-
PDF
- Split View
-
Views
-
Cite
Cite
Lisa-Marie Schmid, Nikolay Manavski, Wei Chi, Jörg Meurer, Chloroplast Ribosome Biogenesis Factors, Plant and Cell Physiology, Volume 65, Issue 4, April 2024, Pages 516–536, https://doi.org/10.1093/pcp/pcad082
- Share Icon Share
Abstract
The formation of chloroplasts can be traced back to an ancient event in which a eukaryotic host cell containing mitochondria ingested a cyanobacterium. Since then, chloroplasts have retained many characteristics of their bacterial ancestor, including their transcription and translation machinery. In this review, recent research on the maturation of rRNA and ribosome assembly in chloroplasts is explored, along with their crucial role in plant survival and their implications for plant acclimation to changing environments. A comparison is made between the ribosome composition and auxiliary factors of ancient and modern chloroplasts, providing insights into the evolution of ribosome assembly factors. Although the chloroplast contains ancient proteins with conserved functions in ribosome assembly, newly evolved factors have also emerged to help plants acclimate to changes in their environment and internal signals. Overall, this review offers a comprehensive analysis of the molecular mechanisms underlying chloroplast ribosome assembly and highlights the importance of this process in plant survival, acclimation and adaptation.
Introduction
The composition of the chloroplast ribosome
Ribosomes are essential components of the translational machinery for all living cells. Advances in crystallization have enabled a detailed understanding of their composition and complex structure in various species (Ramakrishnan 2002, Wilson and Doudna Cate 2012, Bieri et al. 2017). Chloroplasts, similar to eubacteria, possess 70S-type ribosomes consisting of a small 30S subunit (SSU) and a large 50S subunit (LSU), which are named after their sedimentation coefficient (Harris et al. 1994, Garrett 1999). The 30S subunit of chloroplasts contains 25 ribosomal proteins (RPs) and one 16S rRNA molecule, while the 50S subunit houses three distinct rRNA species (23S, 5S and 4.5S) and 33 RPs (Yamaguchi and Subramanian 2000, Yamaguchi et al. 2000, Stoppel and Meurer 2012). A comparison of the protein content shows that chloroplast ribosomes lack the eubacterial subunits L25 and L30 but have five plant-specific RPs referred to as PSRP2-6. These proteins are believed to compensate for differences in ribosome structure and to participate in an alternative translation initiation based on their location in crystal structures (Yamaguchi and Subramanian 2003, Ahmed et al. 2017).
Moreover, chloroplast RPs are often larger than their eubacterial counterparts, which suggests that novel functions have been recruited and a co-evolution has taken place between RPs and their rRNA interaction sites (Manuell et al. 2004, Graf et al. 2017). Importantly, several ribosomal subunits have been found to be essential, as knockout mutants for these subunits result in embryo lethality in Arabidopsis thaliana (Tiller and Bock 2014, Zoschke and Bock 2018, Meinke 2020). In contrast, other subunits such as PRPL11, RPL33 or RPS15 are dispensable, but they contribute to proper ribosome functionality and accurate plastid translation (Pesaresi et al. 2001, Rogalski et al. 2008, Fleischmann et al. 2011). Interestingly, some of the non-essential RPs in bacteria, such as RPL1, RPL32 and RPS15, have gained essential roles in plant ribosomes, highlighting the differences that have arisen during 1.6 billion years of evolution (Tiller and Bock 2014).
In terms of rRNA, the core sequences remained highly conserved between chloroplasts and eubacteria, except that the plastid 16S rRNA is slightly shorter than the Escherichia coli 16S rRNA (Graf et al. 2017). It is worth noting that in eubacteria, the 4.5S sequence is not a separate fragment, as in chloroplasts, but rather an integral part of the bacterial 23S rRNA (Edwards and Kössel 1981). Additionally, the chloroplast 23S rRNA undergoes another round of processing at two defined positions, known as “hidden breaks A and B”, resulting in three differently sized 23S rRNA species (Nishimura et al. 2010). Consequently, these differences lead to a slightly altered overall shape and positioning, particularly regarding the 23S/4.5S rRNA species within the 50S subunit (Bieri et al. 2017).
Plastid ribosomes differ principally from their eubacterial counterparts in their chimeric composition, consisting of both plastid- and nucleus-encoded RPs. This adds a layer of complexity as these highly abundant structural components need to be expressed and delivered to the chloroplast in the correct ratio. In the course of evolution, most organellar genes have been transferred to the nucleus, leaving only a small number of genes, including rDNAs, still transcribed by the plastid transcriptional machinery (Sato et al. 1999, Börner et al. 2015).
Chloroplast ribosome biogenesis
It has been proposed that membrane-associated nucleoids are the sites of important steps in chloroplast RNA metabolism, including ribosome assembly (Bohne 2014). This idea is supported by the identification of factors involved in rRNA processing and ribosome assembly within nucleoids, as revealed by studies on sub-localization or proteomic approaches (Majeran et al. 2012, Kleinknecht et al. 2014, Wang et al. 2016a, Meurer et al. 2017, Schmid et al. 2019, Méteignier et al. 2021). Reports from bacteria suggest that ribosome biogenesis also takes place within nucleoids, while translation primarily occurs on mature ribosomes in the cytoplasm (Bakshi et al. 2015). This spatial separation is supported by the fact that several rRNA processing events take place in a co-transcriptional way. At later stages, processing steps also occur in an assembly-assisted manner outside of nucleoids (Shajani et al. 2011).
The biogenesis of ribosomes is a highly complex process that involves the proper processing, covalent modification and restructuring of various rRNA species, as well as the coordinated assembly of RPs in a spatiotemporal manner (Stoppel and Meurer 2012, Manavski et al. 2021). While the pathways involved in ribosome biogenesis are better understood in eubacteria, with detailed assembly maps and characterization of approximately 100 auxiliary factors, the same level of understanding is lacking for chloroplasts (Kaczanowska and Ryden-Aulin 2007, Shajani et al. 2011). However, in recent years, numerous chloroplast ribosome assembly factors involved in rRNA processing, maturation, restructuring and coordinated insertion of RPs into the functional ribosomal complex have been discovered and characterized (Fig. 1–3; Table. 1).
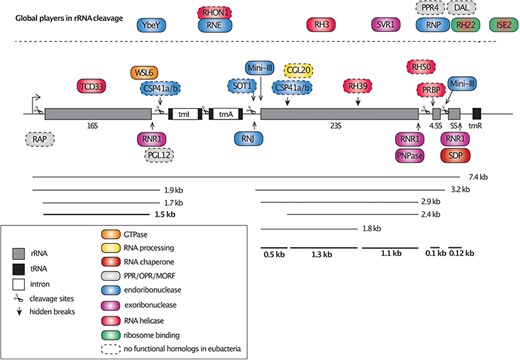
The arrangement of the rrn operon in chloroplasts and RNA processing factors. The four rRNA species of chloroplasts are organized in one operon and are transcribed as a single polycistronic precursor. As indicated by arrows and scissors, the precursor is then cleaved at several positions and undergoes further processing to form the mature rRNA species (bold lines). The nuclear-encoded PPR and OPR proteins, endo- and exoribonucleases and RNA-helicases and ribosome-binding factors with defined roles in rRNA processing and maturation are indicated at the respective positions and color-coded according to their function and activity. Factors that lack eubacterial counterparts were framed with dashed lines (see legend for details).
Protein . | Arabidopsis identifier . | Activity/interaction/family/domain . | Proposed function in ribogenesis . | References . |
---|---|---|---|---|
Ribonucleases | ||||
PNPase | At3g03710 | 3ʹ-5ʹ exoribonuclease | Maturation of 23S rRNA 3ʹ ends | Yehudai-Resheff et al. 2001 |
RNE | At2g04270 | Endunuclease | Production of the dicistronic 23S-4.5S rRNA precursor | Stoppel et al. 2012 |
RNR1 | At5g02250 | 3ʹ-5ʹ exoribonuclease | 3ʹ trimming of 23S, 16S and 5S rRNAs | Kishine et al. 2004; Bollenbach et al. 2005 |
RNJ | At5g63420 | Endoribonuclease and 5′-to-3′ exoribonuclease | Trimming of 16S and 23S 5ʹ rRNA termini and quality control | Hotto et al. 2020 |
RNC3/4 | At1g55140/At3g13740 | Endonucleases, mini-ribonuclease III | rRNA maturation and intron recycling | Hotto et al. 2015 |
CSP41a/b | AT3G63140/AT1G09340 | Endoribonuclease | Association and stability of 23S and 16S rRNAs; processing of hidden break A | Beligni and Mayfield 2008 |
YbeY | AT2G25870 | Endoribonuclease | Maturation of the 5ʹ and 3ʹ ends of 16S, 23S and 4.5S rRNAs | Liu et al. 2015 |
Helicases | ||||
RH39 | AT4G09730 | DEAD-box RNA helicase, binds to and associates in cleavage of 23S rRNA | Processing of a stem-loop structure of 23S rRNA, introduction of the hidden break B site into the 23S rRNA | Nishimura et al. 2010 |
RH22 | AT1G59990 | DEAD-box RNA helicase, interacts with RPL24 | Rearrange secondary structures and processing of the 23S-4.5S precursor rRNA | Chi et al. 2012 |
RH50 | At3g06980 | DEAD-box RNA helicase, binds to rRNAs | Maturation of the 23S and 4.5S rRNAs | Paieri et al. 2018 |
RH3 | At5g26742 | DEAD-box RNA helicase | Associates with Group II introns and 23S rRNA, chaperone function | Asakura et al. 2012; Gu et al. 2014 |
ISE2 | AT1G7007 | Ski2-like RNA helicase | RNA editing and splicing of mRNAs for RPs, rRNA processing | Bobik et al. 2017 |
PRBP (EMB1513) | AT2G37920 | DEAD-box RNA helicase | Maturation of the 4.5 rRNA | Park et al. 2011 |
RHON1 | AT1G06190 | Rho-like RNA helicase | Guides RNE to specific targets, rRNA processing | Stoppel et al. 2012 |
GTPases | ||||
AtRsgA | AT1G67440 | GTPase A, interacts with RbfA | Maturation of 16S rRNA | Janowski et al. 2018 |
WSL6 (Era-1) | AT5G66470 | Era-type GTPase | Binds to the 3ʹ end region of 16S rRNA, 30S assembly | Suwastika et al. 2014 |
YqeH (AtNOA1) | AT3G47450 | YqeH class GTPase | cGTPase participating in 30S ribosome assembly | Li et al. 2015 |
BPG2 | AT3G57180 | YqeH-type GTPase | Maturation of 16S and 23S rRNA | Komatsu et al. 2010 |
ObgC | AT5G18570 | Obg GTPases | Binds to 23S rRNA, 50S ribosome assembly | Bang et al. 2012 |
RbgA | AT4G02790 | RbgA GTPase | Interacts with the plastid RPs RPL6 and RPL35, GTP hydrolysis promotes its dissociation from the 50S subunit | Seffouh et al. 2019; Jeon et al. 2017 |
EMB2738 (DER) | AT3G12080 | Double Era-like GTPase | Associated with 50S ribosomal, accumulation of ribosomes | Jeon et al. 2014 |
RNA chaperones | ||||
PAC | AT2G48120 | RNA binding | PAC, co-migrates with premature 50S ribosomal particles, binding to and remodeling a specific region of the 23S rRNA | Meurer et al. 1998; Meurer et al. 2017; Tirlapur et al. 1999 |
CFM4 | AT4G39040 | CRM family member subfamily4 | Processing of 16S and 4.5S rRNA | Lee et al. 2014 |
SDP | AT1G12800 | S1 domain–containing RNA-binding protein | Processing of 23S–4.5S | Han et al. 2015 |
SRRP1 | At3g23700 | S1 domain–containing RNA-binding protein | Splicing of trnL intron and cleavage of the 5S rRNA | Gu et al. 2015 |
Other proteins involved in ribogenesis | ||||
RBF1 | AT4G34730 | RBF A domain, interacts with AtRsgA | 3ʹ and 5ʹ end maturation of 16S rRNA | Fristedt et al. 2014 |
SOT1 | AT5G46580 | PPR-SMR protein, endonuclease | Protects 5ʹ 23S-4.5S dicistronic precursor from exonucleolytic activity of RNJ | Zhang and Lu 2019 |
DCL | AT1G45230 | DUF3223 domain | Generating the 4.5S rRNA | Bellaoui et al. 2003 |
RAP | AT2G31890 | OPR protein | 5ʹ processing of 16S and 23S rRNA | Kleinknecht et al. 2014 |
CRASS | AT5G14910 | Putative heavy metal–associated (HMA) domain | Associates with 16S rRNA and RPs of the small 30S subunit | Pulido et al. 2018 |
WCO (WHITECOTYLEDONS) | Genetically maps on chromosome 1 | Not identified yet | Development- and/or cell-specific 16S maturation | Yamamoto et al. 2000 |
PGL12 | AT1G71460 | PLS-type PPR harboring an RRM domain | Assists in the processing of 16S rRNA | Chen et al. 2019 |
TIC56 | AT5G01590 | Protein of the plastid import machinery | Processing of 23S rRNA and 50S ribosome assembly | Köhler et al. 2016 |
CGL20a/b | AT2G17240/AT3G24506 | Prolin-rich ∼10-kD protein | 50S ribosome biogenesis via the formation of a hidden break A in the 23S rRNA | Reiter et al. 2020 |
PPR4 (WSL5) | AT5G04810 | P-type PPR protein carrying an RRM domain | Editing and rps12 intron 1 trans-splicing and, indirectly, in the assembly of 70S ribosomes | Liu et al. 2018; Lee et al. 2019 |
DAL (MORF2) | AT2G33430 | Part of the MORF family | Splicing and editing of transcripts encoding RPs | Takenaka et al. 2012 |
rRNA modifications | ||||
CMAL | AT5G10910 | MraW/RsmH-like methyltransferase | m4Cm methylation of 16S rRNA at position C1352 | Manavski et al. 2021 |
RsmD | At3g28460 | N2-methylguanosine methyltransferase | m2G methylation of 16S rRNA at position C915 | Manavski et al. 2021 |
PFC | AT1G01860 | KsgA and DIM1 homolog | m6A methylation of 16S rRNA at position C1352 | Manavski et al. 2021 |
SVR1 | AT2G39140 | Ψ synthase | Pseudouridylation of rRNAs | Sun et al. 2019b |
Protein . | Arabidopsis identifier . | Activity/interaction/family/domain . | Proposed function in ribogenesis . | References . |
---|---|---|---|---|
Ribonucleases | ||||
PNPase | At3g03710 | 3ʹ-5ʹ exoribonuclease | Maturation of 23S rRNA 3ʹ ends | Yehudai-Resheff et al. 2001 |
RNE | At2g04270 | Endunuclease | Production of the dicistronic 23S-4.5S rRNA precursor | Stoppel et al. 2012 |
RNR1 | At5g02250 | 3ʹ-5ʹ exoribonuclease | 3ʹ trimming of 23S, 16S and 5S rRNAs | Kishine et al. 2004; Bollenbach et al. 2005 |
RNJ | At5g63420 | Endoribonuclease and 5′-to-3′ exoribonuclease | Trimming of 16S and 23S 5ʹ rRNA termini and quality control | Hotto et al. 2020 |
RNC3/4 | At1g55140/At3g13740 | Endonucleases, mini-ribonuclease III | rRNA maturation and intron recycling | Hotto et al. 2015 |
CSP41a/b | AT3G63140/AT1G09340 | Endoribonuclease | Association and stability of 23S and 16S rRNAs; processing of hidden break A | Beligni and Mayfield 2008 |
YbeY | AT2G25870 | Endoribonuclease | Maturation of the 5ʹ and 3ʹ ends of 16S, 23S and 4.5S rRNAs | Liu et al. 2015 |
Helicases | ||||
RH39 | AT4G09730 | DEAD-box RNA helicase, binds to and associates in cleavage of 23S rRNA | Processing of a stem-loop structure of 23S rRNA, introduction of the hidden break B site into the 23S rRNA | Nishimura et al. 2010 |
RH22 | AT1G59990 | DEAD-box RNA helicase, interacts with RPL24 | Rearrange secondary structures and processing of the 23S-4.5S precursor rRNA | Chi et al. 2012 |
RH50 | At3g06980 | DEAD-box RNA helicase, binds to rRNAs | Maturation of the 23S and 4.5S rRNAs | Paieri et al. 2018 |
RH3 | At5g26742 | DEAD-box RNA helicase | Associates with Group II introns and 23S rRNA, chaperone function | Asakura et al. 2012; Gu et al. 2014 |
ISE2 | AT1G7007 | Ski2-like RNA helicase | RNA editing and splicing of mRNAs for RPs, rRNA processing | Bobik et al. 2017 |
PRBP (EMB1513) | AT2G37920 | DEAD-box RNA helicase | Maturation of the 4.5 rRNA | Park et al. 2011 |
RHON1 | AT1G06190 | Rho-like RNA helicase | Guides RNE to specific targets, rRNA processing | Stoppel et al. 2012 |
GTPases | ||||
AtRsgA | AT1G67440 | GTPase A, interacts with RbfA | Maturation of 16S rRNA | Janowski et al. 2018 |
WSL6 (Era-1) | AT5G66470 | Era-type GTPase | Binds to the 3ʹ end region of 16S rRNA, 30S assembly | Suwastika et al. 2014 |
YqeH (AtNOA1) | AT3G47450 | YqeH class GTPase | cGTPase participating in 30S ribosome assembly | Li et al. 2015 |
BPG2 | AT3G57180 | YqeH-type GTPase | Maturation of 16S and 23S rRNA | Komatsu et al. 2010 |
ObgC | AT5G18570 | Obg GTPases | Binds to 23S rRNA, 50S ribosome assembly | Bang et al. 2012 |
RbgA | AT4G02790 | RbgA GTPase | Interacts with the plastid RPs RPL6 and RPL35, GTP hydrolysis promotes its dissociation from the 50S subunit | Seffouh et al. 2019; Jeon et al. 2017 |
EMB2738 (DER) | AT3G12080 | Double Era-like GTPase | Associated with 50S ribosomal, accumulation of ribosomes | Jeon et al. 2014 |
RNA chaperones | ||||
PAC | AT2G48120 | RNA binding | PAC, co-migrates with premature 50S ribosomal particles, binding to and remodeling a specific region of the 23S rRNA | Meurer et al. 1998; Meurer et al. 2017; Tirlapur et al. 1999 |
CFM4 | AT4G39040 | CRM family member subfamily4 | Processing of 16S and 4.5S rRNA | Lee et al. 2014 |
SDP | AT1G12800 | S1 domain–containing RNA-binding protein | Processing of 23S–4.5S | Han et al. 2015 |
SRRP1 | At3g23700 | S1 domain–containing RNA-binding protein | Splicing of trnL intron and cleavage of the 5S rRNA | Gu et al. 2015 |
Other proteins involved in ribogenesis | ||||
RBF1 | AT4G34730 | RBF A domain, interacts with AtRsgA | 3ʹ and 5ʹ end maturation of 16S rRNA | Fristedt et al. 2014 |
SOT1 | AT5G46580 | PPR-SMR protein, endonuclease | Protects 5ʹ 23S-4.5S dicistronic precursor from exonucleolytic activity of RNJ | Zhang and Lu 2019 |
DCL | AT1G45230 | DUF3223 domain | Generating the 4.5S rRNA | Bellaoui et al. 2003 |
RAP | AT2G31890 | OPR protein | 5ʹ processing of 16S and 23S rRNA | Kleinknecht et al. 2014 |
CRASS | AT5G14910 | Putative heavy metal–associated (HMA) domain | Associates with 16S rRNA and RPs of the small 30S subunit | Pulido et al. 2018 |
WCO (WHITECOTYLEDONS) | Genetically maps on chromosome 1 | Not identified yet | Development- and/or cell-specific 16S maturation | Yamamoto et al. 2000 |
PGL12 | AT1G71460 | PLS-type PPR harboring an RRM domain | Assists in the processing of 16S rRNA | Chen et al. 2019 |
TIC56 | AT5G01590 | Protein of the plastid import machinery | Processing of 23S rRNA and 50S ribosome assembly | Köhler et al. 2016 |
CGL20a/b | AT2G17240/AT3G24506 | Prolin-rich ∼10-kD protein | 50S ribosome biogenesis via the formation of a hidden break A in the 23S rRNA | Reiter et al. 2020 |
PPR4 (WSL5) | AT5G04810 | P-type PPR protein carrying an RRM domain | Editing and rps12 intron 1 trans-splicing and, indirectly, in the assembly of 70S ribosomes | Liu et al. 2018; Lee et al. 2019 |
DAL (MORF2) | AT2G33430 | Part of the MORF family | Splicing and editing of transcripts encoding RPs | Takenaka et al. 2012 |
rRNA modifications | ||||
CMAL | AT5G10910 | MraW/RsmH-like methyltransferase | m4Cm methylation of 16S rRNA at position C1352 | Manavski et al. 2021 |
RsmD | At3g28460 | N2-methylguanosine methyltransferase | m2G methylation of 16S rRNA at position C915 | Manavski et al. 2021 |
PFC | AT1G01860 | KsgA and DIM1 homolog | m6A methylation of 16S rRNA at position C1352 | Manavski et al. 2021 |
SVR1 | AT2G39140 | Ψ synthase | Pseudouridylation of rRNAs | Sun et al. 2019b |
Protein . | Arabidopsis identifier . | Activity/interaction/family/domain . | Proposed function in ribogenesis . | References . |
---|---|---|---|---|
Ribonucleases | ||||
PNPase | At3g03710 | 3ʹ-5ʹ exoribonuclease | Maturation of 23S rRNA 3ʹ ends | Yehudai-Resheff et al. 2001 |
RNE | At2g04270 | Endunuclease | Production of the dicistronic 23S-4.5S rRNA precursor | Stoppel et al. 2012 |
RNR1 | At5g02250 | 3ʹ-5ʹ exoribonuclease | 3ʹ trimming of 23S, 16S and 5S rRNAs | Kishine et al. 2004; Bollenbach et al. 2005 |
RNJ | At5g63420 | Endoribonuclease and 5′-to-3′ exoribonuclease | Trimming of 16S and 23S 5ʹ rRNA termini and quality control | Hotto et al. 2020 |
RNC3/4 | At1g55140/At3g13740 | Endonucleases, mini-ribonuclease III | rRNA maturation and intron recycling | Hotto et al. 2015 |
CSP41a/b | AT3G63140/AT1G09340 | Endoribonuclease | Association and stability of 23S and 16S rRNAs; processing of hidden break A | Beligni and Mayfield 2008 |
YbeY | AT2G25870 | Endoribonuclease | Maturation of the 5ʹ and 3ʹ ends of 16S, 23S and 4.5S rRNAs | Liu et al. 2015 |
Helicases | ||||
RH39 | AT4G09730 | DEAD-box RNA helicase, binds to and associates in cleavage of 23S rRNA | Processing of a stem-loop structure of 23S rRNA, introduction of the hidden break B site into the 23S rRNA | Nishimura et al. 2010 |
RH22 | AT1G59990 | DEAD-box RNA helicase, interacts with RPL24 | Rearrange secondary structures and processing of the 23S-4.5S precursor rRNA | Chi et al. 2012 |
RH50 | At3g06980 | DEAD-box RNA helicase, binds to rRNAs | Maturation of the 23S and 4.5S rRNAs | Paieri et al. 2018 |
RH3 | At5g26742 | DEAD-box RNA helicase | Associates with Group II introns and 23S rRNA, chaperone function | Asakura et al. 2012; Gu et al. 2014 |
ISE2 | AT1G7007 | Ski2-like RNA helicase | RNA editing and splicing of mRNAs for RPs, rRNA processing | Bobik et al. 2017 |
PRBP (EMB1513) | AT2G37920 | DEAD-box RNA helicase | Maturation of the 4.5 rRNA | Park et al. 2011 |
RHON1 | AT1G06190 | Rho-like RNA helicase | Guides RNE to specific targets, rRNA processing | Stoppel et al. 2012 |
GTPases | ||||
AtRsgA | AT1G67440 | GTPase A, interacts with RbfA | Maturation of 16S rRNA | Janowski et al. 2018 |
WSL6 (Era-1) | AT5G66470 | Era-type GTPase | Binds to the 3ʹ end region of 16S rRNA, 30S assembly | Suwastika et al. 2014 |
YqeH (AtNOA1) | AT3G47450 | YqeH class GTPase | cGTPase participating in 30S ribosome assembly | Li et al. 2015 |
BPG2 | AT3G57180 | YqeH-type GTPase | Maturation of 16S and 23S rRNA | Komatsu et al. 2010 |
ObgC | AT5G18570 | Obg GTPases | Binds to 23S rRNA, 50S ribosome assembly | Bang et al. 2012 |
RbgA | AT4G02790 | RbgA GTPase | Interacts with the plastid RPs RPL6 and RPL35, GTP hydrolysis promotes its dissociation from the 50S subunit | Seffouh et al. 2019; Jeon et al. 2017 |
EMB2738 (DER) | AT3G12080 | Double Era-like GTPase | Associated with 50S ribosomal, accumulation of ribosomes | Jeon et al. 2014 |
RNA chaperones | ||||
PAC | AT2G48120 | RNA binding | PAC, co-migrates with premature 50S ribosomal particles, binding to and remodeling a specific region of the 23S rRNA | Meurer et al. 1998; Meurer et al. 2017; Tirlapur et al. 1999 |
CFM4 | AT4G39040 | CRM family member subfamily4 | Processing of 16S and 4.5S rRNA | Lee et al. 2014 |
SDP | AT1G12800 | S1 domain–containing RNA-binding protein | Processing of 23S–4.5S | Han et al. 2015 |
SRRP1 | At3g23700 | S1 domain–containing RNA-binding protein | Splicing of trnL intron and cleavage of the 5S rRNA | Gu et al. 2015 |
Other proteins involved in ribogenesis | ||||
RBF1 | AT4G34730 | RBF A domain, interacts with AtRsgA | 3ʹ and 5ʹ end maturation of 16S rRNA | Fristedt et al. 2014 |
SOT1 | AT5G46580 | PPR-SMR protein, endonuclease | Protects 5ʹ 23S-4.5S dicistronic precursor from exonucleolytic activity of RNJ | Zhang and Lu 2019 |
DCL | AT1G45230 | DUF3223 domain | Generating the 4.5S rRNA | Bellaoui et al. 2003 |
RAP | AT2G31890 | OPR protein | 5ʹ processing of 16S and 23S rRNA | Kleinknecht et al. 2014 |
CRASS | AT5G14910 | Putative heavy metal–associated (HMA) domain | Associates with 16S rRNA and RPs of the small 30S subunit | Pulido et al. 2018 |
WCO (WHITECOTYLEDONS) | Genetically maps on chromosome 1 | Not identified yet | Development- and/or cell-specific 16S maturation | Yamamoto et al. 2000 |
PGL12 | AT1G71460 | PLS-type PPR harboring an RRM domain | Assists in the processing of 16S rRNA | Chen et al. 2019 |
TIC56 | AT5G01590 | Protein of the plastid import machinery | Processing of 23S rRNA and 50S ribosome assembly | Köhler et al. 2016 |
CGL20a/b | AT2G17240/AT3G24506 | Prolin-rich ∼10-kD protein | 50S ribosome biogenesis via the formation of a hidden break A in the 23S rRNA | Reiter et al. 2020 |
PPR4 (WSL5) | AT5G04810 | P-type PPR protein carrying an RRM domain | Editing and rps12 intron 1 trans-splicing and, indirectly, in the assembly of 70S ribosomes | Liu et al. 2018; Lee et al. 2019 |
DAL (MORF2) | AT2G33430 | Part of the MORF family | Splicing and editing of transcripts encoding RPs | Takenaka et al. 2012 |
rRNA modifications | ||||
CMAL | AT5G10910 | MraW/RsmH-like methyltransferase | m4Cm methylation of 16S rRNA at position C1352 | Manavski et al. 2021 |
RsmD | At3g28460 | N2-methylguanosine methyltransferase | m2G methylation of 16S rRNA at position C915 | Manavski et al. 2021 |
PFC | AT1G01860 | KsgA and DIM1 homolog | m6A methylation of 16S rRNA at position C1352 | Manavski et al. 2021 |
SVR1 | AT2G39140 | Ψ synthase | Pseudouridylation of rRNAs | Sun et al. 2019b |
Protein . | Arabidopsis identifier . | Activity/interaction/family/domain . | Proposed function in ribogenesis . | References . |
---|---|---|---|---|
Ribonucleases | ||||
PNPase | At3g03710 | 3ʹ-5ʹ exoribonuclease | Maturation of 23S rRNA 3ʹ ends | Yehudai-Resheff et al. 2001 |
RNE | At2g04270 | Endunuclease | Production of the dicistronic 23S-4.5S rRNA precursor | Stoppel et al. 2012 |
RNR1 | At5g02250 | 3ʹ-5ʹ exoribonuclease | 3ʹ trimming of 23S, 16S and 5S rRNAs | Kishine et al. 2004; Bollenbach et al. 2005 |
RNJ | At5g63420 | Endoribonuclease and 5′-to-3′ exoribonuclease | Trimming of 16S and 23S 5ʹ rRNA termini and quality control | Hotto et al. 2020 |
RNC3/4 | At1g55140/At3g13740 | Endonucleases, mini-ribonuclease III | rRNA maturation and intron recycling | Hotto et al. 2015 |
CSP41a/b | AT3G63140/AT1G09340 | Endoribonuclease | Association and stability of 23S and 16S rRNAs; processing of hidden break A | Beligni and Mayfield 2008 |
YbeY | AT2G25870 | Endoribonuclease | Maturation of the 5ʹ and 3ʹ ends of 16S, 23S and 4.5S rRNAs | Liu et al. 2015 |
Helicases | ||||
RH39 | AT4G09730 | DEAD-box RNA helicase, binds to and associates in cleavage of 23S rRNA | Processing of a stem-loop structure of 23S rRNA, introduction of the hidden break B site into the 23S rRNA | Nishimura et al. 2010 |
RH22 | AT1G59990 | DEAD-box RNA helicase, interacts with RPL24 | Rearrange secondary structures and processing of the 23S-4.5S precursor rRNA | Chi et al. 2012 |
RH50 | At3g06980 | DEAD-box RNA helicase, binds to rRNAs | Maturation of the 23S and 4.5S rRNAs | Paieri et al. 2018 |
RH3 | At5g26742 | DEAD-box RNA helicase | Associates with Group II introns and 23S rRNA, chaperone function | Asakura et al. 2012; Gu et al. 2014 |
ISE2 | AT1G7007 | Ski2-like RNA helicase | RNA editing and splicing of mRNAs for RPs, rRNA processing | Bobik et al. 2017 |
PRBP (EMB1513) | AT2G37920 | DEAD-box RNA helicase | Maturation of the 4.5 rRNA | Park et al. 2011 |
RHON1 | AT1G06190 | Rho-like RNA helicase | Guides RNE to specific targets, rRNA processing | Stoppel et al. 2012 |
GTPases | ||||
AtRsgA | AT1G67440 | GTPase A, interacts with RbfA | Maturation of 16S rRNA | Janowski et al. 2018 |
WSL6 (Era-1) | AT5G66470 | Era-type GTPase | Binds to the 3ʹ end region of 16S rRNA, 30S assembly | Suwastika et al. 2014 |
YqeH (AtNOA1) | AT3G47450 | YqeH class GTPase | cGTPase participating in 30S ribosome assembly | Li et al. 2015 |
BPG2 | AT3G57180 | YqeH-type GTPase | Maturation of 16S and 23S rRNA | Komatsu et al. 2010 |
ObgC | AT5G18570 | Obg GTPases | Binds to 23S rRNA, 50S ribosome assembly | Bang et al. 2012 |
RbgA | AT4G02790 | RbgA GTPase | Interacts with the plastid RPs RPL6 and RPL35, GTP hydrolysis promotes its dissociation from the 50S subunit | Seffouh et al. 2019; Jeon et al. 2017 |
EMB2738 (DER) | AT3G12080 | Double Era-like GTPase | Associated with 50S ribosomal, accumulation of ribosomes | Jeon et al. 2014 |
RNA chaperones | ||||
PAC | AT2G48120 | RNA binding | PAC, co-migrates with premature 50S ribosomal particles, binding to and remodeling a specific region of the 23S rRNA | Meurer et al. 1998; Meurer et al. 2017; Tirlapur et al. 1999 |
CFM4 | AT4G39040 | CRM family member subfamily4 | Processing of 16S and 4.5S rRNA | Lee et al. 2014 |
SDP | AT1G12800 | S1 domain–containing RNA-binding protein | Processing of 23S–4.5S | Han et al. 2015 |
SRRP1 | At3g23700 | S1 domain–containing RNA-binding protein | Splicing of trnL intron and cleavage of the 5S rRNA | Gu et al. 2015 |
Other proteins involved in ribogenesis | ||||
RBF1 | AT4G34730 | RBF A domain, interacts with AtRsgA | 3ʹ and 5ʹ end maturation of 16S rRNA | Fristedt et al. 2014 |
SOT1 | AT5G46580 | PPR-SMR protein, endonuclease | Protects 5ʹ 23S-4.5S dicistronic precursor from exonucleolytic activity of RNJ | Zhang and Lu 2019 |
DCL | AT1G45230 | DUF3223 domain | Generating the 4.5S rRNA | Bellaoui et al. 2003 |
RAP | AT2G31890 | OPR protein | 5ʹ processing of 16S and 23S rRNA | Kleinknecht et al. 2014 |
CRASS | AT5G14910 | Putative heavy metal–associated (HMA) domain | Associates with 16S rRNA and RPs of the small 30S subunit | Pulido et al. 2018 |
WCO (WHITECOTYLEDONS) | Genetically maps on chromosome 1 | Not identified yet | Development- and/or cell-specific 16S maturation | Yamamoto et al. 2000 |
PGL12 | AT1G71460 | PLS-type PPR harboring an RRM domain | Assists in the processing of 16S rRNA | Chen et al. 2019 |
TIC56 | AT5G01590 | Protein of the plastid import machinery | Processing of 23S rRNA and 50S ribosome assembly | Köhler et al. 2016 |
CGL20a/b | AT2G17240/AT3G24506 | Prolin-rich ∼10-kD protein | 50S ribosome biogenesis via the formation of a hidden break A in the 23S rRNA | Reiter et al. 2020 |
PPR4 (WSL5) | AT5G04810 | P-type PPR protein carrying an RRM domain | Editing and rps12 intron 1 trans-splicing and, indirectly, in the assembly of 70S ribosomes | Liu et al. 2018; Lee et al. 2019 |
DAL (MORF2) | AT2G33430 | Part of the MORF family | Splicing and editing of transcripts encoding RPs | Takenaka et al. 2012 |
rRNA modifications | ||||
CMAL | AT5G10910 | MraW/RsmH-like methyltransferase | m4Cm methylation of 16S rRNA at position C1352 | Manavski et al. 2021 |
RsmD | At3g28460 | N2-methylguanosine methyltransferase | m2G methylation of 16S rRNA at position C915 | Manavski et al. 2021 |
PFC | AT1G01860 | KsgA and DIM1 homolog | m6A methylation of 16S rRNA at position C1352 | Manavski et al. 2021 |
SVR1 | AT2G39140 | Ψ synthase | Pseudouridylation of rRNAs | Sun et al. 2019b |
The Role of Ribonucleases and RNA-Helicases in rRNA Maturation
The rRNA gene cluster in plastids consists of the four distinct rRNA species separated by the two intron-encoded trnI and trnA tRNA genes. Further downstream is the trnR gene (Fig. 1). To produce mature monocistronic rRNA and tRNA isoforms, the large precursor RNA molecule undergoes multiple processing steps, which involve various RNA-binding proteins, exo- and endonucleases (Stoppel and Meurer 2012, Manavski et al. 2018). In eubacteria, a similar cleavage and trimming of the single rRNA species have been observed, which involves the participation of at least five different RNases and many additional factors (Shajani et al. 2011).
YbeY is an endonuclease that is responsible for processing rRNA, and along with RNase R (RNR), it regulates the quality of translation in bacteria (Jacob et al. 2013). A homolog of the bacterial YbeY has been discovered in Arabidopsis, and studies have shown that it participates in the maturation of the 5ʹ and 3ʹ ends of 16S, 23S and 4.5S rRNAs in chloroplasts (Liu et al. 2015). In Arabidopsis chloroplasts, the large rRNA precursor molecule of 7.4 kb undergoes two endonucleolytic events that separate the 16S rRNA from the dicistronic 23S-4.5S rRNA, generating monocistronic 5S rRNA (Stoppel and Meurer 2012). The maturation of the dicistronic 23S-4.5S rRNA is then facilitated by the pentatricopeptide repeat (PPR) protein of the PPR- Small MutS-Related (SMR) subfamily, SOT1 (SUPPRESSOR OF THYLAKOID FORMATION1), which has been suggested to exhibit endoribonucleolytic activity (Wu et al. 2016, Zhou et al. 2017). Similar to its homolog in maize, called PPR53, SOT1 binds the 5ʹ overhang of the 23S-4.5S dicistronic precursor to protect it from the 5ʹ-to-3ʹ exonucleolytic activity of RNase J (RNJ), thereby allowing the intermolecular RNA base pairing between complementary segments of the 5ʹ and 3ʹ end of 23S and 4.5S rRNA, respectively (Wu et al. 2016, Zoschke et al. 2016, Zhang and Lu 2019). The double-stranded RNA loop that is formed is recognized and cleaved by the mini-endoribonuclease III, resulting in the formation of the mature 5ʹ and 3ʹ ends of 23S and 4.5S rRNAs, respectively (Hotto et al. 2015, 2020) (Fig. 1).
The 16S rRNA undergoes further processing in which approximately 180 nt downstream of its mature 3ʹ end are cleaved off by the endoribonuclease Chloroplast Stem-loop-binding Protein of 41 kDa (CSP41). CSP41b has been observed to associate with pre-ribosomal particles in vivo, likely playing a role in the final stages of 23S rRNA maturation (Beligni and Mayfield 2008). It has also been suggested that CSP41 interacts with ribosome-nascent chain complexes to process and stabilize chloroplast mRNAs (Qi et al. 2012, Króliczewski et al. 2016). The RNA-binding site of the octotricopeptide repeat (OPR) RNA binding domain abundant in apicomplexans (RAP) protein was mapped to the 5ʹ region of the 16S rRNA precursor. RAP was thought to specify the trimming site, similar to the roles played by RNase E (RNE) and RHON1 (Stoppel et al. 2012, Kleinknecht et al. 2014).
RNase-generated cleavage between 16S and tRNAIle
The endoribonuclease RNE in chloroplasts is responsible for the cleavage of different RNA species. It is lacking the C-terminal degradosome scaffold domain, which is found in bacteria but contains the conserved catalytic domain for endonucleolytic cleavage together with an N-terminal extension (Stoppel et al. 2012). Moreover, there is evidence suggesting the involvement of RNE, along with its interaction partner RHON1, in the processing of rRNA. This is supported by reduced levels of rRNA and 50S ribosomal particles observed in rne mutants (Mudd et al. 2008, Walter et al. 2010, Stoppel et al. 2012). RHON1, which is a Rho-like DNA/RNA helicase (RH), harbors a C-terminal helix-extended-helix-structured Rho-N-like domain, which binds RNA targets. Several lines of evidence, such as RNA immunoprecipitation (RIP)-chip and RNA-binding studies, strongly suggest that RHON1 supports RNE functions presumably by conferring RNA sequence specificity to the endonuclease via the Rho-N-like domain (Stoppel et al. 2012). RHON1 has been found to guide RNE to specific targets, such as 16S and 23S rRNAs, thus reinforcing the role of RNE in rRNA processing (Stoppel et al. 2012, Yang et al. 2020). In bacteria, RNE has been identified as a crucial factor in RNA processing, stability and degradation and plays a key role in rRNA maturation (Bandyra et al. 2018). Additionally, Arabidopsis RNE was able to rescue an E. coli rne mutant strain, indicating functional conservation (Mudd et al. 2008). However, it should be noted that the deficiency in ribosome production observed in rne mutants in Arabidopsis could also be partly attributed to impaired maturation of transcripts coding for the essential RPL22 or other RPs (Walter et al. 2010, Stoppel et al. 2012). Late stages of ribosome assembly require the separation of 23S and 4.5S rRNAs from the polycistronic precursor, which involves the processing of 4.5S rRNA, and may require RNE and polynucleotide phosphorylase (PNPase) in subsequent processing steps (Leal-Klevezas et al. 2000).
Chloroplast and eubacterial RNJ enzymes exhibit both 5ʹ → 3ʹ exoribonuclease and endonucleolytic activities. Surprisingly, in RNJ knockdowns chloroplast antisense RNAs accumulated, suggesting that these dysfunctional RNAs generally produced by inefficient termination of transcription by the chloroplast RNA polymerases are normally eliminated by RNJ in order to avoid the formation of antisense RNA duplexes with sense strand transcripts that would prevent their translation (Sharwood et al. 2011).
The exoribonuclease RNR and PNPase have been shown to be involved in the maturation of the 3ʹ ends of precursor rRNA. These proteins have clear homology to bacterial proteins and are essential for the trimming of 16S, 23S and 5S rRNA 3ʹ ends (Bollenbach et al. 2005). Mutants of chloroplast RNR1 exhibit a high chlorophyll fluorescence phenotype, impaired growth and pale leaves and are massively affected in plastid translation, indicating a significant role for RNR1 in proper rRNA maturation (Kishine et al. 2004). RNR in E. coli has been found to harbor an intrinsic RH activity in addition to its S1 domain–mediated exonuclease activity and is important in rRNA quality control together with bacterial PNPase (Cheng and Deutscher 2003, Hossain et al. 2016). In Arabidopsis, PNPase is involved not only in 23S 3ʹ end maturation but also in mRNA processing as well as the decay of tRNAs and introns (Walter et al. 2002, Germain et al. 2011).
Interestingly, knockouts of both exoribonucleases, RNR and PNPase, in Arabidopsis led to embryo lethality, suggesting a cooperative activity (Germain et al. 2012). PNPase and RNE have been found to be important for the production of the dicistronic 23S-4.5S precursor, and mutants of both factors accumulate this unprocessed precursor (Walter et al. 2002). The 16S precursor is about 1.7 kb in length and contains approximately 180 nt at the 3ʹ end. Endonucleolytic cleavage produces the mature form, which is 1.6 kb in length. Multiple processing steps of the 23S rRNA result in seven major transcripts of 3.2, 2.9, 2.4, 1.7, 1.2, 1.0 and 0.5 kb, but the dicistronic 23S-4.5S precursor is present at very low levels (Komatsu et al. 2010, Stoppel and Meurer 2012) (Fig. 1).
RNA-helicases: Processing and unwinding of rRNA structures
The ribosomal RNAs do not only undergo maturation steps by being correctly processed but also possess a compact and intricate structure with many potential base-pairing configurations that require partial unwinding to enable proper fitting into the ribosomal subunit structure and specific interactions with RPs. To fulfill these additional processing and restructuring tasks, several RHs have been described in chloroplasts (Fig. 1 and Table. 1).
RHs are highly conserved RNA-binding proteins found in all three domains of life: Eubacteria, Archaea and Eukarya, as well as in many viruses (Jankowsky and Fairman 2007). These enzymes are capable of adjusting RNA secondary structures and rearranging RNA–protein complexes in an ATP-dependent manner (Jarmoskaite and Russell 2014). Most RHs belong to the helicase superfamily 2 (SF2), which is the most diverse out of the six existing helicase superfamilies (SF1–SF6) (reviewed in Byrd and Raney 2012). The DEAD-box helicases belong to the largest family in SF2 and are characterized by the presence of two RecA-like domains forming a highly conserved helicase core. Within the two domains, 12 conserved motifs have been described to date. The noteworthy Motif II, which inspired the name DEAD-box for this family, contains the Asp(D)-Glu(E)-Ala(A)-Asp(D) amino acid sequence (Linder et al. 1989). All these 12 motifs have important functions and play distinct roles in RNA binding and hydrolysis/binding of ATP. In addition, variable size and sequence of N- or C-terminal regions confer specificity for RNA substrates and other proteins (Linder et al. 1989, Byrd and Raney 2012).
DEAD-box RHs play crucial roles across multiple biological processes, including transcription, mRNA processing, translation initiation, RNA decay and ribosomal biogenesis. Extensive research has been conducted on these helicases, spanning diverse organisms ranging from bacteria to plants (Jarmoskaite and Russell 2011, Nawaz and Kang 2017, Robles and Quesada 2019). The genome of E. coli encodes only five DEAD-box RHs, which play crucial roles in mRNA decay and ribosome assembly (Charollais et al. 2003, 2004, Iost and Dreyfus 2006). The genome of plants and other eukaryotes harbors an impressive higher number of genes encoding DEAD-box RHs, which suggests a multi-tasking role for individual bacterial RHs. In A. thaliana, 58 DEAD-box proteins have been identified to date (Aubourg et al. 1999, Zybailov et al. 2008), 10 of which are predicted and/or have proven to be involved in chloroplast RNA metabolism (Nawaz and Kang 2017). Functional characterization of nucleo-cytoplasmic-localized RHs involved in plant stress response and plant development has been widely explored to date (Guan et al. 2013, Liu et al. 2016, Wang et al. 2016b, Liu and Imai 2018, Lee and Kang 2020). Recent studies, however, have revealed that specific DEAD-box proteins play a crucial role in chloroplast RNA metabolism, predominantly in ribosomal RNA biogenesis and assembly of ribosomal subunits (Nawaz and Kang 2017, Robles and Quesada 2019). Particularly, the expression and/or activities of DEAD-box proteins in chloroplasts are frequently associated with abiotic stress (Owttrim 2006, Vashisht and Tuteja 2006, Kant et al. 2007, Owttrim 2013, Liu et al. 2016, Nawaz and Kang 2017, Lee and Kang 2020). Thus, it seems as if the formation of cold-adapted ribosomes and RNA degradosomes may not only occur in bacteria but also in chloroplasts, demonstrating important roles in unwinding RNA secondary structures that are thermodynamically formed at low temperatures (Prud’homme-Genereux et al. 2004, Stoppel et al. 2012, Owttrim 2013, Aguirre et al. 2017).
Among the chloroplast RHs in Arabidopsis, RH22 and RH39 were the first to be identified. Using yeast two-hybrid analysis and co-immunoprecipitation assays, researches showed that RH22 interacts with the RPL24 protein from the large ribosomal subunit (Chi et al. 2012). In addition, RH22 binds specifically to the 5´end of 23S rRNA and contributes to the initial assembly of the 50S subunit (Chi et al. 2012). In E. coli, a similar complex involving RPL24 and 23S rRNA has been demonstrated with the RNA helicase SrmB (Trubetskoy et al. 2009). The helicase activity of RH22 likely helps rearrange secondary structures of the 23S precursor rRNA, allowing its contact to PRL24. On the other hand, the chloroplast RH39 is implicated in the introduction of the hidden break B into the 23S rRNA (Fig. 1). More specifically, RH39 plays a role in the processing of a stem-loop structure formed in the chloroplast 23S rRNA by binding to the hairpin loop adjacent to the hidden break site. RH39 is thought to remodel the rRNA–RNase complex and/or release proteins bound to RNA to allow proper positioning and functioning of endonucleases (Nishimura et al. 2010). RH39 may also participate in ribosomal biogenesis in association with degradosome/exosome multiprotein complexes, which are commonly reported in association with many DEAD-box proteins in prokaryotes and eukaryotes (Bandyra et al. 2013, Khemici and Linder 2018).
RH50 and RH3 are two additional RHs involved in chloroplast ribosomal biogenesis. It has been found that RH50 is crucial for the efficient translation of plastid proteins and required for the maturation of the 23S and 4.5S rRNAs (Paieri et al. 2018). Loss of RH50 and erythromycin, an inhibitor that binds to the large ribosomal subunit, renders chloroplast protein synthesis sensitive to cold. RH50 associates with intact ribosomes in vivo and RNA-seq unveiled a significant increase in levels of chloroplast 23S-4.5S precursor transcripts in rh50-1 mutant lines. In agreement, the electrophoretic mobility shift assay showed that RH50 binds specifically to these rRNAs. Therefore, RH50 has a crucial role in the maturation of the 23S and 4.5S rRNAs (Paieri et al. 2018). Additionally, RH3 and its co-orthologs have also been characterized in Arabidopsis and Zea mays (Asakura et al. 2012, Lee et al. 2013, Gu et al. 2014). RH3 belongs to a network of splicing factors in the chloroplast. RNAs from intron-containing loci (e.g. rps12, rpl2, trnA and trnI) were associated in vivo with RH3. The genes rrn23, trnI and tnrA are co-transcribed simultaneously with rrn16 in the chloroplast rrn operon (Fig. 2). Indeed, loss of RH3 leads to reduced levels of mature trnA and trnI and increased levels of the unspliced precursor, indicating a reduction in the splicing efficiency of trnI and trnA. ZmRH3 is associated with premature 50S ribosomal subunits containing incompletely processed 23S rRNA. Similarly, the Arabidopsis atrh3 mutant lines produced pale-green plants with defective splicing of several group II introns (Asakura et al. 2012). In particular, the functions of RH3 in chloroplast RNA metabolism were further investigated. It has been demonstrated that AtRH3 may function as an RNA chaperone during chloroplast intron splicing (Gu et al. 2014). In fact, AtRH3 was able to complement the chaperone function in cold-sensitive E. coli BX04 cells that lacked cold-shock RNA chaperones (Bae et al. 2000, Phadtare et al. 2002). Additionally, the ability of RH3 to disrupt secondary structures in RNA/DNA molecules has been confirmed. In bacteria, SrmB is implied to act as an RNA chaperone, facilitating structural RNA rearrangements that support the recruitment of the L13 protein in ribosomal biogenesis (Redder et al. 2015). Similarly, AtRH3 may act in an equivalent way supporting the interaction between RPs and their rRNA targets.
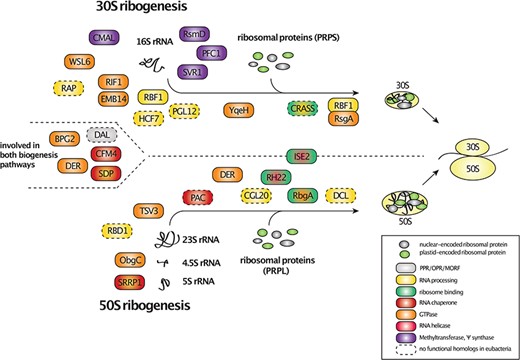
Factors involved in the plastid ribosome assembly. The factors were arranged based on their actions on the small (30S), large (50S) or both ribosomal subunits during the assembly process. The factors were also color-coded based on experimental evidence or known RNA-binding motifs that support their interaction with RPs as well as function in rRNA processing, assembly and RNA chaperone activity (see legend for details). Additionally, GTPases, RNA-helicases and methyltransferases were highlighted in the figure. Factors that lack eubacterial counterparts were framed with dashed lines.
Despite the increasing information on the roles of chloroplast RHs in ribosomal biogenesis, especially in the assembly of the large ribosomal subunit, their association and further functions in the small subunit are poorly characterized. In addition, most of the studies have been performed in the model plant A. thaliana. Recent studies identified two additional chloroplast-localized DEAD-box proteins in crop plants, TCD33 and SIDEAD39. TCD33 was first characterized in rice, and it has been suggested to play a role in chloroplast development and ribosomal biogenesis under cold stress. While Wild Type (WT) plants exhibited normal green coloration at 32°C, tcd33 mutants showed an albino phenotype before the four-leaf stage and died at 20°C. tcd33 mutants displayed reduced chlorophyll contents and abnormal expression of chloroplast-related genes under low temperatures. In addition, low expression of 16S rRNA, 23S rRNA and AtpA in the albino tcd33 plant suggested the involvement of TCD33 in chloroplast ribosome assembly (Xiaomei et al. 2020). SlDEAD39 plays a role similar to its Arabidopsis homolog RH39 (Nishimura et al. 2010). The gene RES (restored cell structure by salinity) is essential in plant stress response, and loss of function leads to delayed development and leaf chlorosis, which are reversed under salt-stress conditions (Garcia-Abellan et al. 2015). Strikingly, map-based cloning demonstrates that RES encodes SlDEAD39 in tomato plants. Functional analysis of mutant lines and in vitro binding assays showed that SlDEAD39 specifically participates in the processing of 23S rRNA at the hidden break B site (Capel et al. 2020). Surprisingly, other nuclear-encoded chloroplast-targeted RHs (SlDEAD5, SlDEAD17 and SlDEAD33) were highly upregulated in different res mutant lines. This could be due to impaired regulation of genes affecting photosynthesis in res mutants, which activates the expression of genes responsible for assisting chloroplast gene expression under hostile conditions. Therefore, the enhancement of photosynthesis as well as the expression of auxiliary RHs possibly have essential roles in the reversion of the phenotypes observed in res mutant lines under salt stress (Capel et al. 2020). Similarly, cross-complementation of functions between two or more RHs is commonly reported in eubacteria. In E. coli, RhlE can associate with RNE and functionally replace RhlB in RNA degradation (Khemici et al. 2004).
Apart from the functions and characteristics already mentioned, a unique factor has been described as having a broad role in plastid RNA metabolism. ISE2 (INCREASED SIZE EXCLUSION LIMIT 2) encodes a Ski2-like RNA helicase identified through genetic screening for mutants with altered plasmodesmata (PD). Loss of ISE2 resulted in structural and functional changes in PD during plant embryogenesis. ise2 mutants produce high numbers of PD with altered branched architecture and allow continued transport of large fluorescent tracers in different stages of embryogenesis in Arabidopsis (Kobayashi et al. 2007). Interestingly, ISE2-GFP is localized in chloroplasts, and alterations in plastid gene expression and levels of photosynthetic pigments have been reported in plants lacking ise2 (Carlotto et al. 2016). Although ISE2 does not belong to the DEAD-box family, it has been recently reported that ISE2—similar to RH3—is involved in the splicing of group II introns in chloroplast transcripts. ISE2 specifically binds to the rpl2, atpF and clpP1 transcripts, and the splicing of all these introns was reduced in ise2 mutants. In addition, ISE2 plays a role in chloroplast rRNA processing. Loss of ISE2 leads to defects in rRNA processing regarding not only large ribosomal subunits but also small subunits, an additional role for chloroplast-localized RHs. In fact, RNA-immunoprecipitation has identified the 23S, 16S, 5S and 4.5S rRNAs in association with ISE2. Interestingly, ISE2 was the first RH reported to be involved in chloroplast RNA editing (Bobik et al. 2017). In plant leaves co-suppressing ISE2, 35% of examined sites displayed altered degrees of RNA editing compared to the WT control. The involvement of ISE2 in RNA editing may be specific to certain sites within transcripts, as distinct sites displayed varying editing patterns in plants lacking ISE2. Recent findings have shown that ISE2 interacts with the RP RPL15 in vivo (Bobik et al. 2019). Similar to ISE2, the loss of uL15c also resulted in abnormal rRNA processing as well as defective chloroplast translation/protein accumulation. In fact, an increase in intercellular trafficking through PD was also reported, providing further indications for a connection between chloroplast functional status and PD activity. No further studies to uncover the functions of chloroplasts associated with PD regulation were reported. Thanks to PD development, substance exchange can take place across the cell boundary, which is vital for the cell survival. In addition to facilitating information exchange between cells, PD serves another function: transporting nutrients produced in the chloroplast to feed the cells (Eom et al. 2012). Presumably, increased PD formation is a mechanism to compensate for the loss of photosynthetic products reflecting a higher demand for exchange and communication.
Silencing of plastid RNA-binding protein (PRBP) expression in tobacco resulted in processing defects of the 4.5S rRNA and drastically reduced amounts of 4.5S and 5S rRNAs in polysomal fractions (Park et al. 2011). The corresponding Arabidopsis protein EMB1513 (AT2G37920) carries an N-terminal region with homology to ATP-dependent RHs, suggesting that PRBP may be another DEAD-box RH important for the maturation of the 4.5 rRNA. In vitro binding assays have also demonstrated that PRBP from Nicotiana benthamiana binds to the 23S-4.5S rRNA precursor, further supporting its role in plastid RNA metabolism (Park et al. 2011). The maturation of rRNA is not complete with the initial cleavage and processing steps. Additional processing and refolding steps are required. This process involves various factors such as GTPases, chaperones and several other proteins, as described in the following paragraphs (Figs. 1–3; Table. 1).
The Ancient Families of GTPases
The importance of GTPases in ribosome assembly has been extensively studied in bacterial systems (Britton 2009). GTPases play a crucial role in supporting the energetically demanding assembly process by providing extra energy through GTP hydrolysis for intramolecular rearrangements or disassembling auxiliary factors after their completion of tasks (Verstraeten et al. 2011). Moreover, GTPases have the capability to connect the translation machinery with the cell cycle and other metabolic processes, thereby linking protein production with various cellular activities (Karbstein 2007).
GTPases involved in 16S rRNA maturation and 30S assembly
Several GTPases implicated in the processing of the chloroplast 16S rRNA also serve as factors for 30S assembly (Fig. 1, Table. 1). AtRsgA, the A. thaliana ribosome small subunit–dependent GTPase A, plays a crucial role in the maturation of 16S rRNA, similar to its bacterial counterpart RsgA. However, AtRsgA only partially rescues the Δrsga E. coli strain, indicating a slightly different and/or additional function (Janowski et al. 2018). Like in E. coli, AtRsgA interacts with RBF1, a homolog of the eubacterial RbfA protein, even though RBF1 was initially identified as part of the GreenCut compilation (proteins only present in Viridiplantae) due to its divergent sequence apart from the RbfA domain and its sequence insertion into the RbfA domain (Fristedt et al. 2014). RBF1 is an essential plastid-localized factor for the 5ʹ end maturation of 16S rRNA and might have gained an additional role in the 3ʹ end maturation of 16S rRNA in plants (Fristedt et al. 2014). In bacteria, RsgA ensures the release of RbfA from the mature 30S ribosome, serving as a quality control step to prevent immature ribosomes from initiating protein synthesis (Goto et al. 2011, Sharma and Woodson 2020). A similar role might be also suggested for the interaction of AtRsgA and RBF1. The rice low-temperature–sensitive wsl6 (white stripe leaf6) mutant had markedly reduced levels of chloroplast rRNAs and amounts of RPs. The corresponding WSL6 protein encodes an Era-type GTPase, which binds to the 3ʹ end region of 16S rRNA in vitro and thus contributes to 30S assembly (Sun et al. 2019a). Similarly, the maize era mutant exhibited a phenotype ranging from virescent to ivory. Both maize and Arabidopsis Era-1 proteins are crucial in the maturation of 16S rRNA and are localized to nucleoids, where they perform their essential functions by binding to the 16S 3ʹ end in a GTP-dependent manner (Majeran et al. 2012, Suwastika et al. 2014, Belcher et al. 2015). Interestingly, a Ras-like small GTPase in E. coli possesses an essential function in cell cycle control and maturation of the 16S rRNA in the 3ʹ end (Ji 2016, Razi et al. 2019).
Another class of GTPases is the so-called YqeH group involved in ribosome assembly. Related GTPases containing circularly permutated G (CPG) motif domains are prevalent in bacteria. The five CPGs (G1–G5), which normally appear in consecutive order, play crucial roles in nucleotide exchange, GTP hydrolysis and conformational changes. Surprisingly, in chloroplast YqeH, the CPGs are rearranged in the linear order of G4-G5-G1-G2-G3, while still allowing the formation of the three-dimensional fold known for canonical GTPases (Sudhamsu et al. 2008). The special arrangement of domains in CPGs allows for a connection between GTP-binding/hydrolysis and RNA-binding and/or protein–protein interactions (Anand et al. 2006). The essential YqeH protein in Bacillus subtilis binds to the 30S subunit in a GTP/GDP-dependent manner during initial assembly stages (Anand et al. 2009). Biochemical analyses could furthermore resolve its unusual GTP hydrolysis mechanism that involves a potassium ion (Anand et al. 2010). The YqeH-type GTPase initially referred to as AtNOA1 (A. thaliana NO-associated protein 1) was later termed AtNOS and thought to function as a nitric-oxide synthase, but in-depth analysis clearly disproved this assumption and substantiated its role as a cGTPase participating in ribosome assembly after successful cross-species complementation of Arabidopsis noa1 with Geobacillus stearothermophilus YqeH (Moreau et al. 2008). The noa1 Arabidopsis mutant appeared again as resistance to inhibition by fosmidomycin (rif1) line in a screen for mutants. This was attributed to the upregulation of two methylerythritol phosphate (MEP) pathway enzymes in the mutant line, which had a secondary effect (Flores-Perez et al. 2008). Quantitative proteomics data of a rice nao1 RNAi line furthermore confirmed the decreased translation of chloroplast-encoded proteins accompanied by a decrease in 16S rRNA levels (Liu et al. 2010). Further studies underlined the importance of rice NOA1 at low temperatures, as the molecular defects in the mutant could be restored to some extent at elevated temperatures (He et al. 2018), a phenomenon that was repeatedly observed for mutants with defects in plastid translation (Ehrnthaler et al. 2014, Xiaomei et al. 2020). Maize EMB14 (EMBRYO DEFECTIVE 14) represents another YqeH class GTPase with in vitro GTP hydrolysis activity and a crucial role in the formation of the 30S ribosomal subunit (Li et al. 2015). In contrast to embryo lethality of emb14 in maize, knockouts of rif1(atnoa1) alleles in Arabidopsis were viable, but leaves appeared pale and growth was retarded. Interestingly, introducing a copy of maize EMB14 could restore the defects of the rif1 Arabidopsis mutant (Li et al. 2015), thus arguing that these two closely related GTPases possess overlapping functions in 30S ribogenesis. Bpg2 (Brz-insensitive pale green 2), also termed yl1 (yellow leaf 1), was identified as a pale-green mutant with reduced sensitivity toward the brassinosteroid biosynthesis inhibitor brassinazole and showed abnormal accumulation of 16S and 23S rRNA precursors (Komatsu et al. 2010). The corresponding BPG2 protein represents a YqeH-type GTPase harboring a zinc finger motif as well as four GTP-binding domains, which are essential for proper protein function (Komatsu et al. 2010). It was demonstrated through co-immunoprecipitation experiments that BPG2 binds specifically to both 16S and 23S ribosomal RNAs, with a higher affinity for the 16S rRNA. As a result, BPG2 is believed to play a significant role in the assembly of the 30S ribosomal subunit (Kim et al. 2012). Previous studies have shown that B. subtilis YqeH, which is homologous to BPG2, is involved in the accumulation of pre-16S rRNA in YqeH-depleted cells (Uicker et al. 2006, Loh et al. 2007). The mature 16S rRNA is produced by endonucleolytic cleavage of the intergenic region of a primary transcript that is around 180 nucleotides downstream of the mature 16S 3ʹ end (Komatsu et al. 2010). These findings suggest that BPG2 has a significant role in the processing and/or maturation of chloroplast 16S and 23S rRNAs.
GTPases involved in 23S rRNA maturation and 50S assembly
Obg proteins, among them ObgC, are GTPases, which are involved in the assembly of the 50S ribosome subunit. ObgC has three significant protein domains: the Obg fold, which is crucial for its association with parts of the 50S ribosomal subunit; the G domain, which possesses GTP hydrolysis activity, and a unique C-terminus. In vivo studies have shown that ObgC binds to 23S rRNA and is essential for 50S ribosome assembly, similar to several eubacterial Obg proteins (Bang et al. 2012). Another mutant, tsv3, was identified in rice lacking a plastid-localized Obg-homologous protein. Under cold stress, tsv3 displayed a massive decrease in 50S ribosome subunits, but a wild-type phenotype was restored under elevated temperatures (Lin et al. 2018). Another GTPase, called RbgA, was discovered in eubacteria, which associates with a 45S intermediate of the 50S ribosomal subunit and stabilizes critical rRNA helices, thereby functioning in a late step of 50S ribosome assembly (Uicker et al. 2006, Seffouh et al. 2019). Furthermore, it has been proposed that RbgA serves as a placeholder and helps in positioning the L16, L27 and L36 ribosomal subunits and that GTP hydrolysis promotes its dissociation from the 50S subunit (Achila et al. 2012, Gulati et al. 2014). In plants, the RbgA homolog co-elutes with 50S ribosomal fractions and interacts with the plastid RPs RPL6 and RPL35, thus playing a critical role in the generation of the large ribosomal subunit (Jeon et al. 2017). Given that Arabidopsis mutants are embryo-lethal, characterization of a tobacco knockdown mutant has revealed lowered levels of 23S and 16S rRNA and impaired plastid translational activity (Jeon et al. 2017). Additionally, tobacco Double Era-like GTPase (DER) is predominantly associated with 50S ribosomal particles in vivo, which depended on the contribution of the two GTPases and its C-terminal domain. However in vitro association with both 23S and 16S rRNA was also observed (Jeon et al. 2014).
Additional Proteins Aiding in rRNA Processing
Many factors not classified as GTPases are involved in the processing of ribosomal RNAs. In rice, the PALE-GREEN LEAF12 (PGL12) gene encodes a canonocal P-type motif and variant long (L)- and short (S)-type motif (PLS)-type PPR protein that assists in the processing of 16S rRNA (Chen et al. 2019). RAP, named for its 3ʹ RNA-binding domain abundant in apicomplexans, was initially identified as important for plant pathogen defense, as the rap mutant in A. thaliana exhibited enhanced resistance against Pseudomonas syringae and displayed growth retardation and chlorosis (Katiyar-Agarwal et al. 2007). RAP represents the only OPR protein found in A. thaliana and was later shown to be required for the maturation of the 16S rRNA by specifically binding to the 5ʹ end of its precursor (Kleinknecht et al. 2014). CRASS (CHLOROPLAST RIBOSOME ASSOCIATED), termed according to its tight co-expression with plastid RPs, was found to co-immunoprecipitate with 16S rRNA and RPs of the small 30S subunit and to be of particular importance as a ribogenesis factor under stressful conditions such as cold (Pulido et al. 2018). The maize mutant hcf7 was characterized in the early nineties, and it exhibited reduced amounts of polysomes and a processing defect of 16S rRNA, which led to incompletely processed 5ʹ and 3ʹ ends, but the corresponding protein has not yet been functionally characterized (Barkan 1993). Still, in-depth analyses of polysomes of the hcf7 mutant clearly proved that immature forms of 16S rRNA are largely excluded from polysomal fractions, thus only mature 16S rRNA functions efficiently in ribosome biogenesis and translation (Barkan 1993). Interestingly, one development-specific protein involved in 16S maturation was discovered as the corresponding Arabidopsis wco (white cotyledon) mutant exhibited maturation defects only in the cotyledon state, but not in true leaves (Yamamoto et al. 2000).
The processing of chloroplast rRNA during 50S subunit biogenesis involves various proteins, including DCL (DEFECTIVE CHLOROPLAST AND LEAF) in tomato, which is essential for generating the 4.5S rRNA (Bellaoui et al. 2003). The Arabidopsis ortholog of DCL (AT1G45230), also known as DUF3223 (Domain of Unknown Function3223), remains poorly understood, and it is unknown how it might interact with RNA. RBD1 (RNA-BINDING DOMAIN 1) represents another thoroughly characterized RNA-binding protein harboring an RNA recognition motif (RRM) domain, which directly binds to 23S rRNA in a temperature-dependent manner and aids in its maturation (Wang et al. 2016a).
The dal mutant (dag-like = differentiation and greening-like) was initially identified in a T-DNA collection (SK1-N2 line), exhibiting an albino phenotype (Babiychuk et al. 1997). Due to the observed accumulation of 16S and 23S rRNA precursors and their inefficient maturation in an allelic mutant line, a function of DAL in rRNA processing was suggested (Bisanz et al. 2003). Closer analysis of the sequence revealed that DAL is part of the MORF family (MORF2, MULTIPLE ORGANELLAR RNA EDITING FACTOR 2) of organellar proteins involved in plastid RNA metabolism with a major role in RNA editing and that knockouts of DAL affect almost all known plastid RNA-editing sites (Takenaka et al. 2012). MORF2 was found to form homo- or heterodimers with MORF8 and MORF9 and to interact with multiple PPR proteins including PDM1 (Pigment-Defective Mutant), PDM2, CLB19 (CHLOROPLAST BIOGENESIS19), CRR28 (CHLORORESPIRATORY REDUCTION 28) and OTP82 (ORGANELLE TRANSCRIPT PROCESSING 82) (Zhang et al. 2014, 2015, Ramos-Vega et al. 2015, Zehrmann et al. 2015, Du et al. 2017, Huang et al. 2019). Unfortunately, the recent studies could neither reveal a detailed explanation for the observed rRNA defect nor exclude that impaired translation arises due to editing defects in genes coding for ribosomal subunits (RPS14, RPS12 and RPL23) (Takenaka et al. 2012). Similarly, secondary effects on rRNA levels were observed in mutants of the chloroplast moonlighting UMP kinase PUMPKIN, which associates with intron-containing mRNAs and tRNAs and assists in their splicing (Schmid et al. 2019). Thus, it is possible that MORFs may attract additional proteins involved in the processing of rRNAs. Surprisingly, a component of the plastid protein import machinery, Tic56, was identified to play a role in the processing of 23S rRNA and 50S ribosome assembly as well, independent of its function in protein import, which was further supported by the correlation between the expression of Tic56 and nucleases/RNA-helicases involved in rRNA maturation (Köhler et al. 2016). CGL20 (CONSERVED ONLY IN THE GREEN LINEAGE20) is a recently identified factor implicated in 50S ribosome biogenesis. This small protein is encoded by two closely related genes and is known to be dual-localized to both chloroplasts and mitochondria (Reiter et al. 2020). The cgl20a/cgl20b double-knockout mutant displayed a virescent phenotype and defective plastid translation and exhibited impaired hidden break A formation in the 23S rRNA, arguing for a role of the CGL20 protein in late processing steps during the 50S assembly (Reiter et al. 2020). However, an association of CGL20 with ribosomal components has not been investigated.
WSL5 in rice is homolog to PPR4 in Arabidopsis and encodes a chloroplast P-type PPR protein with an N-terminal RNA recognition motif. The rice mutant wsl5 develops white-striped leaves and becomes albino in low temperatures, whereas atppr4 mutants are lethal (Liu et al. 2018, Lee et al. 2019). The failure to efficiently trans-splice and edit mRNAs encoding RPs may lead to chloroplast failure to form functional ribosomes in the cold (Liu et al. 2018). All these findings highlight that the process of cold acclimation predominantly occurs on multiple levels of mRNA and rRNA processing within the chloroplast.
Chloroplast RNA Chaperones
In contrast to RHs, chaperones are capable to resolve or prevent unfavorable RNA structures without the need for additional energy supply by ATP hydrolysis (Russell 2008). Only a few RNA chaperones have been identified in chloroplasts so far (Choi et al. 2015, Jiang et al. 2019, Carius et al. 2022). The pac (pale cress) mutant was described in a collection of T-DNA mutants with pale leaves and defects in chloroplast development (Reiter et al. 1994). Further analysis pointed to a chloroplast localization of the plant-specific Pale Cress (PAC) protein and uncovered a glutamic acid–rich stretch within its protein sequence (Grevelding et al. 1996, Meurer et al. 1998). The analysis of chloroplast transcripts in the pac mutant revealed massive defects in their maturation pattern and abundance arguing for a role of PAC in plastid RNA metabolism (Meurer et al. 1998). Similarities of these transcript patterns to lincomycin-treated plants, co-migration experiments and RIP-seq analyses could finally prove that PAC acts in plastid translation where it co-migrates with premature 50S ribosomal particles and is thus involved in early steps of ribosome assembly. Dimethyl sulfate-probing, a technique used to identify variations in RNA structure, provided evidence that PAC acts as an RNA chaperone by binding to and remodeling a specific region of the 23S rRNA (Meurer et al. 2017).
CRM family member subfamily4 (CFM4), an RNA-binding protein, was found to be important for the correct processing of 16S and 4.5S rRNAs and to play a role in growth and stress response in A. thaliana (Lee et al. 2014). Cross-species complementation of an RNA-chaperone-deficient E. coli strain and transcription anti-termination assays demonstrated that CFM4 possesses RNA chaperone activity in vivo although a direct restructuring activity on its rRNA targets was not investigated (Lee et al. 2014).
The nuclear-encoded S1 domain–containing RNA-binding protein, designated SDP, is localized to chloroplasts and important for growth and development since sdp mutants showed impaired chloroplast biogenesis and photosynthetic activity (Han et al. 2015). The 3.2 kb 23S–4.5S processing intermediate of the rRNA operon strongly accumulated in sdp mutants, indicating that the binding of SDP to rRNA facilitates the processing of this transcript. As a result, several smaller processed rRNA intermediates were found to be decreased in sdp. Although SDP binds to several rRNA species, the binding appears to be nonspecific, which suggests the possibility of additional, as yet unidentified, targets. Several lines of evidence indicated that SDP possesses unspecific RNA chaperone activity. Viable knockout mutants of SRRP1, another S1 domain–containing protein in the chloroplast, failed to splice the trnL intron and to cleave the 5S rRNA. Expression of SRRP1 in RNA chaperone–deficient E. coli cells complemented the growth phenotype at low temperatures, demonstrating RNA chaperone function (Gu et al. 2015). In addition to SDP and SRRP1, the chloroplast petB/petD Stabilizing Factor (BSF) protein also contains an S1 domain and possesses RNA chaperone activity (Jiang et al. 2019). However, BSF, in conjunction AtprfB3, has specific effects on RNA stability and translation of transcripts encoding Cytb6 and Cytf proteins (Stoppel et al. 2011, Jiang et al. 2019). Thus, the RNA chaperone function of the S1 domain has been recruited several times during endosymbiosis to specifically act on chloroplast transcripts.
Interestingly, approximately one-third of all RPs in the 50S subunit have been described to possess RNA chaperone activity in E. coli, with the evolutionary conserved L1 subunit displaying the highest activity (Semrad et al. 2004, Ameres et al. 2007). This may explain why ribosomes can self-assemble in vitro in the absence of auxiliary ribogenesis factors under certain experimental conditions such as various temperatures and ionic strength (Traub and Nomura 1968, Nierhaus and Dohme 1974). Since many ribosomal subunits are conserved between eubacteria and chloroplasts, it is possible that similar chaperone functions may also apply to plastid RPs.
Assembly Factors Interacting with Ribosomal Subunits
There is currently limited experimental evidence on chloroplast ribogenesis factors that directly interact with specific RPs (Fig. 2). To date, only a few factors, including the RNA-helicases ISE2 and RH22, the GTPase RbgA and CRASS, have been reported to interact with PRPL15, PRPL24, PRPL6/PRPL35 or PRPS1/PRPS5, respectively (Chi et al. 2012, Jeon et al. 2017, Pulido et al. 2018, Bobik et al. 2019). However, it is worth noting that searches for interaction partners of RPs have only been conducted for a few known ribosome assembly factors. Thus, further detailed analyses will likely uncover additional factors that interact with RPs and help to categorize them into different phases of ribogenesis.
New Insights into Environmental Regulation of Ribosome Assembly
Previous investigations have demonstrated that rRNA processing is influenced by developmental stages, as well as various stress conditions such as cold or heat stress. Since chloroplast translation efficiency is important for plant acclimation to cold or heat, not only mutations in ribogenesis but also many mutants of non-essential chloroplast ribosomal subunits, such as rps1, rps5, rps6, rps15, rps17 and rpl13, display minimal deficiencies or none at all when grown in normal conditions. However, these mutations exhibit severe deficiencies when subjected to cold or heat stress (Zoschke and Bock 2018, Gao et al. 2022, Robles and Quesada 2022).
A temperature-sensitive phenotype of the maize hcf7 mutant, which is impaired in 16S maturation, was reported already many years ago by Barkan (1993). Mutants exhibiting a cold-sensitive phenotype have also been observed for genes affecting ribogenesis such as wsl6, atrsgA, rh3, noa1/rif1, pgl12 or cgl20, among others (Gu et al. 2014, He et al. 2018, Janowski et al. 2018, Chen et al. 2019, Sun et al. 2019a, Reiter et al. 2020). It is worth noting that certain mutants such as rbd1, crass or rh50 did not exhibit severe phenotypes under normal temperatures. However, they displayed pale leaves and reduced growth under cold conditions, indicating that certain auxiliary factors involved in ribosome biogenesis are specifically necessary in low-temperature environments (Wang et al. 2016a, Paieri et al. 2018, Pulido et al. 2018).
This phenomenon could not only be found in Arabidopsis but also found in crops such as rice (Wang et al. 2022, Lan et al. 2023). Thus, the adjustment of ribosome biogenesis seems to represent a conserved mechanism to adapt to low temperatures in both monocots and dicots. Particularly, low temperatures influence RNA structures and provoke unfavorable conformational states of rRNA, which impedes the correct restructuring during the assembly process (Wu and D’Souza 2020). This notion is further supported by the fact that several investigations report at least partial rescue of mutants impaired in chloroplast translation, including mutants with defects in ribogenesis, when grown under elevated temperatures (Ehrnthaler et al. 2014, He et al. 2018, Lin et al. 2018, Xiaomei et al. 2020). This clearly demonstrates that additional energy in the form of heat is beneficial for the efficiency of plastid ribosome biogenesis and protein production, most probably by relaxing tight RNA structures. It was demonstrated that defects in 23S maturation occurred early in the rbd1 mutant during cold treatment in comparison with defects in other transcript patterns (Wang et al. 2016a).
Cold-sensitive phenotypes of mutants impaired in ribogenesis are a common phenomenon in eubacterial systems and in eukaryotes as well, underlining the common features and conserved principles of rRNA processing, folding and assembly (Falke and Wright 1975, Charollais et al. 2003, 2004, Connolly et al. 2008). As sessile organisms, plants rely even more on regulatory proteins that can sense and respond to external signals to adjust cellular processes. Thus, it is not surprising that ribogenesis factors were reported to respond to different types of external stimuli and stresses ranging from changing temperatures to salt, dehydration or light intensity. Plastid-localized RHs have been shown to be involved in abiotic stress responses, as their expression was highly responsive to various abiotic stimuli (Nawaz and Kang 2017). Detailed analyses of RH3 and RH50 confirmed this assumption as both proteins are particularly important under cold conditions and RH3 was furthermore reported to be involved in maintaining ABA levels (Lee et al. 2013, Nawaz and Kang 2017). The expression of several ribosome biogenesis factors has been shown to be regulated by light, including the RNA chaperone PAC, the GTPases RbgA and BPG2 (Reiter et al. 1994, Kim et al. 2012, Jeon et al. 2017). Additionally, BPG2 expression was also responsive to brassinosteroid biosynthesis as well as to salt stress (Komatsu et al. 2010, Li et al. 2016). Similarly, cfm4 mutants displayed an increased sensitivity to salt and cold stress (Lee et al. 2014). It has also been observed that chloroplast ObgC possesses a threonyl-tRNA synthetase-GTPase-SpoT proteins TGS domain, which is not present in mitochondrial ObG proteins and has been implicated in stress response mechanisms in bacteria (Suwastika et al. 2014). Even though experimental data are missing, the existence of this domain might argue for a special role of this protein in chloroplast stress response as well. Last but not least, the exoribonuclease PNPase, which is important for 23S 3ʹ processing, was reported to be involved in the acclimation of plants to phosphor availability, while the activity of the endonuclease AtYbeY could be altered by metal ion concentrations due to its metal-binding H3xH5xH motif (Marchive et al. 2009, Liu et al. 2015). In summary, plants are able to finely regulate their complex rRNA metabolism and coordinate the function of their organelles by using regulators of plastid translation, allowing them to acclimate to different environmental cues and stresses, as well as to optimize their growth and development.
The Evolution of Plastid Ribosome Assembly Factors
As the chloroplast originated from an endosymbiotic event with a cyanobacterium, many of the factors involved in chloroplast function and biogenesis have eubacterial origins (Figs. 1 and 2). It is important to note that only a few factors involved in plastid RNA processing can be traced back to the cyanobacterial ancestor or even algae, as the plastid RNA metabolism is a fast-evolving process (Manavski et al. 2012, Barkan 2011, Greiner et al. 2011, Manavski et al. 2018). Diversification of genes encoding components of the ribosomes including rRNAs during plastome-genome co-evolution established species-specific nucleo-organelle interactions. This potentially results in plastome-genome incompatibilities in genetic hybrids and is often phenotypically recognized as inter-lineage hybrid breakdown, bleaching, variegation or disruptions in the sexual phase. Thus, it is likely that the evolution of ribosomal components substantially contributed to speciation processes (Greiner et al. 2011). Moreover, some of these factors may have been acquired through lateral gene transfer events or may have originated from de novo synthesis. Hence, it is important to be cautious while tracing the origins of chloroplast factors in order to ensure accuracy in evolutionary studies. Analysis on the origin of GTPases revealed, for instance, that the previously described Arabidopsis ObgC protein is indeed closely related to cyanobacterial proteins and thus most likely derived from the endosymbiotic precursor of chloroplasts, while the Era-type GTPase, including WSL6 in rice, clustered with Bacteriodes and green sulfur bacteria thus originating from lateral gene transfer events (Suwastika et al. 2014). Likewise, RHs are widely distributed in eubacteria, and many of them were reported to be involved in ribogenesis, but chloroplast-targeted RNA-helicases, such as RH50 or RH39, often lack bacterial orthologs.
Still, in many cases, homologs of eubacterial ribosome assembly factors even fulfill very similar tasks during rRNA processing and ribosome assembly. However, as pointed out in the Introduction, chloroplast ribosomes exhibit several differences in comparison to their eubacterial counterpart, such as a different overall composition and shape as well as positioning of rRNA and proteins within the ribosomal subunits. Thus, several ribogenesis factors adopted and gained a certain degree of specialization in planta. Such adjustments include the gain or loss of specific functions, changes in crucial protein domain sequences and an increased importance for the organism as corresponding plant knockouts are often lethal in contrast to the defects observed in eubacteria. AtRsgA harbors a plant-specific stretch within its GTPase domain. Additionally, its importance has increased in the course of endosymbiosis. While the E. coli mutant strain ΔrsgA exhibits a slow growth phenotype, homozygous knockout mutants of Arabidopsis AtRsgA are embryo-lethal.
It has been suggested that the Arabidopsis RH22 originated from the eubacterial SrmB protein (Chi et al. 2012). RH22 functions in the processing of 23S-4.5S RNA and interacts with RPL24, but the E. coli DEAD-box RH SrmB is primarily involved in the assembly of the 50S ribosome. This observation reflects evolutionary divergence and supports the idea of co-evolution between DEAD RHs and rRNA structure (Chi et al. 2012). Intriguingly, several identified chloroplast ribogenesis factors are not related to any eubacterial progenitors but newly evolved in the plant lineage. While factors such as CGL20 are found in the green-lineage, proteins like PAC and CRASS are only present in embryophytes. In particular, some chloroplast ribogenesis factors such as PPR proteins evolved later during the co-evolution of the nuclear and chloroplast genomes and expanded predominantly in vascular plants (Barkan and Small 2014). While the majority of ribosome assembly factors exhibit conserved functions in both monocots and dicots, there have also been reports of divergent functions between the two groups. One example is the PPR protein PGR3 whose function in plastid ribosome biogenesis was only found in maize but not in Arabidopsis (Cai et al. 2011, Belcher et al. 2015). Moreover, in spite of the conserved function, the phenotypes resulting from the loss of the orthologous chloroplast ribogenesis factors can differ substantially between monocots and dicots: in Arabidopsis, the complete absence of these factors often leads to an embryo-lethal phenotype, whereas in maize, such as in the case of the RH3 protein, it results in albinotic mutants and seedling lethality (Asakura et al. 2012). This difference might be due to species-specific requirements for plastid translation during embryogenesis or the capability to bypass the deficiencies (Bryant et al. 2011). This again underlines the necessity of additional proteins to aid in rRNA maturation and ribosome assembly in distinct species. Such evolutionary pressure might have been caused by changing environmental conditions when conquering land, such as drastic changes in temperature, light intensity, and quality, a different composition of the ribosomes that contain five plant-specific subunits and the regulation of the coordinated assembly of plastid- and nucleus-encoded ribosomal subunits.
Epitranscriptomic modifications of rRNAs
Additionally, rRNA modifications, such as the frequent m6A modification, m5C, m4Cm, m2G, dimethylations, ribose methylation and pseudouridylation, are relevant for ribosome interactions, structure and activity and, thus, for the post-transcriptional regulation of chloroplast gene expression and its role in controlling plant development, stress reactions and acclimation processes (Manavski et al. 2021). Even though a plethora of RNA methylations occur in chloroplasts, only the three RNA methyltransferases CMAL, PFC1 and RsmD, also known as writers, have been identified (Wang et al. 2017, Zou et al. 2020, Manavski et al. 2021) (Fig. 1). Surprisingly, no erasers or readers for chloroplast RNA methylations have been described so far (Manavski et al. 2021). The investigation of ribosome biogenesis is further complicated by the discovery of the dual functionality of the rRNA methyltransferase RsmC, which can also function as an RNA chaperone (Gc et al. 2020). In the future, many more plastid and cytoplasmic rRNA-modifying factors await their discovery and functional thorough characterization, especially under cold acclimation conditions (Manavski et al. 2021, Vicente et al. 2023).
Pseudouridine (ribosyluracil; Ψ) is the most prevalent RNA nucleoside modification commonly found in conserved positions of non-coding RNAs and also plays important roles at lower temperatures and plant sensitivity to Pi starvation in chloroplasts (Manavski et al. 2021). It plays a role in ribosome biogenesis and function often in response to various environmental stimuli (Charette and Gray 2000). In chloroplasts, the Ψ synthase SVR1 (At2g39140) is responsible for installing 11 out of the 15 Ψ sites in rRNAs and is important for ribosome assembly and translation (Sun et al. 2019b). Thus, SVR1 represents not the sole active Ψ synthase in chloroplasts.
Obstacles in Finding and Defining Chloroplast Ribogenesis Factors
One approach that shows promise is comparing the phenotype of mutants that are suspected to have impaired ribosome biogenesis. The absence of most factors participating in rRNA processing and ribosome assembly leads to severe mutant phenotypes, which are even embryo- or seedling-lethal, thus underlining the importance of a guided and coordinated sequence of assembly steps. Often, the analysis of weaker alleles provides further insight into the molecular consequences of reduced amounts of these ribogenesis factors (Meurer et al. 2017). Commonly observed pleiotropic defects in mutants include retarded growth and development as well as reduced photosynthesis and plastid translation. In many cases, regardless of the mutational background, impaired translation was associated with a downregulation of transcripts preferentially generated by the plastid-encoded polymerase (PEP), while transcripts generated by the nuclear-encoded polymerase (NEP) were upregulated (Cho et al. 2009, Wang et al. 2016a). Similar down- and upregulation patterns have been consistently observed in other mutants affected by chloroplast functions, such as those lacking PEP subunits or tRNAs. This further underscores the downstream effects resulting from impaired plastid translation or function. These phenotypes also strongly resemble pleiotropic mutants that lack essential subunits of the plastid ribosomes, which are lethal as well. Thus, abnormal patterns and abundance of 16S and 23S transcripts are a widespread phenomenon of mutants impaired in plastid translation, transcription and/or general plastid development and not necessarily of mutants primarily affected in rRNA processing. Mutants with defects in chloroplast development and plastid gene expression often display pleiotropic defects, which lead to disturbances in plastid translation. As a result, it is quite difficult to differentiate between factors that are directly implicated in ribosome biogenesis and those that are involved in other aspects of plastid gene expression (e.g. Meurer et al. 2017, DeTar et al. 2021, Chen et al. 2022). Taken together, one might speculate that the correct maturation and structuring of the ribosomal RNA is a prerequisite for the following steps, while proteins that aid in later steps and interact with RPs are less essential or that in some cases factors possess overlapping functions.
Future Perspective—Lessons to Be Learned from Other Species and New Technologies
In comparison to eubacterial ribosome assembly, detailed analyses of assembly intermediates or networks remain elusive for chloroplast ribosome biogenesis. Thus, it is reasonable to assume that similar mechanisms will be identified in chloroplasts as well. The study of ribonucleoprotein complexes in plant chloroplasts, particularly those that work in tandem to facilitate proper RNA folding, remains largely unexplored (Bohnsack and Bohnsack 2019). Despite the recent identification of numerous new plastid factors involved in rRNA maturation and ribosome assembly, it often remains uncertain at which stage of ribosome assembly they function. Regrettably, critical information is still missing regarding the sequence of events in which the various rRNA molecules and numerous RPs are incrementally assembled into functional complexes in plastids (Fig. 3). In bacteria, the assembly process may not always follow a linear path, and multiple pathways may occur concurrently, resulting in a diverse array of assembly intermediates, which complicates the molecular analysis of ribosome biogenesis (Shajani et al. 2011). Established techniques from other organisms are promising tools to be transferred to chloroplast research. Especially, the isolation of subunit precursors and their proteomic analysis have been successfully performed in yeast (Fromont-Racine et al. 2003). This method would further contribute to the identification and validation of auxiliary factors aiding in ribogenesis. Many of the studies discussed here did not include experiments to examine protein–protein interactions between assembly factors and RPs. Consequently, the overview of auxiliary factors and their characteristics depicted in Figs. 1–3 may be subject to alteration with the incorporation of additional analyses, such as co-immunoprecipitation, to determine which of these factors may also interact with specific RPs. Interactions between rRNA-cleaving enzymes and RNA-helicases were suggested, which would explain how the nonspecific exoribonuclease might gain a certain degree of specificity (Bollenbach et al. 2005). Such a guidance role was already proven for the RNA-binding protein RHON1, which supports the function of RNE in rRNA processing and other activities (Stoppel et al. 2012). Similarly, the rRNA-binding mitochondrial Transcription Termination Factor (mTERF) protein interacts with ribosome biogenesis factors and CPN60 to promote ribosome assembly (Méteignier et al. 2021).
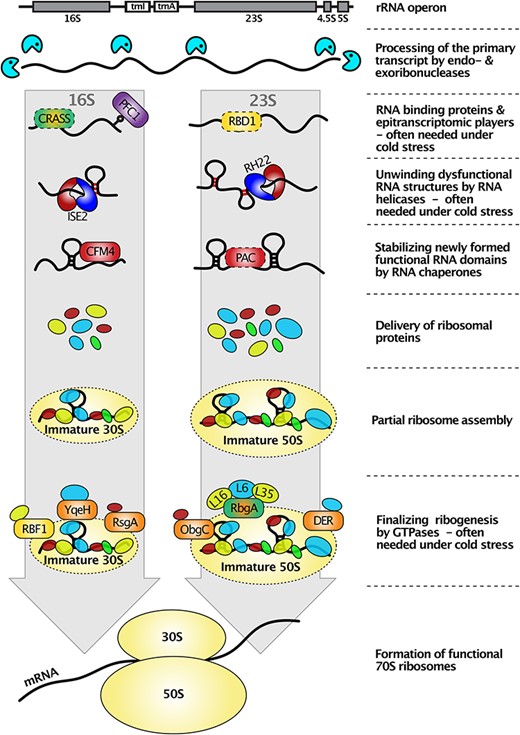
Chloroplast ribogenesis: from transcripts to functional ribosomes. The entire biogenesis pathway is schematically illustrated from rRNA transcripts to the assembly of functional chloroplast 70S ribosomes. Some factors involved in ribogenesis are exemplified. The initial processing of the primary polycistronic rRNA transcript is mediated by exo- and endoribonucleases as well as numerous RNA-binding proteins that facilitate further rRNA maturation steps. Methyltransferases and other writers install epitranscriptomic marks into rRNA species to support the processing, assembly and function of ribosomes. DEAD-box RNA-helicases, which generally contain two RecA-like domains, are often important under abiotic stress conditions to unwind dysfunctional RNA structures (red duplexes) that are thermodynamically trapped especially in the cold. This process typically requires energy in the form of GTP or ATP hydrolysis or even heat. RNA chaperones enable the correct folding of functional rRNAs (black duplexes) without requiring further energy input. Subsequently, RPs are delivered, which partially assemble into the immature ribosomal 30S and 50S subunits presumably due to their own RNA chaperone activity. This process is supported by GTPases, which can serve as placeholders and facilitate the incorporation of additional proteins into the ribosomal subunits. Finally, both subunits come together to form translational competent 70S ribosomes. Note that the coordinated sequence of rRNA maturation and ribosome assembly events does not strictly follow the order shown in the simplified figure, that temporal aspects have not been considered and that the contribution of the 4.5S and 5S rRNAs has been excluded.
Advanced mass spectrometry techniques can provide comprehensive profiling of RPs and their assembly intermediates (Williamson 2008, Liu et al. 2022). These approaches also allow for the identification of post-translational modifications that may be involved in the assembly process. Additionally, chemical crosslinking coupled with mass spectrometry may enable the identification of protein–protein interactions and the mapping of protein contacts within ribosome assembly intermediates (Leitner et al. 2016). Furthermore, it can be used to investigate the dynamic changes in protein composition during ribosome assembly. Moreover, single-particle cryogenic Electron Microscopy (cryo-EM) or fluorescence microscopy provides detailed structural insights into the assembly process and allows for the characterization of intermediate states that may be difficult to capture using traditional cryo-EM methods (Knoops et al. 2012, Duss et al. 2019, Khawaja et al. 2023).
Since numerous rRNA modifications play crucial roles in ribosome assembly, rRNA processing and translational fidelity, nanopore direct RNA sequencing is a relatively new powerful technology for the identification of covalent modifications, providing valuable insights into the dynamic and diverse landscape of rRNA epigenetics (Reddy et al. 2020, Manavski et al. 2021). Nanopore sequencing has already provided valuable insights into the prevalence and dynamics of various RNA modifications, such as m6A, m5C, pseudouridine and many others (White and Hesselberth 2022).
In the future, further research on chloroplast ribosome assembly and its implications for plant survival, acclimation and adaptation holds great potential. The evolutionary origins of chloroplasts and their relationship with bacterial ancestors provide a promising path for further investigations. Studying the specific mechanisms by which newly evolved plastid factors involved in ribosome assembly enable in coping with environmental changes and internal signals can uncover important aspects in plant acclimation, cellular specificity and development. Additionally, understanding the interplay between ancient proteins and newly evolved factors in ribosome assembly could reveal the dynamics of this crucial and complex process during endosymbiosis. Investigations of the intricate molecular processes involved in chloroplast ribosome assembly can yield valuable knowledge with practical implications for enhancing plant resilience and improving agricultural practices under changing environmental conditions.
Data Availability
No new datasets were generated or analyzed in this study.
Funding
National Key Research and Development Program (grant number 2022YFF1001700 to W.C.); German Science Foundation (grant numbers ME 1794/10 and TRR 175 A03 to J.M.)
Acknowledgments
We thank Alexandre Magno Vicente for helpful comments on the manuscript.
Author Contributions
L.-M.S. wrote the first draft. N.M., W.C. and J.M. critically reviewed the draft. J.M. wrote, reviewed and edited the final version of the manuscript. All authors contributed to drafting the work, revised the final manuscript and approved submission.
Disclosures
The authors have no conflicts of interest to declare.
References
Author notes
Present address: Loewe Biochemica GmbH, Mühlweg 2a, Sauerlach 82054, Germany.