-
PDF
- Split View
-
Views
-
Cite
Cite
Haruka Oshikiri, Hao Li, Misaki Manabe, Hirobumi Yamamoto, Kazufumi Yazaki, Kojiro Takanashi, Comparative Analysis of Shikonin and Alkannin Acyltransferases Reveals Their Functional Conservation in Boraginaceae, Plant and Cell Physiology, Volume 65, Issue 3, March 2024, Pages 362–371, https://doi.org/10.1093/pcp/pcad158
- Share Icon Share
Abstract
Shikonin and its enantiomer, alkannin, are bioactive naphthoquinones produced in several plants of the family Boraginaceae. The structures of these acylated derivatives, which have various short-chain acyl moieties, differ among plant species. The acylation of shikonin and alkannin in Lithospermum erythrorhizon was previously reported to be catalyzed by two enantioselective BAHD acyltransferases, shikonin O-acyltransferase (LeSAT1) and alkannin O-acyltransferase (LeAAT1). However, the mechanisms by which various shikonin and alkannin derivatives are produced in Boraginaceae plants remain to be determined. In the present study, evaluation of six Boraginaceae plants identified 23 homologs of LeSAT1 and LeAAT1, with 15 of these enzymes found to catalyze the acylation of shikonin or alkannin, utilizing acetyl-CoA, isobutyryl-CoA or isovaleryl-CoA as an acyl donor. Analyses of substrate specificities of these enzymes for both acyl donors and acyl acceptors and determination of their subcellular localization using Nicotiana benthamiana revealed a distinct functional differentiation of BAHD acyltransferases in Boraginaceae plants. Gene expression of these acyltransferases correlated with the enantiomeric ratio of produced shikonin/alkannin derivatives in L. erythrorhizon and Echium plantagineum. These enzymes showed conserved substrate specificities for acyl donors among plant species, indicating that the diversity in acyl moieties of shikonin/alkannin derivatives involved factors other than the differentiation of acyltransferases. These findings provide insight into the chemical diversification and evolutionary processes of shikonin/alkannin derivatives.
Introduction
Plants produce a variety of specialized metabolites to protect themselves from pathogens and herbivores and to adapt to harsh environments such as low temperatures, drought and UV irradiation. The number of specialized metabolites has been estimated to range from 200,000 to 1,000,000 (Dixon and Strack 2003, Afendi et al. 2012, Rai et al. 2017), with their production in plant species catalyzed by modifying enzymes, including glycosyltransferases, methyltransferases and acyltransferases (Wang et al. 2019a). For example, glycosyltransferases catalyze the glycosylation of metabolites, altering their stability, coloration, hydrophilicity and biological activity (Yonekura-Sakakibara et al. 2008, Zhao et al. 2014). Mutations in glycosyltransferases alter the structure, number and position of sugar moieties, resulting in the diversification of glycosylated metabolites (Yonekura-Sakakibara and Hanada 2011, Peng et al. 2017). BAHD acyltransferases also contribute to the generation of specialized metabolites in plants by catalyzing acylation using short- to medium-chain fatty acyl-CoA as acyl donors. The differences in regiospecificity and acyl-CoA preferences among plant species were found to result in the production of various acylated metabolites with different biological activities (D’Auria 2006, Fan et al. 2019, Schenck et al. 2022).
Specialized metabolites produced by plants in the genera Lithospermum, Arnebia, Echium, Alkanna and Onosma, which belong to the family Boraginaceae, include shikonin/alkannin derivatives, which are present as red naphthoquinone pigments in the roots of these plants. These roots have long been utilized as traditional medicines, with shikonin/alkannin and their fatty acid esters later shown to possess various biological activities such as anti-inflammatory, antimicrobial and anticancer activities (Yazaki 2017, Yadav et al. 2022). The pathways responsible for the biosynthesis of shikonin/alkannin derivatives have been partially identified. The initial step is mediated by the enzyme p-hydroxybenzoic acid geranyltransferase, which catalyzes the coupling of p-hydroxybenzoic acid and geranyl diphosphate, derived from the shikimate and mevalonate pathways, respectively, to produce m-geranyl-p-hydroxybenzoic acid (Supplementary Fig. S1) (Yazaki et al. 2002). Two types of cytochrome P450 enzymes, geranylhydroquinone hydroxylases and deoxyshikonin hydroxylases (DSHs), have recently been shown to be involved in the biosynthesis of intermediates in the shikonin/alkannin biosynthetic pathway (Wang et al. 2019b, Song et al. 2021). In addition, two BAHD acyltransferases, LeSAT1 and LeAAT1, were shown to mediate the enantiomer-specific acylation of shikonin and alkannin in Lithospermum erythrorhizon (Takanashi et al. 2019, Oshikiri et al. 2020). Because shikonin/alkannin derivatives are mainly detected as acylated forms, these two BAHD acyltransferases likely catalyze the last step in the shikonin/alkannin biosynthetic pathway.
The composition of shikonin/alkannin derivatives differs among plant species. Intact roots of L. erythrorhizon mainly produce acetylshikonin and β-hydroxyisovalerylshikonin, roots of Arnebia euchroma and Alkanna tinctoria produce high amounts of β,β-dimethylacrylalkannin and roots of Echium italicum produce angelylshikonin and acetylshikonin (Assimopoulou et al. 2006, Albreht et al. 2009, Zhou et al. 2011). In addition, various shikonin/alkannin derivatives that differ in the length and structure of their aliphatic acyl moieties have been isolated from Boraginaceae plants (Papageorgiou et al. 1999), with these differences in acyl chains affecting the physiological activities of these derivatives (Zhao et al. 2015, Sut et al. 2017, Skoneczny et al. 2019, Fazal et al. 2021). The abundance of acetylated shikonin/alkannin derivatives in the roots of L. erythrorhizon likely reflects the catalytic efficiency for acetyl-CoA of LeSAT1 and LeAAT1 (Oshikiri et al. 2020). However, the mechanisms by which other Boraginaceae species produce various shikonin/alkannin derivatives remain unclear.
The present study was performed to identify homologs of LeSAT1 and LeAAT1 in six species of Boraginaceae, including L. erythrorhizon, to determine whether these enzymes produce various shikonin/alkannin derivatives in these plants. Twenty-three homologs of LeSAT1 and LeAAT1 were identified in L. erythrorhizon and the other species of Boraginaceae, with functional analyses showing that these enzymes could be classified into five groups according to their specificity for acyl donors and subcellular localization. Because the functions of these homologs did not vary among plant species, additional factors are likely involved in the diversity in acyl moieties of shikonin/alkannin derivatives in Boraginaceae plants.
Results
Identification of LeSAT1 and LeAAT1 orthologs in Boraginaceae plants
Deposited RNA-seq data of five species of Boraginaceae, including three shikonin/alkannin-producing species, Echium plantagineum, A. euchroma and Cynoglossum officinale (Supplementary Fig. S2) (Papageorgiou et al. 1999), and two shikonin/alkannin non-producing species, Symphytum officinale and Heliotropium arborescens (Dresler et al. 2017), were searched for orthologs of LeSAT1 and LeAAT1. In addition, the RNA-seq data of L. erythrorhizon were again searched for paralogs of LeSAT1 and LeAAT1. Using LeSAT1 as a query, 23 homologs were identified (Supplementary Table S1), including two paralogs of L. erythrorhizon (LeSAT2 and LeAAT2). Because intact A. euchroma plants could not be obtained and LeSAT1 orthologs could not be amplified in the roots, stems and leaf tissues of C. officinale, transcripts in both plants were synthesized. Genes with high amino acid sequence identities (86–99%) were detected in E. plantagineum, e.g. EpSAT1-1 and EpSAT1-2. Phylogenetic analysis showed that these homologs could be classified into five groups: a clade containing LeSAT1 and its sister clade, another clade with LeAAT1 and its sister clade, and candidates that did not belong to any of these four clades (Fig. 1A). The intra- and inter-clade amino acid sequence identities were found to be 76–99% and 60–74%, respectively.
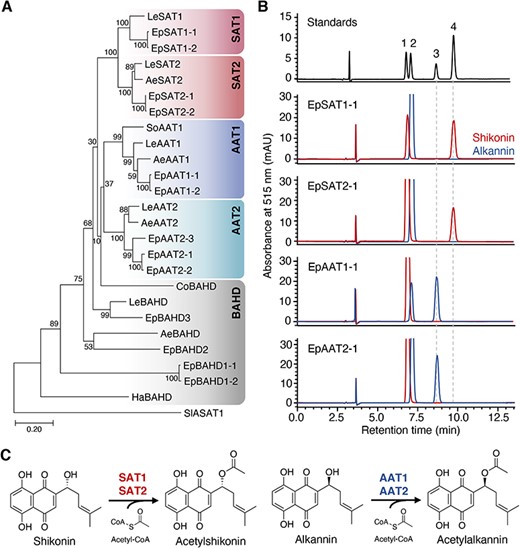
Stereospecific acetylation of shikonin and alkannin. (A) Phylogenetic analysis of LeSAT1 and LeAAT1 homologs of six Boraginaceae plants. Amino acid sequences were obtained from L. erythrorhizon (Le), E. plantagineum (Ep), A. euchroma (Ae), S. officinale (So), C. officinale (Co) and H. arborescens (Ha). Sequence alignment was performed using MAFFT, and a phylogenetic tree was generated using MEGA7 using the maximum-likelihood method. Bootstrap values based on 1,000 replicates are shown at the branching points. (B) Acetylation of shikonin and alkannin by SATs and AATs as analyzed by chiral HPLC. SAT1 and SAT2 mediated the acetylation of shikonin, whereas AAT1 and AAT2 mediated the acetylation of alkannin. Labeled compounds: 1, shikonin; 2, alkannin; 3, acetylalkannin; 4, acetylshikonin. (C) Reaction schemes of SATs and AATs.
Stereospecific acetyltransferase activity of BAHD acyltransferases in Boraginaceae
To investigate their ability to acetylate shikonin and alkannin, the recombinant proteins encoded by the 23 homologs of LeSAT1 and LeAAT1 were expressed in Escherichia coli and purified by nickel affinity chromatography. All SAT1s and BAHDs with poor solubility were co-expressed with chaperonins and were co-eluted during the purification process, in a manner identical to that of LeSAT1 (Supplementary Table S2) (Oshikiri et al. 2020). The acetylation activity of these recombinant enzymes was evaluated using acetyl-CoA as an acyl donor and either shikonin or alkannin as an acyl acceptor. All SAT1s and SAT2s had stereospecific acetyltransferase activity for shikonin, and all AAT1s and AAT2s had stereospecific acetyltransferase activity for alkannin (Fig. 1B, Supplementary Fig. S3). In contrast, none of the BAHDs had acetyltransferase activities for shikonin/alkannin, except that LeBAHD produced a trace amount of acetylalkannin (Supplementary Fig. S3). These results showed that SAT1s, SAT2s, AAT1s and AAT2s function as shikonin/alkannin acyltransferases in other Boraginaceae plants in a manner identical to their function in L. erythrorhizon (Fig. 1C).
Acyl donor substrate preferences of SATs and AATs
To evaluate the acyl donor specificities of these homologs, each SAT and AAT were incubated with a specific acceptor, either shikonin or alkannin, and an acyl-CoA donor, i.e. acetyl-CoA, isobutyryl-CoA, isovaleryl-CoA or methylcrotonyl-CoA (Fig. 2A). High-performance liquid chromatography (HPLC) analysis revealed that SATs and AATs recognized acetyl-CoA, isobutyryl-CoA and isovaleryl-CoA to produce their corresponding shikonin/alkannin derivatives, but did not recognize methylcrotonyl-CoA as an acyl donor (Fig. 2B–R). Both SAT1s and SAT2s showed the highest acylation activity when acetyl-CoA was the acyl donor, whereas the acylation activities of SAT2s tended to be lower than those of SAT1s when isovaleryl-CoA was the acyl donor (Fig. 2B–H). These findings indicated that SAT1s and SAT2s, which showed redundancy in acyl acceptor selectivity, slightly differed in acyl donor selectivity, and that these features were conserved among plant species. Similarly, AAT1s and AAT2s showed distinct acyl donor specificities, with their highest activities observed when acetyl-CoA and isobutyryl-CoA, respectively, were the acyl donors (Fig. 2I–R). These findings suggested that these acyltransferases contribute to the biosynthesis of shikonin/alkannin derivatives with short-chain acyl moieties in a molecular species–specific manner in Boraginaceae plants.
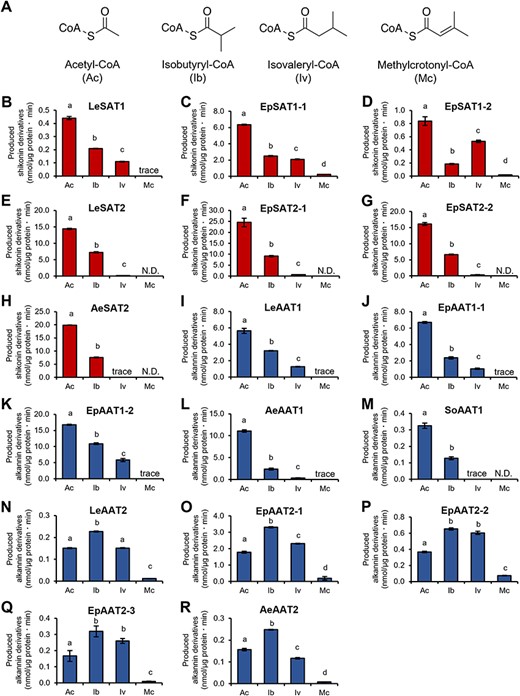
Acyl donor specificities of SATs and AATs. (A) Chemical structures of acyl donors used in the enzyme assays: acetyl-CoA (Ac), isobutyryl-CoA (Ib), isovaleryl-CoA (Iv) and methylcrotonyl-CoA (Mc). Acyltransferase activities of (B) LeSAT1, (C) EpSAT1-1, (D) EpSAT1-2, (E) LeSAT2, (F) EpSAT2-1, (G) EpSAT2-2, (H) AeSAT2, (I) LeAAT1, (J) EpAAT1-1, (K) EpAAT1-2, (L) AeAAT1, (M) SoAAT1, (N) LeAAT2, (O) EpAAT2-1, (P) EpAAT2-2, (Q) EpAAT2-3 and (R) AeAAT2 determined using four acyl donors and either shikonin or alkannin as an acyl acceptor. The amounts of various shikonin/alkannin derivatives produced per reaction time and enzyme content were compared. N.D., not detected; trace, trace amounts detected. Significant differences (P < 0.05) of means ± standard error (n = 3) were determined using the Turkey–Kramer test and are indicated by different lowercase letters.
Subcellular localization of SATs and AATs in Nicotiana benthamiana
To determine the functional differences, other than acyl donor specificity, between groups of SATs and AATs, their subcellular localization was analyzed. Green fluorescent protein (GFP) was fused to the C-terminals of LeSAT1, LeSAT2, LeAAT1 and LeAAT2, and vectors to express these fused proteins were introduced into Nicotiana benthamiana leaves via Agrobacterium tumefaciens–mediated infiltration. The GFP fluorescence of LeSAT2 and LeAAT1 was observed in the cytosol (Fig. 3A), whereas that of LeSAT1 and LeAAT2 was detected in the mitochondria (Fig. 3B). Similar results were observed for other SATs and AATs, i.e. the GFP fluorescence of EpSAT2-1, EpAAT1-1 and SoAAT1 was observed in the cytosol and that of EpSAT1-2 and EpAAT2-2 in the mitochondria (Supplementary Fig. S4A, B), indicating that their subcellular localizations were conserved within these groups of enzymes. BAHDs unable to acetylate both shikonin and alkannin were localized to both the cytosol and mitochondria, except that LeBAHD showed distinct localization to the mitochondria (Supplementary Fig. S4C).
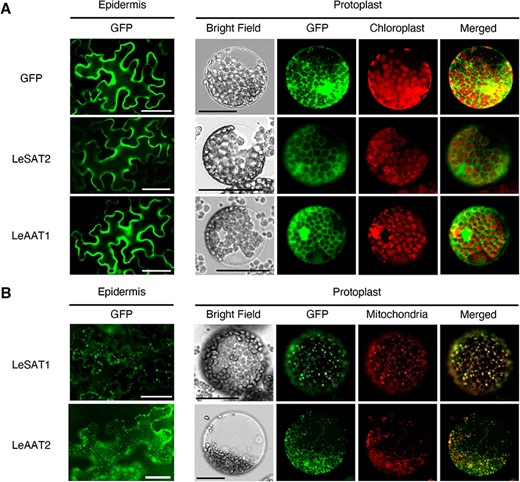
Subcellular localization of LeSAT1, LeSAT2, LeAAT1 and LeAAT2 in N. benthamiana leaves. (A) Epidermal cells and protoplasts of N. benthamiana leaves following introduction of LeSAT2-GFP, LeAAT1-GFP or free GFP (control). Fluorescence was evaluated 4 d after infiltration. (B) Expression of LeSAT1-GFP or LeAAT2-GFP in epidermal cells and protoplasts of N. benthamiana leaves. Mitochondria were stained with Mitotracker Orange CMTMRos before observation. Bars = 50 μm.
To determine whether these SAT1s and AAT2s have a mitochondrial targeting sequence at their N-termini, vectors to express LeSAT1 missing the first 10, 20, 40 and 70 N-terminal amino acids were constructed (Supplementary Fig. S5A) and the acetylation activity of these truncated proteins was investigated in an E. coli expression system. Elimination of the first 10 N-terminal amino acids resulted in a significant decrease in acetylation activity, whereas elimination of the first 20, 40 and 70 N-terminal amino acids completely abolished its activity (Supplementary Fig. S5B). Fusion of GFP with the first 10 N-terminal amino acids of LeSAT1 resulted in the detection of GFP fluorescence in the cytosol, whereas fusion of GFP with the first 70 N-terminal amino acids of LeSAT1 resulted in the detection of GFP fluorescence partly in the mitochondria (Supplementary Fig. S5C, D). These results suggested that LeSAT1 possesses a functional domain between N-terminal amino acids 10 and 70 that allowed it to localize to the mitochondria rather than having a truncatable signal peptide at the N-terminal. When the first 70 N-terminal amino acids were compared between cytosolic and mitochondrial BAHD acyltransferases, a cationic amino acid residue below the IKPSSPTP motif (Tuominen et al. 2011) is conserved in the mitochondrial BAHD acyltransferases (Supplementary Fig. S6), suggesting that this residue might be involved in the mitochondrial localization.
Effect of SAT and AAT gene expression levels on the composition of shikonin/alkannin derivatives
The expression levels of each SAT and AAT and the composition of shikonin/alkannin derivatives in L. erythrorhizon and E. plantagineum were analyzed to investigate their linkages. Because EpSAT1-1 and EpSAT1-2 have very similar coding sequences, RT-qPCR analysis did not distinguish between these two genes. Similarly, RT-qPCR could not distinguish between three other pairs of genes, EpSAT2-1 and EpSAT2-2, EpAAT1-1 and EpAAT1-2, and EpAAT2-1 and EpAAT2-2. All four SATs and AATs were expressed in the roots of L. erythrorhizon, with the expression of LeSATs being approximately 5-fold higher than that of LeAATs (Fig. 4A). In E. plantagineum, however, only EpSAT2 and EpAAT1, whose proteins localized in the cytosol, were highly expressed with the latter being dominant (Fig. 4B).
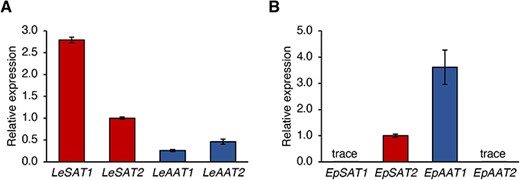
RT-qPCR analysis of SATs and AATs in roots of (A) L. erythrorhizon and (B) E. plantagineum. GAPDH expression was used as the control, and values are means ± standard deviation of three technical replicates. These experiments were performed independently on two different individual plants of each species.
Approximately 84% of the derivatives in L. erythrorhizon were shikonin derivatives, with the most abundant being acetylshikonin (24.1%), followed by isovalerylshikonin (14.8%) and isobutyrylshikonin (14.7%) (Fig. 5, Supplementary Table S3). Approximately 70% of the derivatives in E. plantagineum were alkannin derivatives, with the most abundant being isovalerylalkannin (23.9%) and acetylalkannin (19.8%) (Fig. 5, Supplementary Table S4). The ratios of shikonin to alkannin derivatives correlated with the ratios of SAT to AAT gene expression levels in both L. erythrorhizon and E. plantagineum, indicating that SATs and AATs contribute highly to the stereoisomer ratio of shikonin to alkannin derivatives in Boraginaceae plants.
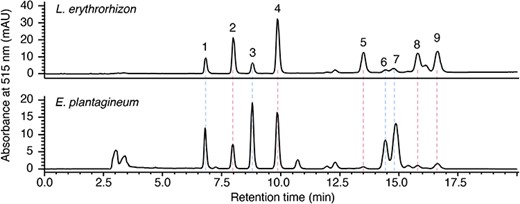
Analysis of shikonin/alkannin derivatives in root extracts. Methanol extracts of L. erythrorhizon and E. plantagineum were subjected to HPLC with a chiral column, and each representative chromatogram is shown. Labeled compounds: 1, β-hydroxyisovalerylalkannin; 2, β-hydroxyisovalerylshikonin; 3, acetylalkannin; 4, acetylshikonin; 5, isobutyrylshikonin; 6, α-methyl-n-butyrylalkannin; 7, isovalerylalkannin; 8, α-methyl-n-butyrylshikonin; 9, isovalerylshikonin.
Discussion
The present study characterized 23 shikonin/alkannin acyltransferase homologs in six species of Boraginaceae plants. Shikonin-/alkannin-producing plants have four groups of enzymes, SAT1, SAT2, AAT1 and AAT2, which differ in substrate specificities and subcellular localization. Acyl donor specificities were conserved among plant species, suggesting that these enzymes may not be directly involved in the production of the side chain variety of shikonin/alkannin derivatives.
Four groups of SATs and AATs were highly conserved in shikonin-/alkannin-producing species belonging to tribe Lithospermeae. A recent study suggested that, in addition to LeSAT1 and LeAAT1, two other BAHD members that descended from a common ancestor were present in the genome of L. erythrorhizon (Tang 2021). These genes corresponded to LeSAT2 and LeAAT2 in the present study. The enzyme copies of each SAT and AAT isolated from E. plantagineum were found to be functionally redundant, except for EpSAT1-1 and EpSAT1-2, which showed distinct specificities for isobutyryl-CoA and isovaleryl-CoA, respectively. These results suggest that gene duplication and neofunctionalization were responsible for enzyme diversification (Panchy et al. 2016). In contrast, SAT1 was not detected in the transcriptome data of A. euchroma. Because the levels of expression of the SAT and AAT genes correlated with the ratio of shikonin to alkannin derivatives, SAT1 may be only slightly expressed in A. euchroma, which mainly produces alkannin derivatives (Zhou et al. 2011). Similarly, because of the inability to produce shikonin/alkannin derivatives, SATs and AATs were not detected in C. officinale. Although C. officinale and its related species have been reported to produce shikonin derivatives (Fedoreev et al. 1979, Olennikov et al. 2020), other studies failed to detect these derivatives in Cynoglossum species (Dresler et al. 2017, Jeziorek et al. 2019). It is therefore unclear whether C. officinale possesses functional SAT and AAT homologs. In contrast, a functional SoAAT1 was isolated from S. officinale in this study (Supplementary Fig. S3), a species that does not produce shikonin/alkannin derivatives, indicating that the common ancestor of tribe Lithospermeae and Boragineae acquired at least one gene encoding an AAT. Comprehensive genomics approaches in Boraginaceae plants may shed light on the molecular evolution of SAT and AAT.
The acyl donor specificities and subcellular localizations of SATs and AATs, which were grouped according to the phylogenetic tree, suggested a functional differentiation between these groups of enzymes. The finding that previously characterized BAHD acyltransferases localized to the cytosol (Fujiwara et al. 1998, Carqueijeiro et al. 2018, Fu et al. 2021) suggested that these enzymes utilize cytosolic acyl-CoA as an acyl donor (Bontpart et al. 2015). In turn, SAT1s and AAT2s localized to the mitochondria and recognized isobutyryl-CoA and isovaleryl-CoA, which are produced during the catabolism of branched-chain amino acids in the mitochondria (Taylor et al. 2004, Binder 2010, Latimer et al. 2018). SAT1s and AAT2s were found to have somewhat higher catalytic activity to isobutyryl-CoA and isovaleryl-CoA than SAT2s and AAT1s, suggesting that SATs and AATs utilize acyl-CoA pools corresponding to the organelles in which they localize. In other plants, the BAHD acyltransferase CER2, which is involved in the biosynthesis of very-long-chain fatty acids, localizes to the endoplasmic reticulum (ER) by interaction with other enzymes in the same biosynthetic pathway on the ER membrane (Xia et al. 1997, Haslam et al. 2012, 2017). LeSAT1 may interact with other proteins that localize to the mitochondria in a similar manner. However, there is an inconsistency. Studies using 14C-labeled deoxyshikonin and shikonin suggested an interaction between an acetyltransferase that synthesizes acetylshikonin from shikonin, i.e. LeSATs, and a hydroxylase that synthesizes shikonin from deoxyshikonin, recently identified as LeDSH1, a cytochrome P450 generally localized to the ER (Okamoto et al. 1995, Song et al. 2021). Further studies, such as analyses of knockout mutants and proteomics following immunoprecipitation of SATs and AATs, may determine the mechanism by which SAT1 and AAT2 interact with other proteins and localize to the mitochondria. Some protein–protein interactions alter their conformations and activities. For example, the interaction of chalcone synthase with chalcone isomerase–like proteins alters the products produced by the former (Ban et al. 2018, Waki et al. 2020). Results showing that SAT1s require chaperonins for solubilization in E. coli and that the activities of AAT2s were lower than those of AAT1s suggest that interactions of SAT1s and AAT2s with other proteins may be required for their stabilization and/or activation in vivo.
Unexpectedly, the present findings suggested that, in addition to the functional differentiation among SATs and AATs, other, yet undetermined factors may be responsible for the production of various shikonin/alkannin derivatives in plant species. Differences in both acyltransferase activities and acyl-CoA compositions enhanced the variation of acyl chains in acylsugars in Solanaceae plants (Fan et al. 2019). For example, deletion of the isopropylmalate synthase–like 3 (IPMS3) gene, which encodes the protein that synthesizes 2-isopropylmalate from 2-oxoisovalerate in the branching point from the isobutyryl-CoA biosynthesis pathway to the isovaleryl-CoA biosynthesis pathway, greatly influenced the profile of acylsugars in tomato species (Ning et al. 2015). Moreover, the absence of mitochondrial acyl-CoA synthase and enoyl-CoA hydratase disrupted C10–C12 middle-chain acylsugars (Fan et al. 2020). Differences in acyl-CoA profiles among Boraginaceae species may therefore be responsible for the diversity of shikonin/alkannin derivatives. In addition, because SATs and AATs were generally unable to use methylcrotonyl-CoA as an acyl donor, β,β-dimethylacrylshikonin/alkannin often found in Lithospermeae may be produced by other acyltransferases or by yet unknown enzymes that modify shikonin/alkannin derivatives produced by SATs and AATs.
Materials and Methods
Plant materials and chemicals
Lithospermum erythrorhizon plants were grown and harvested as described (Oshikiri et al. 2020). Seedlings of E. plantagineum, C. officinale and H. arborescens were purchased and grown until flowering. Symphytum officinale plants were harvested from a field in Nagano, Japan. Nicotiana benthamiana plants used for subcellular localization analysis were grown at 23°C with 16 h light/8 h dark cycles in a cultivation chamber for 2 months. Shikonin, alkannin and their derivatives were purchased from Nagara Science (Gifu, Japan). Acetyl-CoA was purchased from Fujifilm Wako (Osaka, Japan), and isobutyryl-CoA, isovaleryl-CoA and methylcrotonyl-CoA were purchased from Sigma-Aldrich (St. Louis, Mo, USA).
Transcriptome assembly and identification of LeSAT1 homolog gene
To identify the LeSAT1 homologs in Boraginaceae plants, RNA-seq data of L. erythrorhizon, E. plantagineum, A. euchroma, S. officinale, C. officinale and H. arborescens in the Sequence Read Archive (SRA) database were assembled by Trinity (Supplementary Table S5) (Haas et al. 2013). The transcriptome completeness was assessed using BUSCO (Simão et al. 2015) (Supplementary Fig. S7). Candidate genes were searched using tblastn programs with LeSAT1 as a query. Genes coding full-length open reading frames (ORFs) were selected for subsequent experiments.
Phylogenetic analysis
A maximum-likelihood phylogenetic tree of LeSAT1 and LeAAT1 homologs was generated from amino acid sequences aligned by MAFFT program using MEGA version 7 (Kumar et al. 2016), based on a JTT matrix–based model with a discrete Gamma distribution (five categories), and bootstrap of 1,000 replicates. SlASAT1, the acylsugar acyltransferase of Solanum licopersicum (accession number: ALU64003), was used as an out-group.
Cloning and heterologous expression of SATs and AATs
Total RNAs were extracted from the roots and leaves of each Boraginaceae plant using ISOSPIN Plant RNA (Nippon Gene, Tokyo, Japan). These RNA samples were treated with DNaseI, followed by reverse transcription using ReverTra Ace (Toyobo, Osaka, Japan) with oligo-dT primers. The full-length ORFs and LeSAT1s without N-terminal sequences were amplified using prepared cDNAs as templates and primers corresponding to each gene (Supplementary Tables S6, S7), and the products were cloned into the NdeI/SalI sites of pCold I vector (Takara Bio, Shiga, Japan). AeSAT2, AeAAT1, AeAAT2, AeBAHD and CoBAHD were synthesized by Eurofins Genomics (Tokyo, Japan) and cloned into the NdeI/SalI sites of pCold I vector using specific primers (Supplementary Table S6). All AAT2 constructs were used to transform E. coli Rosetta2 (DE3), and other constructs were used to transform E. coli Origami strain. For expression of SAT1s and BAHDs, E. coli was co-transformed with pGro7 plasmids encoding the chaperonins GroEL and GroES (Takara Bio) (Supplementary Table S2). Transformants were cultured in LB medium until they reached an optical density at 600 nm (OD600) of 0.6–0.8. The bacteria were cooled on ice for 20 min, and 0.5 mM of isopropyl β-d-1-thiogalactopyranoside was added to induce protein expression, followed by further cultivation at 15°C for 12–24 h. Proteins were extracted and purified as described (Oshikiri et al. 2020). To purify SAT1s, wash buffer containing 10 mM ATP was treated before elution as described (Oshikiri et al. 2020). Purified enzyme fractions were desalted on a PD-10 column containing 50 mM of MES (pH 6.5) buffer for enzyme assays.
Enzyme assays
The enzyme assays were performed as previously described with slight modifications (Oshikiri et al. 2020). Briefly, enzyme assays were performed in 50 μl of reaction mixtures containing 50 mM MES (pH 5.5), 10 mM sodium ascorbate, 0.3% (v/v) Triton-X, 250 μM acyl donor, 250 μM shikonin or alkannin and enzyme fraction (25 μl). Samples were incubated at 30°C for 1–5 min to determine the acyl donor specificities or for 5 min to detect stereospecific acetylation activities. The reaction products were extracted with equal volumes of ethyl acetate and analyzed by HPLC as previously reported (Oshikiri et al. 2020).
Transient expression and subcellular localization analysis
The ORFs of LeSAT1, LeSAT2, LeAAT1, LeAAT2, EpSAT1-2, EpSAT2-1, EpAAT1-1, EpAAT2-2, SoAAT1, LeBAHD, CoBAHD, AeBAHD and HaBAHD were individually subcloned into the SalI/NotI sites of the pENTR 1A Dual selection vector (Invitrogen, Waltham, MA, USA) and transferred to the pGWB505 binary vector using Gateway LR clonase enzyme (Invitrogen) (Nakagawa et al. 2007). The primer sequences used in subcloning are listed in Supplementary Table S8. N70LeSAT1 and N10LeSAT1-GFP were amplified with corresponding primer sets (Supplementary Table S7) and subcloned into the pENTR/D-TOPO vector (Invitrogen). N70LeSAT1 and N10LeSAT1-GFP were introduced into the vectors pGWB505 and pGWB502, respectively, and these constructs were used to transform the LBA4404 strain of A. tumefaciens. Transformed agrobacteria were grown overnight in Yeast Extract Beef (YEB) medium at 28°C, harvested and resuspended in sterilized water at an OD600 of 0.6 with agrobacterium harboring p19 RNA interference suppressor. The suspension was infiltrated into the leaves of N. benthamiana. pHKN29 harboring GFP was used as a control for cytoplasm localization (Kumagai and Kouchi 2003). After 4 d, the fluorescence of leaf epidermal cells and their protoplasts, prepared with 20 mM MES buffer (pH 6.5) containing 5 mM MgCl2, 600 mM sorbitol, 1% (w/v) cellulase and 0.5% (w/v) macerozyme, was assessed using a fluorescence microscope (KEYENCE BZ-X710). Mitochondria were stained with 500 nM MitoTracker Orange CMTMRos (Invitrogen) for 3 h before protoplast preparation.
Gene expression analysis of SATs and AATs
RT-qPCR analyses were performed using a KOD SYBR qPCR Mix (Toyobo) with gene-specific primers (Supplementary Table S9) on a StepOnePlus Real-Time PCR System (Thermo Fisher Scientific, Waltham, MA, USA). Root cDNAs of L. erythrorhizon and E. plantagineum prepared as described earlier were used as templates. Gene expression levels were normalized using GAPDH as an internal standard and calculated relative to LeSAT2 or EpSAT2 using the 2−ΔΔCt method (Livak and Schmittgen 2001).
Detection of shikonin, alkannin and their derivatives in root extract
Approximately 0.1 g of each frozen root was ground with metal beads in liquid nitrogen and extracted with 10-fold (v/w) methanol for 3 h. After centrifugation, each supernatant was evaporated and dissolved in 100 μl of methanol. Each extract was filtered with a non-sterile syringe filter (0.45 μm, Hawach Scientific, Xian, China) and analyzed by HPLC with a CHIRALPAK IH-3 column as described (Oshikiri et al. 2020).
Supplementary Data
Supplementary Data are available at PCP online.
Data Availability
The data underlying this article are available in the DNA Data Bank of Japan (https://www.ddbj.nig.ac.jp/index-e.html), under the accession numbers: LC747165–LC747187 (Supplementary Table S1).
Funding
Japan Society for the Promotion of Science KAKENHI (19K05825 and 22K05438 to K.T. and 21J12807 to H.O.), the Sasakawa Scientific Research Grant from The Japan Science Society (2020-4082 to H.O.) and a research grant for Mission Research on Sustainable Humanosphere from Research Institute for Sustainable Humanosphere, Kyoto University.
Acknowledgments
The authors thank Dr. T. Nakagawa (Shimane University) for the series of pGWB plasmids, and Amato Pharmaceutical Products Ltd., for providing the L. erythrorhizon seeds.
Author Contributions
K.T. conceived the research plan and designed the experiments; H.O. performed most of the experiments; H.L. performed transcriptome analysis; M.M. performed a part of the HPLC analysis and cloning; H.Y. and K.Y. interpreted the results and commented on the manuscript; H.O. and K.T. wrote the manuscript.
Disclosures
The authors have no conflicts of interest to declare.