crystallised phases it predicts are closer to the observed mineral compositions. Holtz et al. (2001) showed that a saturated granitic melt at ∼7 kbar and ∼900oC contains between 3 and 4 wt % H2O. As most initial anatectic melts are believed to be somewhat H2O-undersaturated, simulations were run with 1.5%, 2%, 2.5% and 3% H2O in the starting melt and at quartz-fayalite-magnetite (QFM), QFM-1 and Ni-NiO oxygen buffers. Results show more variation between different pressures and H2O contents than between the oxygen buffers, and therefore only the results for QFM simulations are used.
The first phase to crystallise in the simulations with 1.5%, 2% and 2.5% H2O at 7 kbar and 2%, 2.5% and 3% H2O at 8 kbar was orthopyroxene, followed by plagioclase, quartz, and K-feldspar. Typically, plagioclase appears after 1 to 2% crystallisation, quartz after 3 to 10%, and K-feldspar after 11 to 13%; biotite, magnetite and ilmenite appear at ∼40%. Simulated crystallisation of AMM (and of 1017) at 7 kbar with 3% H2O and at 6 kbar with 2, 2.5 and 3% H2O all produced K-feldspar before quartz. Thus, simulated crystallisation of AMM at 7 and 8 kbar starting with 1.5, 2 or 2.5% H2O match the order of crystallisation inferred from the majority of feldspar framework structures. Higher H2O content, lower pressure, or a different starting melt composition may account for the cases where K-feldspar crystallised before quartz.
The compositions of the 7-kbar fractionated liquids (FL1) and crystallised solids (CS1) from simulated crystallisation of AMM are shown on QAP plots in Fig. 11a (the compositions of the crystallised solids and fractionated liquids from AMM used for the modelling and for Figs 11 and 12 are given in Supplementary Data Electronic Appendix 4). The earliest-crystallised solids (CS1 in Fig. 11a) contain only orthopyroxene + plagioclase and so have low SiO2 and K2O, but high Al2O3, (MgO+FeOT) and (CaO+Na2O) contents; the composition of the crystallised solid moves progressively towards the P apex as crystallisation advances and more plagioclase than orthopyroxene crystallises. When quartz crystallises, the solid fraction gains SiO2 and moves back towards the Q apex (Fig. 11a), and when K-feldspar finally crystallises, the solid fraction contains K2O and moves into QAP space. The liquid fraction (FL1) is progressively richer in SiO2 (on an anhydrous basis), Na2O/CaO increases, but (MgO+FeOT) and Al2O3 decrease. Hence, diatexites, leucogranites and leucosomes with frameworks of plagioclase + orthopyroxene are largely the ‘melt products’ from an earlier, higher-temperature, stage of crystallisation than those with orthopyroxene + plagioclase + K-feldspar frameworks.
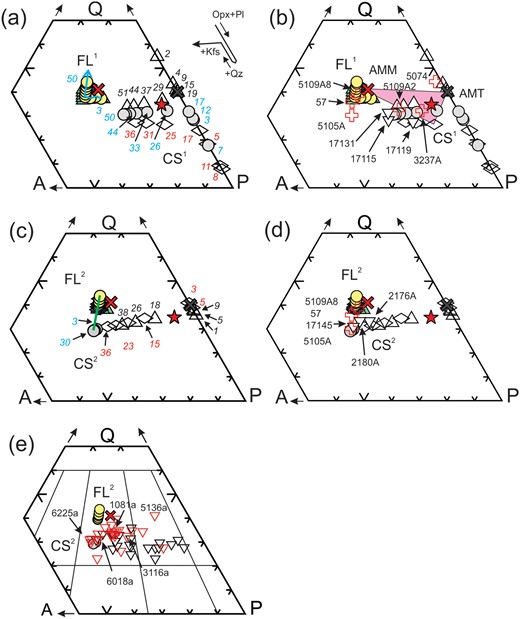
QAP mesonorm plots. (a) Results of the simulated crystallisation of AMM (red cross) at 7 kbar and QFM; triangles, circles and diamonds indicate AMM with 1.5, 2.0 and 2.5 wt % H2O respectively. Open and grey symbols show the compositions of crystallised solids (CS1), and colour-filled symbols show fractionated liquid (FL1) compositions. Numbers against the open symbols indicate the percentage crystallisation. (b) Selected diatexites and leucogranites compared with results from (a) showing that the average mesocratic diatexite (red star) is off the AMM–AMT tie-line, but in a three-component FL1-CS1-AMT composition space. Similarly, leucodiatexites 5074, 3237A, 5109A2 and leucogranites 17119, 17115 and 7131 can be modelled as various mixes of FL1, CS1 and AMT, but leucodiatexites 5109A8, 57 and 5105 cannot. (c) Results of the simulated crystallisation at 7 kbar and QFM of fractionated liquids (FL1) segregated as AMM crystallised; open diamonds, triangles and grey circles indicate compositions of crystallised solid (CS2) of a FL1 extracted after 7%, 12% and 26% crystallisation AMM with 2 wt % H2O respectively. Colour-filled symbols are the second-cycle fractionated liquid (FL2) compositions. Numbers against the open symbols are percentage crystallised. The green tie-line connects the second-cycle CS2 with its corresponding FL2 for 30% crystallisation. Panel (d) shows that leucodiatexites 5109A8, 57, 5105A and leucogranite 17145 all lie on the tie-line shown in (c). The location of leucogranites 2176A and 2180A indicate that they consist predominately of early crystallised phases from ∼30% crystallisation of a FL1 extracted from AMM when it was ∼12% crystallised. (e) Opinaca (red triangles, data from Morfin et al., 2014) and Ashuanipi leucogranites (black triangles) compared. Note the higher proportion of monozogranitic leucogranites in the Opinaca suite corresponding to the various CS2 compositions shown in (c) and the absence of leucogranite composition corresponding to fractionated liquid compositions FL1 or FL2.
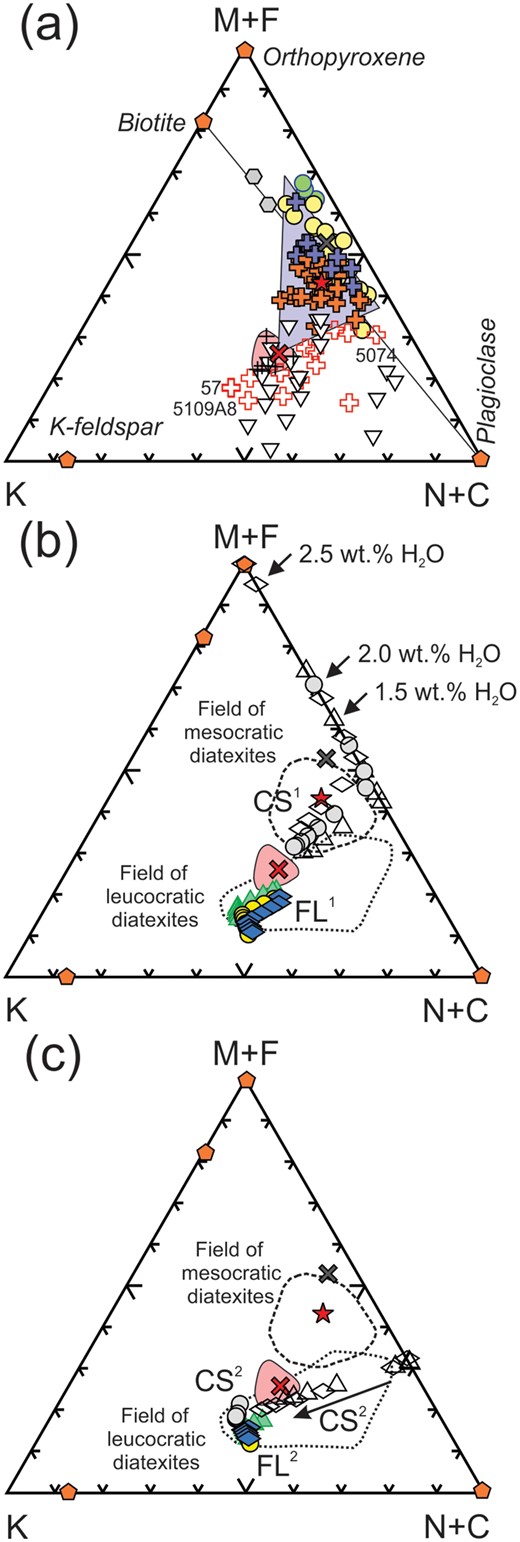
Molar K2O-(MgO+FeOT)-(Na2O+CaO) plots. (a) Ashuanipi anatectic rocks showing that metatexite migmatites lie in the Opx-Bt-Pl space, whereas the diatexites and leucogranites lie towards K-feldspar. The pink shaded field is for the experimental melts from metagreywacke (Montel & Vielzeuf, 1997). The blue triangle shows the space covered by mixtures of the full range of metatextite + AMM; the leucodiatexites and leucogranites lie outside such mixtures. (b) Plot showing the results of the simulated crystallisation of AMM (symbols as in Fig. 11a), that the field of mesocratic diatexites lies between the average metatexite and CS1 and FL1 and that mesocratic diatexites all have a large CS1 component. (c) The location of second-cycle crystallised solids (CS2) and fractionated liquids (FL2) obtained from simulated crystallisation of first cycle fractionated liquids (FL1); symbols as for Fig. 11c. Note that the leucodiatexites (e.g. 57, 5109A8) and some leucogranites correspond to CS2 compositions or CS2-FL2 mixtures.
Crystallisation of fractionated melts
Most, but not all, diatexites and leucogranites lie within the triangular composition space defined by AMT and the crystallised solids (CS1) and fractionated liquids (FL1) derived directly from AMM (Fig. 11b) and can, therefore, be modelled as mixtures of these three components, as will be shown in a subsequent section. Obvious exceptions are leucocratic diatexite samples 5109A8, 57 and 5105A and leucogranites 17145 and 2180A, which have K2O >5.6 wt %. To investigate these, the crystallisation of selected fractionated melts (FL1) from AMM was simulated to obtain compositions for the second-cycle crystallised solids (CS2) and fractionated liquids (FL2) that they generate. The results are shown in Fig. 11c, and the complete data set is given in the Supplementary Data Electronic Appendix 4. Crystallisation of first-cycle fractionated liquids (FL1) extracted before AMM reached ∼10% crystallisation have the same crystallisation order as AMM, and so their crystallised solids (CS2) are similar to CS1 (compare Figs 11a and c). In contrast, the CS2 obtained from FL1 extracted after AMM had undergone >20% crystallisation are significantly different. The crystallisation order is orthopyroxene, plagioclase, K-feldspar then quartz, but K-feldspar dominates. Consequently, these second-cycle crystallised solids (CS2) plot well into the monzogranite field (Fig. 11c), but FL2 compositions are rather similar to FL1.
The QAP plots do not show mafic components; these are shown in Fig. 12, a (MgO+FeOT)–K2O–(Na2O+CaO) molar plot. The melanocratic and mesocratic diatexites might be explained as mixtures of an initial anatectic melt and various metatexites (Fig. 12a), but the leucocratic diatexites and leucogranites clearly cannot because they have less (MgO+FeOT) than the initial anatectic melt. Fig. 12b shows that mesocratic diatexites are better fit as mixtures of first-cycle crystallised solids (CS1) and fractionated melts (FL1) from AMM plus metatexite, the latter controlling (MgO+FeOT) contents. However, many leucodiatexites, especially those with the highest K2O (e.g. 57, 5109A8) have even lower (MgO+FeOT) and lie close (Fig. 12c) to second-cycle crystallised solids CS2 and have no metatexite component. Fig. 12 illustrates the decrease in (MgO+FeOT) with increasing fractionation of the anatectic melt and is evidence that these evolved melts did not entrain wall-rock material.
The simulations indicate that the petrological diversity in the Ashuanipi diatexites reflects their progressive development. The plagioclase-dominated parts crystallised at higher temperatures from the initial anatectic melt, whereas the K-feldspar-rich parts formed at lower temperatures from more evolved melts.
Petrogenesis of the melanocratic and mesocratic diatexites
This section examines how the melanocratic and mesocratic diatexites formed and estimates the fraction of ‘melt product’, i.e. the fraction derived from anatectic melt, that they contain. Mass-balance calculations equate the composition of a target diatexite (or leucogranite) to combinations of metatexite country rock (typically AMT), AMM, or the crystallised solid (CS1) and fractionated liquid (FL1) obtained from its simulated crystallisation (Fig. 13). The starting point for the mass balance was determined from the position of the target rock relative to AMT and a crystallised solid and fractionated liquid pair, for example see the three-component mixing triangle for the average ‘typical’ diatexite in Fig. 11b, and then refined by iteration. The mass balance also yields how much melt was expelled in the diatexite-making process as a percentage of the initial amount of melt in the initial AMT+AMM mix.
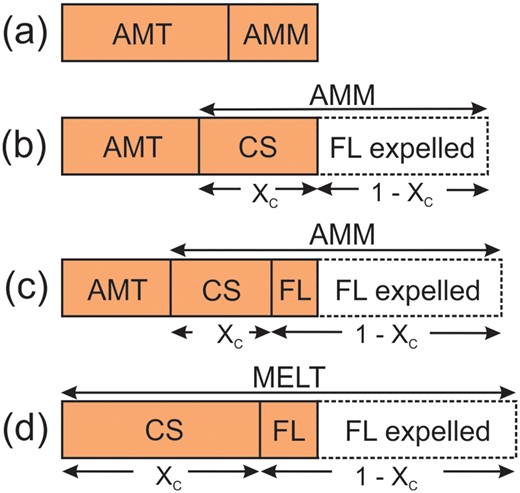
Summary of the mass-balance models used for diatexites and leucogranites. The shaded boxes represent the target rock with the components used to model it. Melanocratic and mesocratic diatexites are modelled as mixtures: (a) AMT + AMM, (b) AMT (or a specific metatexite as mentioned in the text) + crystallised solids (CS1), and (c) AMT + CS1 + fractionated liquid (FL1) from AMM. (d) Leucodiatexites and leucogranites derived from melt (AMM or a fractionated melt from it) are modelled as mixtures of crystallised solids + fractionated liquids, either CS1 + FL1 or CS2 + FL2 as appropriate. The dashed boxes represent the expelled portion of fractionated melt, the amount of which is calculated from the degree of crystallisation (XC) undergone by AMM, or the fractionated melt FL1 derived from it, given with the compositions of CS1, FL1, CS2 and FL1 in Supplementary Data Electronic Appendix 4.
Melanocratic diatexites
The majority (10 of 13) of melanocratic diatexites have higher (MgO+FeOT) contents than the average metatexite (AMT) therefore, the average metatexite composition is not a realistic starting composition for investigating how they formed. Fig. 5 shows that potential protoliths with higher (MgO+FeOT) include the three Cr-rich metagreywackes and two metapelites. The former can be discounted because their MgO and Cr (average 628 ppm) contents are too high to be a major component in any diatexite (average Cr 175 ppm), and the latter because their high Al2O3 contents result in mixes of pelite and AMM that are too aluminous (>19 wt % Al2O3).
An alternative is to use metatexites with the highest (MgO+FeOT) contents (i.e. samples 194A, 5099A, 7016A) as the country-rock component. A general mass balance for the average of 10 melanocratic diatexites (Amdtx, Table 3), using the average of the three metatexites (Rmtx, Table 3) with the highest (MgO+FeOT) and the products of simulated crystallisation of AMM with 2.5% H2O, is 0.72Rmtx + 0.08CS1 + 0.2FL1. In geological terms, this corresponds to an initial injection of 31% AMM into the metatexite country rocks; then, upon reaching 25% (this is XC for the composition of CS and FL used in the mass balance) crystallisation, 18% of the remaining melt was expelled. This process means that the average melanocratic diatexite contains 18% ‘melt product’, and that 14% of the amount of melt initially injected was segregated (i.e. Fig. 13c). This expelled melt is fractionated and could generate the K-feldspar-bearing veinlets in thin sections of some melanocratic diatexites. The injection of ∼31% melt to create the average melanocratic diatexites may be insufficient to completely disaggregate the host metatexite, enabling small domains with the metatexite microstructure to survive.
The ‘typical’ diatexite
The mass balance for the average ‘typical’ mesocratic diatexites (Atdtx) using only AMM and average metatexite (AMT) mentioned earlier underestimates their (CaO+Na2O) content (Fig. 8a). Therefore, this section examines mass balances using crystallised solid (CS1) and fractionated liquid (FL1) compositions from AMM to better fit the CaO and Na2O contents of the ‘typical’ diatexite. Because CS1 is dominated by plagioclase (for <40% crystallisation), CS1 must be a significant component in the ‘typical’ diatexite. The K2O in these diatexites comes either from the K-feldspar in the crystallised solid (Fig. 13b), or from a trapped fractionated melt (Fig. 13c).
Two end-member models made with the CS1 and FL1 from AMM with 2.5% H2O illustrate the counterbalance between crystallised solid and fractionated melts. The first prioritises the crystallised solids: Atdtx = 0.57AMT + 0.38CS1 + 0.05FL1. In physical terms, the diatexite magma begins as a mixture 0.352AMT + 0.648AMM. Then, when 36% (= XC) crystallised, most (92%) of the remaining melt is segregated. The final diatexite contains 43% ‘melt product’, which makes the plagioclase-dominated framework with some K-feldspar and ∼7.5% trapped melt. Of the original amount of intruded melt, 59% is segregated and crystallises elsewhere. The second mass balance favours the retention of fractionated melt to provide K2O and to dilute (MgO+FeOT), thus Atdtx = 0.56AMT + 0.24CS1 + 0.2FL1. In the outcrop, diatexite magma begins as a mixture of 0.365AMT + 0.635AMM, and then when 25% crystallised, 73% of the remaining melt was expelled. The resulting diatexite is 44% ‘melt product’, evident from its plagioclase framework. Some 55% of the original amount of melt was removed. Switching to AMM with less (2 wt %) H2O changes little; for example, Atdtx = 0.6AMT + 0.4CS1 if minimising the fractionated melt component. The diatexite magma starts as a mixture of 0.42AMT + 0.58AMM, from which all the remaining melt was expelled when 48% crystallised. The resulting diatexite is 40% ‘melt product’ and 52% of the original amount of melt expelled.
Although the mass-balance calculations carried out do not give a unique solution (they might if trace-element compositions for the experimental glasses were available), they all point to the same robust conclusions, as follows. (1) The process forming ‘typical’ diatexite expelled a large amount of fractionated melt, ∼56% of that initially injected; this expelled, fractionated melt is crucial to the melt budget developed in a later section. (2) The ‘typical’ diatexite contains ∼43% ‘melt product’, which consists mainly of the early-crystallised minerals. (3) The process forming ‘typical’ diatexite magma begins with intrusion of ∼64% anatectic melt into metatexite. This is twice the threshold necessary for magma-like behaviour (Vigneresse et al., 1996), so injection would disaggregate the metatexite, forming a secondary diatexite magma capable of flow, developing a magmatic foliation and entraining schollen.
Petrogenesis of the leucocratic diatexites and leucogranites
In total, 22% (13 of 58) of diatexites are leucocratic and occur as patches and dyke-like bodies within mesocratic diatexite. There are two main types: one is leucotonalitic (5074 and 3127B) or leucogranodioritic (3237A, 5109A2, 5109A1 and 1181), with K2O content less than the anatectic melt; the other is leucomonzogranitic and more potassic (5109A8, 57, 5105A and 3311A) than the anatectic melt.
Leucodiatexite with a plagioclase-dominated framework
Tonalitic leucodiatexite 5074 has low K2O (1.23 wt %) and FeOT (2.31 wt %) contents, which precludes any metatexite component. Furthermore, high SiO2 and low Al2O3 contents indicate that a considerable amount of quartz crystallised before K-feldspar appeared. Simulated crystallisation of AMM containing 1.5 wt % H2O does this, and leucodiatexite 5074 lies near the orthopyroxene + plagioclase crystallised solid from a low degree of crystallisation of it (Fig. 11b), but there is also a small fractionated melt component present. A mass balance using this is leucodiatexite 5074 = 0.80CS1 + 0.2FL1, and thus it could be derived from AMM if, at 19% crystallisation (= XC of the composition of CS and FL used), 94% of the remaining melt was expelled. Although 100% ‘melt product’, it represents just 24% of the initial amount of anatectic melt; 76% was expelled. At solidification, this yields ∼6% interstitial K-feldspar to a plagioclase + orthopyroxene framework, as observed. Consequently, 5074 and similar tonalitic leucodiatexites are the early crystallisation products of an anatectic melt that did not entrain wall-rock metatexite.
Leucodiatexite with plagioclase + K-feldspar framework
Leucogranodioritic diatexite 3237A lies near to the composition of crystallised solids from between 10 and 29% crystallisation of AMM containing 2 wt % H2O (Fig. 11b). The best mass balance is achieved with the products from 17% crystallisation of AMM: 3237A = 0.5CS1 + 0.5FL1. It was produced from anatectic melt if at 17% crystallisation ∼79% of the remaining melt was removed and thus represents 34% of the initial amount of melt, with 66% expelled.
The combination of low (MgO+FeOT) and high Al2O3 (>16 wt %), Na2O+CaO (>5.7%) and K2O in leucogranodioritic diatexites 5109A1 and 5109A2 places strict limits on their formation. They contain little, or no, entrained wall rock, or orthopyroxene crystallised from anatectic melt, but have a large plagioclase component. This requires an initial anatectic melt that crystallised more plagioclase relative to orthopyroxene and quartz than AMM does. Assuming that Ashuanipi leucodiatexite 1017, which lies close to AMM on most compositional plots (e.g. Fig. 6), represents an anatectic melt composition, then simulations of its crystallisation meet these major element requirements. Both 5109A1 and 5109A2 lie near to tie-lines between the crystallised solids and fractionated liquids for <36% crystallisation of 1017 with 2 wt % H2O, and an approximate mass balance is 5109A2 ≈ 0.45CS1 + 0.55FL1. Thus, from the field relations, when a patch of anatectic melt in the diatexite reached 20% crystallisation, 69% of the remaining melt was expelled, and the plagioclase and trapped melt left behind became leucodiatexite 5109A2. About 55% of the initial amount of melt was expelled in forming these leucodiatexites.
Leucodiatexite with K-feldspar-dominated framework.
Sample 5109A8 is a narrow vein-like body of leucocratic diatexite in the same outcrop as 5109A1 and 5109A2. It has K-feldspar/plagioclase ∼1.26, K-feldspar/quartz ∼1.10 and quartz/plagioclase ∼1.19, and hence is too evolved to be the initial anatectic melt. In Fig. 11, 5109A8 lies close to a second-cycle fractionated liquid (FL2) composition. In the context of its outcrop, the fractionated melt expelled as leucodiatexites 5109A1 and 5109A2 formed may simply have intruded the adjacent diatexite, crystallised and become leucodiatexite 5109A8.
Two leucodiatexites (57 and 5105A) have K-feldspar-dominated frameworks, very high K2O contents (>6.3 wt %), moderate to high SiO2 (73 to 75 wt %), low Al2O (<14.1 wt %), Na2O/CaO of 2.8 to 3.6 and modal Kfs/Qz >1.25. They plot (Fig. 11) too far towards K-feldspar to be a FL1 from an initial anatectic melt. Sample 57 is nearest to (Fig. 11) the fractionated liquid (FL1) formed after ∼20% crystallisation of AMM. Using this FL1 as the starting composition, sample 57 can be modelled if, after reaching 27% crystallisation, 64% of the remaining melt is removed. This means that 47% of the initial amount of melt was expelled, leaving equal parts of second-cycle crystallised solid (CS2) and fractionated liquid (FL2) to form leucodiatexite 57. Leucodiatexite 5105A has 6.78 wt % K2O and plots close the second-cycle crystallised solids (CS2) obtained from the crystallisation of first-cycle fractionated liquids (FL1) extracted after >25% crystallisation of the anatectic melt (Fig. 11). The mass balance is 5105A = 0.85CS2 + 0.15FL2; that is, when a FL1 extracted after 27% crystallisation of AMM reached 30% crystallisation, 92% of the remaining melt was extracted from it, leaving a K-feldspar-dominated rock with ∼15% trapped melt; 64% of the initial amount of melt was expelled in the process.
Leucogranites
Ashuanipi leucogranites form two main groups on a QAP plot (Fig. 6). One (13 of 21 samples) lies in the granodiorite and the right side of the monzogranite fields on a trend from AMM to the plagioclase apex that crosses the modelled crystallised solids (CS1). Taking leucogranodiorite 17119 (Fig. 11b) and AMM with 2.5% H2O as the starting points, a mass balance is 17119 = 0.7CS1 + 0.3FL1. In geological terms, when a body of AMM reaches 25% crystallisation, 86% of the remaining melt has been removed, i.e. 65% of the initial amount of AMM has been expelled. The monzogranitic members of this group must contain more fractionated melt, or be from higher degrees of crystallisation because of their greater K2O contents. An approximate mass balance for samples 17131, 17115 (Fig. 11b) and 17134 using AMM with 2% H2O is (0.6CS1 + 0.4FL1). These three leucogranites formed when a body of AMM reached ∼46% crystallisation and 48% of the remaining melt, equivalent to ∼27% of the initial amount of AMM, was expelled.
The second group of seven leucogranites have >5 wt % K2O, plot below AMM in the monzogranite field on a QAP plot (Fig. 6), and lie between the CS2 and FL2 obtained from first-cycle fractionated liquids (FL1) extracted before 12% crystallisation of AMM (Fig. 11d). Representative samples 2180A and 2176A can be modelled as mixes of CS2 and FL2 from such a FL1, if after 30% crystallisation 57% of the remaining melt was removed; for example, leucogranite 2180A = 0.25CS2 + 0.75FL2. In this case, about 40% of the original melt body was expelled in making these leucogranites.
The most potassic leucogranite (17145) lies near (Fig. 11d) a CS2 derived from a FL1 segregated after AMM had passed 26% crystallisation. Starting with this FL1, a mass balance is 17145 = 0.6CS2 + 0.4FL2; in geological terms, when the FL1 reached 30% crystallisation, 83% of the remaining melt was removed, i.e. ∼66% of the initial amount of melt was expelled.
Repeated melt segregation in the Ashuanipi middle crust
Most Ashuanipi diatexites and leucogranites plot close to the crystallised solids in Figs 11 and 12; a few plot near to AMM or the fractionated melts FL1 and FL2. This indicates that almost all diatexites and leucogranites lost a significant amount of fractionated melt as they formed. Results from the mass-balance models imply that melt segregation from the Ashuanipi migmatites was long-lived. It began on the prograde path during melting and left behind melt-depleted metatexites. Much of the anatectic melt that was segregated then migrated to, and accumulated in, large-scale structural sites forming as the terrain shortened. There, it entrained metatexite wall rocks and became the secondary diatexite magma. Melt that did not entrain metatexite became either leucocratic diatexite or intrusions of leucogranite. Segregation continued on the retrograde path as the diatexite magma and anatectic melt progressively crystallised and was driven by shear-enhanced compaction of the crystal mush during ongoing regional transpression. The crystal-rich mush left behind became the ‘typical’ mesocratic diatexites, leucodiatexites (e.g. 5074 and 3237A) and leucogranites (e.g. 17119 and 17131) that have plagioclase-dominated frameworks (CS1), whereas the expelled, fractionated melts (FL1) became leucodiatexite, leucosome and leucogranite. As the segregated (FL1) melts accumulated and crystallised, they too were subject to episodic compaction, which expelled progressively more fractionated melts, leaving behind second-cycle crystal mushes that became leucosomes, leucogranites (e.g. 17145) and leucodiatexites (e.g. 5105A) with distinctive K2O-rich compositions (CS2) and characteristic compacted K-feldspar frameworks. Some of the second-cycle melts FL2 expelled at this stage became evolved leucodiatexite (e.g. 5109A8).
Leucogranites in the Opinaca Subprovince
The Opinaca Subprovince (Fig. 1) contains 63% leucogranite, mostly as thin dykes, but the melting of its metagreywackes and pelites produced <10% melt at the level now exposed there (Morfin et al., 2013). Thus, Morfin et al. (2014) interpreted the Opinaca to have been intruded by large amounts of felsic magma. Leucogranites from the Opinaca (red triangles in Fig. 11e; the major element compositions of the Opinaca leucogranites are given in Supplementary Data Electronic Appendix 5) show the same groupings as the Ashuanipi, but monzogranites are preponderant. The largest group (13 of 24) have moderate K2O (4.2 to 5.6 wt %) and (Na2O+CaO) (4.1 to 5.3 wt %) contents, and lie between the FL2 and CS2 obtained from fractionated melts extracted at 12% crystallisation of AMM. For example, leucogranite 3116a plots on the CS2 from 15% crystallisation of a FL1 extracted when AMM was 12% crystallised; it is essentially a CS2 cumulate. In contrast, the mass balance for sample 1081a indicates a mixture of 0.7CS2 + 0.3FL2 from the same first-cycle fractionated melt, but had melt removed when 23% crystallised. Leucogranite 1081a differs from 3116a only in that the final segregation of melt occurred at a ∼20oC lower temperature (temperature interval between 15% and 23% crystallisation), but both lost >65% of the original amount of melt.
A second group of monzogranites (6 of 24) have higher K2O (6.3 to 6.9 wt %) and lie (Fig. 11e) between the FL2 and CS2 derived from a FL1 expelled from AMM after >25% crystallisation. The composition of leucogranite 6225a, for example, lies close to the FL2-CS2 tie-line (Fig. 11c) after >15% crystallisation; it is essentially a K-feldspar cumulate (0.75CS2 + 0.25FL2) with ∼70% of the initial amount of melt expelled in making it. Leucogranite 6018A lies closer to FL2 and has more trapped melt (0.5CS2 + 0.5FL2); ∼66% of the initial amount of melt was expelled from it.
Three leucogranites lie in the granodiorite field in Fig. 11e. One of these, 5136a, lies between the crystallised solids and fractionated liquids from AMM with 1.5 wt % H2O. The mass balance with this starting melt is 5163a = 0.45CS1+ 0.55FL1, and thus it formed from AMM when upon reaching 14% crystallisation 79% of the remaining melt was removed. The other two may also be from an initial anatectic melt, although one with a higher H2O content.
Most (21 of 24, ∼85%) leucogranites in the Opinaca were derived from fractionated melts and typically have a high fraction of crystallised solids; just three of these have fractionated liquid compositions. Only two Opinaca leucogranites were clearly derived directly from an initial anatectic melt, although another may have been. In contrast, >50% of the Ashuanipi leucogranites crystallised directly from an initial anatectic melt. Thus, the composition of Opinaca leucogranites is consistent with their being derived from fractionated melts such as those expelled when diatexites and leucogranites formed in the Ashuanipi.
Melt budget for the Ashuanipi mapped surface
Guernina and Sawyer (2003) estimated the average degree of partial melting in the Ashuanipi to be ∼34 vol. % at the level exposed there now, based on the amount of peritectic orthopyroxene produced by the melt-producing reaction in the metatexites. Johnson et al. (2008) confirmed this with petrogenetic modelling that showed that Archean metagreywackes from the Quetico Subprovince (Fig. 1) would yield ∼30% melt during closed-system melting. Guernina and Sawyer (2003) also estimated that the entire Ashuanipi Subprovince produced ∼640 000 km3 of melt; this section examines where in the crust that melt went.
The first step is to estimate how much ‘melt product’ is at the present erosion level (PEL), i.e. the mapped surface. As greywacke is >10 times more abundant than metapelite, the protolith is considered to be metagreywacke. Subtracting the areas occupied by pre-anatectic mafic rocks, DIS and BIF and re-normalising, the area of protolith is [90 000 (1 – (0.03 + 0.04)] = 83 700 km2. Thus, anatexis produced 83 700 × 0.34 = 28 458 km2 of melt at the PEL. That melt has, of course, crystallised; its products are in the diatexites, leucosomes and leucogranites. Leucosomes and leucogranites are entirely ‘melt product’, but the diatexites are not. The mass-balance calculations show that ‘typical’ diatexite contains ∼43% ‘melt product’ as crystallised solid and trapped melt, melanocratic diatexite ∼18%, and leucodiatexite 100%. Therefore, the weighted total fraction of ‘melt product’ contained in the diatexites (32 mesocratic, 13 melanocratic and 13 leucocratic) is
((32/58) × 0.43) + ((13/58) × 0.18) + ((13/58) × 1.0) = 0.502.
Mesocratic melanocratic leucocratic
The metatexites also retain 0.2 in situ ‘melt product’: ∼0.05 as pseudomorphs after former melt (Sawyer, 2001) plus ∼0.15 as leucosomes. Furthermore, the ∼5% leucogranite sheets (Fig. 2) in the metatexite and diatexite that are too small to be mapped as leucogranite bodies are also ‘melt product’. The total ‘melt product’ at the PEL, after subtracting the post-anatectic granites and re-normalising area fractions as 0.176 metatexite, 0.517 diatexite and 0.222 leucogranite, and where 0.2, 0.502 and 1 are the melt fractions in the metatexite, diatexite and leucogranite respectively, and 0.05 is the fraction of leucogranite sills in the metatexites and diatexites, is
[((0.176 × 0.2) + (0.517 × 0.502) + (0.222 × 1) + ((0.176 + 0.517) × 0.05)] × 83 700 = 46 151 km2
Hence the PEL contains 1.62 times (46151/28458) more ‘melt product’ than the amount of anatectic melt produced there; most of this is as diatexite. However, modelling indicates a substantial loss of fractionated melt as the diatexites and leucogranites were formed, and thus ‘melt-product’ underestimates the amount of melt once at the PEL. The amount of melt once in the melanocratic and mesocratic diatexites is obtained from the AMM + AMT mix in each mass balance model presented earlier (i.e. 0.311 and 0.642 respectively) and amounts to {(13 × 0.517 × 0.311)/58 + (32 × 0.517 × 0.642)/58} × 83 700 = 18 344 km2. For the leucodiatexite and leucogranite (no metatexite component), this is obtained by adding back the expelled melt fraction. From the modelling, the tonalitic and granodioritic leucogranites (n= 13) lost ∼60% melt, the monzogranite (n=1) below (in Fig. 6) and left of AAM lost ∼70%, and the seven monzogranites below AMM lost ∼35%. The weighted bulk melt fraction lost from the 21 leucogranites is approximately ((13/21) × 0.60) + ((1/21) × 0.7) + ((7/21) × 0.35) = 0.521. Thus, the amount of melt once in the leucocratic diatexites and leucogranites is {((13 × 0.517)/58(1 – 0.59)) + (0.222) / (1 – 0.521))} × 83 700 = 62 448 km2. Still to be added is melt lost from the thin leucogranite sheets in metatexite and diatexite, as they do not have the composition of an initial melt; this amounts to (((0.176 + 0.517) × 0.05) / (1 – 0.521)) × 83 700 = 6055 km2. Summed over metatexite, diatexite and leucogranite, the total area of melt once at the PEL is 86 847 km2. This is 3.05 (86 847/28 458) times the amount produced there, and hence the crustal level now exposed at the PEL was one of significant melt accumulation. The melt flux into the PEL was 3.05 – 1.0 = 2.05 and that out from it was 3.05 – 1.62 = 1.43 times the amount of melt made at the PEL (Fig. 14). The diatexites at the present exposure level, corresponding to ∼26 km depth, are mostly cumulate rocks. Melt was made there, even more melt was added to that, and then during crystallisation a large amount of fractionated melt was expelled.
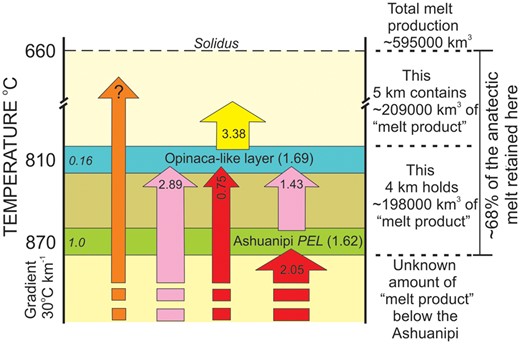
Diagram illustrating the melt budget for crustal anatexis and reworking of the Ashuanipi crust during late Archean orogenesis showing the melt fluxes into and out of the Ashuanipi (green) and Opinaca (blue) presently at the surface. The Ashuanipi and Opinaca subprovinces are large and show topographic and structural relief, therefore, each is represented by a layer 2 km thick, the mid-points of which are respectively at 870oC and 810oC. The number in italics at the left is the proportion of anatectic melt made at that level normalised to the Ashuanipi production, and the number in parentheses is the net gain of ‘melt product’ in the layer. Mass-balance modelling indicates a large flux (2.05) of initial anatectic melt (red arrow) into and fractionated melt (1.43, pink arrow) from the Ashuanipi; the difference is the net gain of ‘melt product’. The Opinaca contains abundant leucogranite, most with the compositions of early-crystallised solids: this requires a very large input of fractionated melt (1.43 and 2.89, pink arrows) from the Ashuanipi and farther below, in addition to a small influx (0.75, red arrow) of initial anatectic melt. Crystallisation of this added melt released a large amount (3.38, yellow arrow) of highly fractionated melt from the Opinaca-like layer. The orange arrow represents a potential, small, flux of melt (initial and fractionated) from below Ashuanipi; its magnitude depends on the amount of melt held below the Ashuanipi. The volumes of melt retained in the Ashuanipi and Opinaca-like layers and above to the solidus are shown on the right, assuming a gradient of 30oC km-1; note the break in the vertical scale just below the solidus.
Melt budget for reworking of the Ashuanipi crust
The objective in this section is to extend the 2-D melt budget to a 3-D one for the whole crustal section above and below the level (∼26 km) of the present-day surface. Because the slightly shallower crustal level exposed in the Opinaca gained, and the deeper one in the Ashuanipi lost fractionated anatectic melt, an Opinaca-like layer is assumed to have overlain the crustal level exposed in the Ashuanipi. Assuming a thermal gradient of 30oC km-1 and T=810oC (Morfin et al., 2013) for the exposed Opinaca surface, it lies 2 km above the Ashuanipi PEL (Fig. 13). This Opinaca-like layer has 63% leucogranite, that is 1.85 times (i.e. {(0.63× 83 700)/28 458}) more melt than made at the Ashuanipi PEL. Metagreywackes in the Opinaca produce <10% melt at 810oC; therefore, anatexis accounts for <0.16 (i.e. 0.1/0.63) of the total melt product there, and the fraction of leucogranite gained is 1.85 – 0.16 = 1.69. Of that melt gain, at least 85% was fractionated and <15% an initial anatectic melt, and hence the proportions of ‘melt product’ from these two sources are ∼1.44 (i.e. 0.85 × 1.69) and ∼0.25 (i.e. 0.15 × 1.69) respectively. The flux of fractionated melt leaving the Ashuanipi PEL (1.43) was the same as the ‘melt product’ gained by the Opinaca-like layer (1.44), but this flux was, nevertheless, insufficient. More melt had to be added. This is because almost all Opinaca leucogranites are compositionally crystallised solids, either CS1 or CS2 (Figs 11 and 12), which lost on average 66.7% of the initial amount of melt, and this lost melt was highly fractionated. Therefore, the total influx of melt into the Opinaca-like layer is the sum of the flux of fractionated (pink arrow in Fig. 14) and initial anatectic (red arrow in Fig 14) melts; these are respectively ∼4.32 (i.e. 1.44 × 3 of which 1.43 is from the Ashuanipi) and 0.75 (i.e. 0.25 × 3). The flux of fractionated melt out of the Opinaca-like layer after its leucogranites formed and based on their composition is approximately {(4.32+0.75) × 0.667)} = 3.38 (yellow arrow in Fig. 14).
Assuming that the Ashuanipi PEL and Opinaca-like layer are each 2-km thick, then the volume of ‘melt product’ retained in them is (46151 × 2) + (83700 × 0.63 × 2) = 197 764 km3, i.e. ∼198 000 km3. If thin dykes of fractionated leucogranite magma ascend to the solidus (∼660oC) and the injection complex that formed below it contains 50% leucogranite as Leitch and Weinberg (2002) suggest, then the 5.0 km (810–660o/30oC km-1) of crust between the solidus and the Opinaca-like layer contains another 83 700 × 5.0 × 0.5 = 209 250 km3 (i.e. ∼209 000 km3) of ‘melt product’ and this would be highly fractionated. Thus at least 68% (i.e. 100[(197 764 + 209 250) / (640 000 × 0.93)]) of the volume of melt (corrected for the smaller area of protolith used in this study, i.e. 83 700 cf 90 000 km2) produced during regional anatexis remained between the PEL and the solidus (Fig. 14 right). More ‘melt product’ must lie below the PEL and should be included, but the depth to which diatexites and leucogranites extend is unknown. However, if the same proportions of diatexite and leucogranite extended just 4.1 km (i.e. [(640 000 × 0.93) – 407 014 km3] / 46 151 km2) deeper (melt-depleted rocks would lie below), then all the melt is accounted for and none may have risen past the solidus.
The efficacy of crustal-scale melt migration expressed as (melt passed × 100) / (melt generated) from the PEL to the subsolidus upper crust for the Ashuanipi anatectic event was <32%, i.e. ((100 (595 200 – 394 459)) / 595 200). The overall efficacy must be less owing to the undetermined amount of ‘melt product’ below the PEL. In comparison, the efficacy of the short length-scale processes as melt is segregated from its metatexite residuum is ∼85%. The discrepancy between these two estimates of efficacy means that the ascent of most of the anatectic melt ended in the middle crust.
Yakymchuck and Brown (2014) examined conditionally drained melting in which melt is removed once a threshold value of 7% is reached, and found melt productivity to be halved. If that scenario applied to the Ashuanipi, the melt volume produced would be reduced to ∼300 000 km3. However, the melt budget derived from the diatexites and leucogranites indicates that more melt than this was retained in the middle crust. If conditional melting occurred in the Ashuanipi, then the melt budget implies that no granite magma reached the upper crust. Conditional melting may not be generally applicable to crustal melting.
Implications for crustal reworking and differentiation
The metagreywacke protolith in the Ashuanipi Subprovince was relatively homogeneous, and field relations indicate that the ascent of anatectic melt during late Archaean orogenic shortening there was arrested mainly at major shear zones. In other terranes, the ascent of anatectic melt may be impeded by lithological discontinuities, such as unconformities or thick marble units, where the upward propagation of the fractures, along which melt ascends, is stopped, leading to the accumulation of melt under the discontinuity (Hall & Kisters, 2012, 2016; Kruger & Kisters, 2016). Arrested ascent and accumulation of anatectic melt in the middle crust may, therefore, be common.
The accumulation of large volumes of melt in the middle crust retains the heat of crystallisation there and prolongs orogenic cooling. This enhances the effect of processes such as assimilation and crystal fractionation, giving rise to the widespread development of crystal mushes. Each increment of deformation during orogenic contraction compacts the crystallising mushes, driving melt from them (Rutter & Neumann, 1995). Thus, progressively more fractionated melts are expelled, move upwards, become trapped again and undergo further compaction. This imparts a very different geochemical signature to crustal reworking compared with that if melt were to move rapidly and directly to the upper crust. Consequently, the middle crust where the anatectic melt accumulated becomes compositionally differentiated with two very different parts. The deeper part, now secondary diatexite in the Ashuanipi, consists of compacted former crystal mushes (derived from a mixture of accumulated anatectic melts and a lesser amount of residual wall rocks) that is enriched in the components held in the phases that crystallised early from the anatectic melt, in this case plagioclase and orthopyroxene. The upper part is an injection complex of leucogranite dykes enriched in components, such as K2O and Na2O that partition into the fractionated melts expelled from the underlying crystal mushes, and extends to the solidus. Moreover, as crystallisation advances, an H2O-rich fluid exsolves from the melt and may further enhance chemical fractionation by fluxing H2O, K2O and SiO2 into the mobile fraction (Rodriguez & Castro, 2017). As the host rocks are at supra solidus temperatures, the exsolved H2O fluid may also induce partial melting by increasing aH2O (Schwindinger & Weinberg, 2017).
CONCLUSIONS
The diatexites and leucogranites in the Ashuanipi Subprovince are petrologically diverse rocks that contain a wide range of microstructures and compositions critical to an understanding of their petrogenesis. Feldspar framework microstructures range from plagioclase-dominated (Pl + Opx) through Pl + Kfs + Opx to K-feldspar-dominated (Kfs + Pl ± Opx), and although a few frameworks are open, most show evidence of compaction. The principal compositional trait across the suite of diatexites and leucogranites is the inverse variation in (Na2O+CaO) and K2O contents. Modelling indicates that most diatexites and leucogranites in the Ashuanipi are accumulations of the minerals that crystallised first (Pl + Opx frameworks) from anatectic melt or from a fractionated melt (Kfs + Pl frameworks) derived from an initial anatectic melt, and that the formation of these ‘cumulate’ compositions expelled >50% fractioned melt. The morphology of leucosome arrays and the macroscopic structure in the diatexites indicate that they were deformed whilst partially molten; therefore, shear-enhanced compaction likely drove the expulsion of melt as the anatectic rocks slowly crystallised. Segregation of much of the remaining melt from the crystallised phases was a widespread and essentially continuous process affecting the anatectic melt as it crystallised in the middle crust.
Quantification of the melt budget for the Ashuanipi migmatite terrain reveals that at least 68% (∼400 000 km3) of the anatectic melt produced there remained below the solidus, with half of that trapped in granulite-facies rocks. The accumulation of melt near its source and subsequent entrainment of hot residual wall rocks produced a large volume of secondary diatexite magma. As this magma slowly crystallised in the mid-crust (∼26 km) it underwent episodes of compaction, which expelled fractionated melt. The crystal mushes left behind became the secondary diatexite migmatites, which have a characteristic plagioclase signature to their composition and microstructure. The expelled fractionated melts migrated upwards and formed an injection complex of leucogranite directly above the diatexite and up to the solidus.
Because most anatectic terrains were deformed with melt present and continental crust is mechanically anisotropic, the ascent of anatectic melt in collisional orogenic settings will be retarded at deep crustal structural and lithological discontinuities. Crustal reworking may then be characterised by a compositionally zoned mid-crust with large volumes of plagioclase-rich diatexite below an injection complex of highly fractionated leucogranite.
ACKNOWLEDGEMENTS
I thank Daniel Bandyayera, Samuel Morfin, Bruna Carvalho, Johann Diener, Alex Kisters, Tolene Kruger and Richard White for illuminating visits to their migmatite terrains. I thank the journal reviewers, Chris Yakymchuk, Richard White and an anonymous reviewer for their constructive comments and the improvements that they suggested.
FUNDING
This work was supported by a Natural Sciences and Engineering Research Council of Canada Discovery Grant [number 03694] to the author.
SUPPLEMENTARY DATA
Supplementary data are available at Journal of Petrology online.