-
PDF
- Split View
-
Views
-
Cite
Cite
Nanaka Murakami, Saashia Fuji, Shota Yamauchi, Sakurako Hosotani, Jun’ichi Mano, Atsushi Takemiya, Reactive Carbonyl Species Inhibit Blue-Light-Dependent Activation of the Plasma Membrane H+-ATPase and Stomatal Opening, Plant and Cell Physiology, Volume 63, Issue 8, August 2022, Pages 1168–1176, https://doi.org/10.1093/pcp/pcac094
- Share Icon Share
Abstract
Reactive oxygen species (ROS) play a central role in plant responses to biotic and abiotic stresses. ROS stimulate stomatal closure by inhibiting blue light (BL)-dependent stomatal opening under diverse stresses in the daytime. However, the stomatal opening inhibition mechanism by ROS remains unclear. In this study, we aimed to examine the impact of reactive carbonyl species (RCS), lipid peroxidation products generated by ROS, on BL signaling in guard cells. Application of RCS, such as acrolein and 4-hydroxy-(E)-2-nonenal (HNE), inhibited BL-dependent stomatal opening in the epidermis of Arabidopsis thaliana. Acrolein also inhibited H+ pumping and the plasma membrane H+-ATPase phosphorylation in response to BL. However, acrolein did not inhibit BL-dependent autophosphorylation of phototropins and the phosphorylation of BLUE LIGHT SIGNALING1 (BLUS1). Similarly, acrolein affected neither the kinase activity of BLUS1 nor the phosphatase activity of protein phosphatase 1, a positive regulator of BL signaling. However, acrolein inhibited fusicoccin-dependent phosphorylation of H+-ATPase and stomatal opening. Furthermore, carnosine, an RCS scavenger, partially alleviated the abscisic-acid- and hydrogen-peroxide-induced inhibition of BL-dependent stomatal opening. Altogether, these findings suggest that RCS inhibit BL signaling, especially H+-ATPase activation, and play a key role in the crosstalk between BL and ROS signaling pathways in guard cells.
Introduction
Stomatal pores, formed by pairs of guard cells in the epidermis of terrestrial plants, control gas exchange between leaves and the atmosphere. Fine control of the stomatal aperture is essential to maximize CO2 uptake for photosynthetic carbon fixation and prevent water loss through transpiration. Guard cells have evolved sophisticated sensory systems to monitor diverse environmental and endogenous stimuli and integrate the signals to optimize the aperture under the given conditions (Assmann and Jegla 2016, Shimazaki et al. 2007, Murata et al. 2015, Zhang et al. 2018). Among the complex signaling network, reactive oxygen species (ROS), such as hydrogen peroxide (H2O2), act as a central hub for regulating stomatal movements (Song et al. 2014, Sierla et al. 2016).
Antioxidant systems, such as ascorbate peroxidase (APX1) and catalase (CAT3), maintain cellular ROS levels under steady-state conditions (Pnueli et al. 2003, Jannat et al. 2011). Autophagy also contributes to ROS homeostasis maintenance in guard cells by eliminating oxidized peroxisomes (Yamauchi et al. 2019). The plasma membrane NADPH oxidases, which are encoded by respiratory burst oxidase homolog, and the cell wall peroxidases, mediate ROS production in response to various plant hormones and environmental factors, such as abscisic acid (ABA), methyl jasmonate, salicylic acid, CO2, pathogen and yeast elicitors (Song et al. 2014). H2O2 triggers an increase in cytosolic free Ca2+ concentration by activating the Ca2+-permeable cation (ICa) channels localized to the plasma membrane, thereby activating the S-type anion channels encoded by SLOW ANION CHANNEL-ASSOCIATED 1 (SLAC1) (Pei et al. 2000, Kwak et al. 2003, Hua et al. 2012). The anion efflux from guard cells via SLAC1 causes plasma membrane depolarization, promoting K+ efflux via voltage-dependent outward-rectifying K+ channels and stomatal closure (Schroeder et al. 1987). A leucine-rich repeat receptor-like kinase (LRR-RLK) GUARD CELL HYDROGEN PEROXIDE-RESISTANT1 (GHR1) is involved in H2O2-induced ICa channel activation (Hua et al. 2012). HYDROGEN PEROXIDE-INDUCED Ca2+ INCREASES 1 (HPCA1), a membrane-localized LRR-RLK, acts as a sensor for extracellular H2O2 to mediate ICa channel activation in guard cells and stomatal closure (Wu et al. 2020).
ROS accelerate stomatal closure by inhibiting the stomatal opening processes in guard cells (Zhang et al. 2004). The blue light (BL) receptor phototropins (phot1 and phot2) mediate stomatal opening in response to BL (Kinoshita et al. 2001, Shimazaki et al. 2007, Inoue and Kinoshita 2017). Phototropins contain two light-sensing light, oxygen, or voltage (LOV) domains in the N-terminus and a Ser/Thr kinase domain in the C-terminus (Christie 2007). The light-activated phototropins phosphorylate BLUE LIGHT SIGNALING1 (BLUS1), a Ser/Thr protein kinase specifically expressed in guard cells (Takemiya et al. 2013a, Takemiya and Shimazaki 2016). Phosphorylation of BLUS1 C-terminal auto-inhibitory regulatory domain by phototropins is essential for BLUS1 activation and stomatal opening (Takemiya et al. 2013a, Hosotani et al. 2021). The signal from BLUS1 mediates the activation of the plasma membrane H+-ATPase via phosphorylation of the penultimate Thr and phosphorylation-dependent binding of 14-3-3 proteins (Kinoshita and Shimazaki 1999, Emi et al. 2001). The consequent H+ pumping causes plasma membrane hyperpolarization, allowing K+ uptake in guard cells through voltage-gated inward-rectifying K+ channels, resulting in water influx and stomatal opening (Schroeder et al. 1987). The Raf-like mitogen-activated protein kinase kinase kinase (MAPKKK) BLUE LIGHT-DEPENDENT H+-ATPASE PHOSPHORYLATION (BHP) and protein phosphatase 1 (PP1) act as positive regulators of the signaling pathway linking BLUS1 to the H+-ATPase (Takemiya et al. 2006, 2013b, Hayashi et al. 2017). Additionally, phototropins phosphorylate CONVERGENCE OF BLUE LIGHT AND CO2 1 (CBC1) kinase, thereby inactivating SLAC1 anion channels to promote stomatal opening (Hiyama et al. 2017). ABA inhibits BL activation of H+-ATPase, preventing membrane hyperpolarization and opposing stomatal opening (Shimazaki et al. 1986, Goh et al. 1996). Such H+-ATPase inhibition is crucial for sustaining plasma membrane depolarization and promoting stomatal closure under drought stress in the daytime (Merlot et al. 2007). Furthermore, ABA-induced H2O2 generation mediates the inhibition of BL-dependent activation of H+-ATPase (Zhang et al. 2004). However, the mechanisms by which H2O2 inhibits H+-ATPase activation remain enigmatic.
Increasing evidence has suggested that reactive carbonyl species (RCS), the α,β-unsaturated aldehydes and ketones, act as second messengers of ROS signaling (Mano et al. 2019, Biswas and Mano 2021). ROS oxidize various biomolecules, including membrane lipids, facilitating lipid peroxide (LOOH) production. The LOOH is further oxidized to generate RCS via enzymatic or nonenzymatic radical-catalyzed reactions (Blée 1998). RCS have high electrophilicity and can form covalent bonds with proteins, causing the alteration of protein functions and cellular processes (Mano 2012, Mano et al. 2019). Under nonstress conditions, cellular RCS are maintained at micromolar levels, which are increased by several times upon stress treatments (Mano et al. 2010, Yin et al. 2010, Biswas and Mano 2015). Treatments of ABA and H2O2 to leaf epidermal tissues of Arabidopsis and tobacco result in a significant increase in several RCS, including acrolein and 4-hydroxy-(E)-2-nonenal (HNE) (Islam et al. 2016, 2019). Acrolein and HNE induce stomatal closure, and RCS scavengers diminish ABA- and H2O2-induced stomatal closure (Islam et al. 2019). Acrolein causes cytosolic Ca2+ elevation by activating the ICa channels in guard cells (Islam et al. 2019). Additionally, acrolein inhibits light-dependent stomatal opening by inhibiting inward K+ channels in guard cells (Islam et al. 2016). Thus, RCS appear to function as key signal mediators in the ROS signaling pathway in guard cells. However, whether RCS inhibit BL signaling to mediate H2O2-induced inhibition of stomatal opening remains unclear.
In this study, we determined whether RCS could inhibit BL-dependent activation of H+-ATPase and stomatal opening. We report that RCS inhibit the BL signaling pathway between BLUS1 and H+-ATPase, especially H+-ATPase activation.
Results
RCS inhibit BL-dependent stomatal opening
To determine whether RCS inhibit BL-dependent stomatal opening, we analyzed the effect of acrolein and HNE on stomatal responses to red light (RL) and BL in epidermal tissues from Arabidopsis wild-type leaves. RL-induced slight stomatal opening, and simultaneous irradiation of RL and BL elicited significant stomatal opening in control (Fig. 1). Application of 10 and 100 µM acrolein to the epidermis suppressed BL-dependent stomatal opening in a concentration-dependent manner. Similarly, HNE inhibited stomatal opening in response to BL. We also confirmed that acrolein suppressed BL-dependent stomatal opening in phot1 and phot2 single mutants (Supplementary Fig. S1). These results suggest that RCS inhibit BL-dependent stomatal opening.
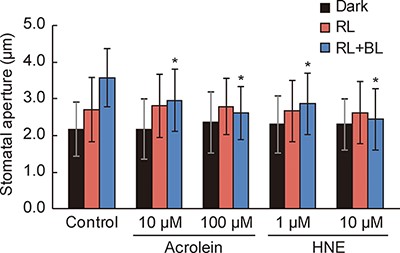
RCS inhibit BL-dependent activation of H+-ATPase
BL-dependent activation of H+-ATPase creates the driving force for stomatal opening (Kinoshita and Shimazaki 1999). To clarify whether RCS inhibit H+-ATPase activation, we examined BL-induced H+ pumping from guard cell protoplasts. A pulse of BL elicited H+ pumping, and acrolein application significantly suppressed the response (Fig. 2A). The H+ pumping was inhibited by 70% and 90% by 10 and 100 µM acrolein, respectively (Fig. 2B).
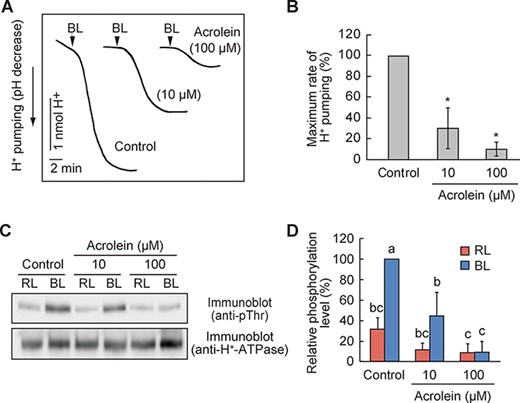
We further verified whether RCS could inhibit BL-dependent phosphorylation of the penultimate Thr residue of H+-ATPase by immunoblot analysis using phospho-specific antibodies. Consistent with H+ pumping, H+-ATPase phosphorylation in response to BL was decreased by applying acrolein (Fig. 2C, D). Altogether, these data suggest that RCS inhibit BL activation of H+-ATPase.
RCS have been shown to cause programmed cell death in plants (Biswas and Mano 2015). Therefore, we examined the viability of guard cells treated with acrolein by fluorescein diacetate (FDA) staining. No difference in viability between control and acrolein-treated cells was found, suggesting that this level of acrolein was not detrimental to guard cells (Supplementary Fig. S2).
RCS do not inhibit the upstream BL signaling
Given that RCS inhibit BL-dependent phosphorylation of H+-ATPase (Fig. 2C, D), we suspected that RCS might suppress BL signaling between phototropins and H+-ATPase. We examined whether RCS could affect BL activation of phototropin kinases. BL perception by the LOV domain induces autophosphorylation of phototropins, an essential step for downstream signal transduction (Inoue et al. 2008). We evaluated the effect of acrolein on BL-dependent autophosphorylation of phototropins by the phosphorylation-dependent electrophoretic mobility shift assay. Both phot1 and phot2 exhibited BL-induced mobility shifts on sodium dodecyl sulfate-polyacrylamide gel electrophoresis (SDS-PAGE) (Fig. 3A), and such mobility shifts were seen even in acrolein-treated guard cells (Fig. 3A).
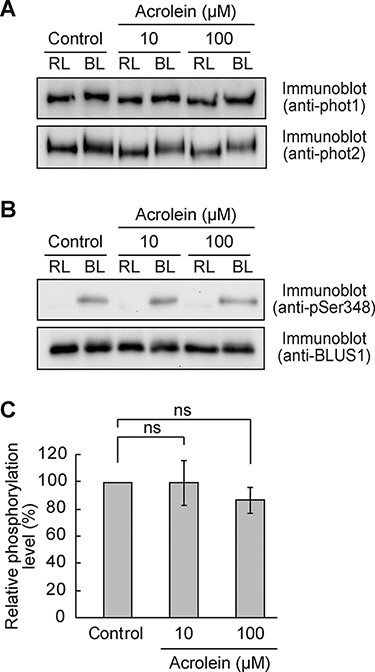
Effect of RCS on BL-dependent phosphorylation of phototropins and BLUS1.
The light-activated phototropins phosphorylate the downstream target BLUS1 to mediate H+-ATPase activation (Takemiya et al. 2013a). Therefore, we examined BL-dependent phosphorylation of BLUS1 at Ser-348 and confirmed no significant difference in the level of phosphorylation between control and acrolein-treated cells (Fig. 3B, C). These results suggest that RCS do not affect BL-induced activation of phototropins in guard cells.
Further, we investigated the effect of RCS on the kinase activity of BLUS1. Recombinant BLUS1 was expressed as an N-terminal fusion to maltose-binding protein (MBP) in Escherichia coli, and the autophosphorylation activity of purified MBP-BLUS1 was evaluated. Autoradiography revealed that wild-type MBP-BLUS1 but not a catalytic inactive mutant MBP-BLUS1 (D157N) exhibited autophosphorylation activity. Furthermore, acrolein did not affect the activity (Fig. 4A, B).
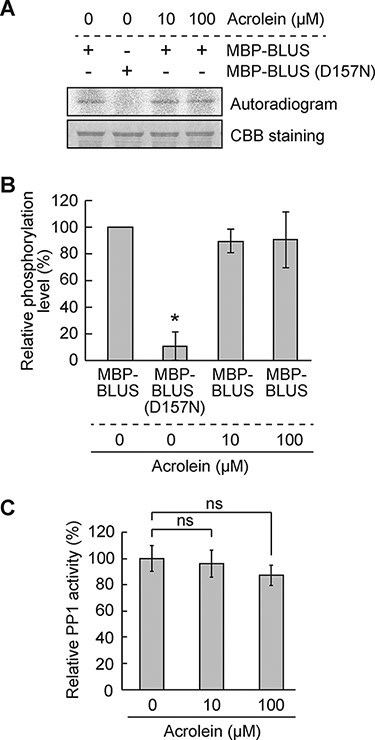
PP1 positively regulates the BL signaling downstream of BLUS1 and upstream of H+-ATPase (Takemiya et al. 2006, 2013a). We conducted an in vitro phosphatase assay to explore whether the PP1 phosphatase activity is affected by RCS and found that acrolein did not affect the PP1 activity even at 100 µM (Fig. 4C). Altogether, these results indicate that RCS do not inhibit early signaling events in the BL activation of H+-ATPase.
RCS inhibit Fc-dependent activation of H+-ATPase and stomatal opening
A fungal toxin fusicoccin (Fc) irreversibly activates H+-ATPase by stabilizing the binding between phosphorylated penultimate Thr of H+-ATPase and 14-3-3 proteins, causing constitutive stomatal opening (Kinoshita and Shimazaki 2001). Fc could stimulate H+-ATPase phosphorylation, bypassing the need for BL signaling. We rationalized that if RCS inhibit H+-ATPase phosphorylation, then Fc-induced responses might be suppressed by RCS treatment. Hence, we applied acrolein to guard cell protoplasts and analyzed H+ pumping by Fc. A significant decrease in H+ pumping was observed with acrolein addition (Fig. 5A, B). Following this result, acrolein suppressed Fc-induced H+-ATPase phosphorylation in a concentration-dependent manner (Fig. 5C, D). Furthermore, along with H+-ATPase inhibition, we confirmed that acrolein suppressed stomatal opening in response to Fc (Fig. 5D).
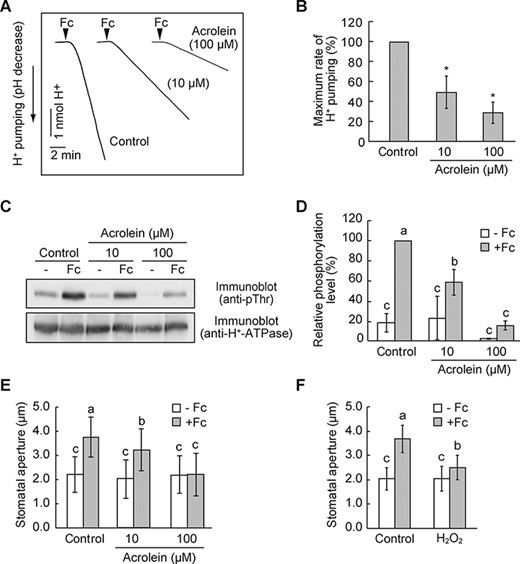
RCS inhibit Fc-dependent activation of H+-ATPase and stomatal opening.
Previous investigation has shown that H2O2 inhibits Fc-induced H+ pumping and phosphorylation of H+-ATPase in guard cell protoplasts (Zhang et al. 2004). In accordance with this, we found that H2O2 suppressed Fc-induced stomatal opening (Fig. 5F). Thus, RCS as well as ROS appeared to inhibit H+-ATPase phosphorylation in the signaling pathway.
Carnosine partially restores the inhibition of BL-dependent stomatal opening by ABA and H2O2
If RCS play a role in ABA and H2O2-induced inhibition of stomatal opening, scavenging of intracellular RCS should diminish the ABA- and H2O2-induced inhibition. To ascertain this, we investigated the effect of carnosine, an RCS scavenger (Biswas and Mano 2015), on the inhibition of BL-dependent stomatal opening. We first verified that carnosine blocked the inhibition of BL-dependent stomatal opening by acrolein and HNE (Fig. 6A). Carnosine alone did not affect stomatal opening to BL (Fig. 6A); however, it alleviated the ABA- and H2O2-induced inhibition of stomatal opening in response to BL (Fig. 6B). Altogether, these results indicate that RCS might be responsible for the inhibition of BL-dependent stomatal opening by ABA and H2O2.
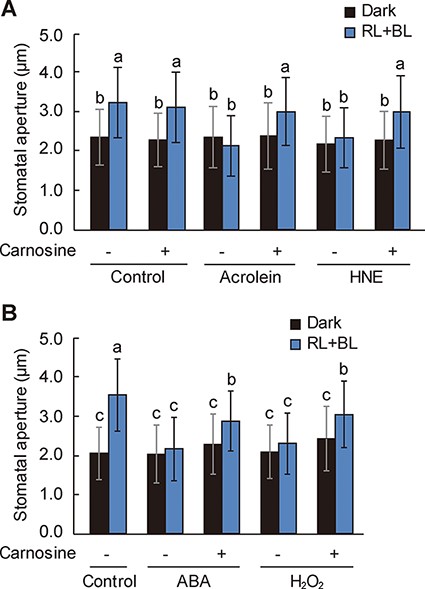
Restoration of ABA- and H2O2-induced inhibition of BL-dependent stomatal opening by carnosine.
Discussion
In this study, we demonstrated that lipid-peroxidation-derived RCS inhibit BL-dependent stomatal opening. Exogenous treatment of Arabidopsis epidermal tissues with acrolein and HNE, major lipid peroxidation products in guard cells (Islam et al. 2016), suppressed stomatal opening in response to BL (Fig. 1). Such inhibition was reversed by adding the carbonyl scavenger carnosine (Fig. 6A). Furthermore, acrolein inhibited BL-dependent H+ pumping and H+-ATPase phosphorylation in guard cell protoplasts (Fig. 2). Acrolein suppresses hyperpolarization-activated K+ channels in guard cells (Islam et al. 2016). Therefore, in addition to inhibiting K+ channels, our data suggest that RCS inhibit BL activation of H+-ATPase, thereby preventing plasma membrane hyperpolarization and stomatal opening.
Phototropins possess two BL-sensing LOV domains, each containing a chromophore flavin mononucleotide (FMN). BL absorption by FMN triggers a covalent adduct formation to the conserved Cys residue within the LOV domain, leading to structural changes and C-terminal kinase domain activation (Christie 2007). Considering that RCS exert their biological effects on intracellular signaling through protein carbonylation to the side chains of Cys, Lys and His residues (Mano et al. 2019), we suspected that the Cys residue within the phototropin LOV domain might be affected by RCS. However, this is erroneous because neither BL-dependent autophosphorylation of phototropins nor phosphorylation of BLUS1 was inhibited by acrolein (Fig. 3), suggesting that RCS do not affect the BL activation of phototropins. Therefore, phototropins are unlikely to be the direct targets of RCS.
Our data suggest that RCS inhibit the BL signaling pathway downstream of BLUS1, thereby suppressing H+-ATPase activation. BLUS1 and PP1 are unlikely to be molecular targets of RCS because their kinase and phosphatase activities were unaffected by acrolein (Fig. 4). Acrolein suppressed Fc-induced H+ pumping and H+-ATPase phosphorylation (Fig. 5A–D). Therefore, RCS might affect regulatory mechanisms of H+-ATPase phosphorylation conserved between BL and Fc. One possibility is that RCS directly modulate the activity of protein kinase or phosphatase, regulating H+-ATPase phosphorylation. D-clade type 2C protein phosphatases (PP2C-D) are responsible for dephosphorylating the penultimate Thr residue of H+-ATPase in guard cells (Akiyama et al. 2022). In contrast, the leucine-rich repeat receptor-like transmembrane kinases (TMKs) directly phosphorylate H+-ATPase in an auxin-dependent manner, although their involvement in the BL signaling is unknown (Li et al. 2021, Lin et al. 2021). Therefore, it will be interesting to witness whether RCS inactivate TMKs or activate PP2C-D in future studies. Alternatively, RCS may be directly bound to H+-ATPase, affecting the interaction with binding proteins, including TMKs and PP2C-D. Further biochemical analysis will help identify the molecular targets of RCS in guard cells.
Our findings provide a link between RCS- and ROS-induced inhibitions of BL-dependent stomatal opening. Carnosine prevents the ABA- and H2O2-induced accumulation of RCS, including acrolein and HNE, in the epidermal tissues of Arabidopsis (Islam et al. 2019). Consistently, our findings show that carnosine diminishes the inhibition of stomatal opening by ABA and H2O2 (Fig. 6B). These data suggest that RCS mediate ABA- and H2O2-induced inhibition of stomatal opening.
However, carnosine could not fully recover the inhibition by ABA and H2O2 (Fig. 6B). This partial recovery may be attributed to the action of second messengers of ROS other than RCS. H2O2 induces nitric oxide (NO) and phosphatidic acid (PA) production in guard cells (Jacob et al. 1999, Garcia-Mata and Lamattina 2002, Neill et al. 2002). Previously, we have demonstrated that NO and PA inhibit the BL-dependent activation of H+-ATPase (Zhang et al. 2007, Takemiya and Shimazaki 2010). Although whether RCS mediate the production of NO and PA, or vice versa, is currently unknown, NO and PA might have different inhibitory effects and targets against the BL signaling than RCS. Consistently, PA, unlike RCS, inhibits the catalytic activity of PP1 (Takemiya and Shimazaki 2010). Therefore, ROS in guard cells appear to act as the central hub for generating various second messengers controlling stomatal opening.
In conclusion, the findings in this study suggest that RCS achieve strict control of stomatal aperture through the inhibition of H+-ATPase activation by BL in addition to ICa channel activation and K+ channel inactivation. Our results provide important insight into how plants optimize gas exchange under diverse stresses in the daytime. Identifying molecular targets for RCS will lead to major advances in understanding ROS signaling and environmental stress responses in plants.
Materials and Methods
Plant materials and growth conditions
The Arabidopsis thaliana ecotype Columbia was used in all experiments. The plants were grown in soil supplemented with vermiculite (1:1) for 4 weeks under white light (50 μmol m−2 s−1) with a 14/10 h light/dark cycle at 24°C.
Measurement of stomatal opening
Epidermal tissues prepared from dark-adapted plants were incubated in stomatal opening buffer containing 5 mM MES-bis-tris propane (pH 6.5), 50 mM KCl and 0.1 mM CaCl2 in the dark or under RL (50 μmol m−2 s−1) with or without BL (10 μmol m−2 s−1) for 2 h at 24°C (Kinoshita et al. 2001). Acrolein, HNE, ABA or H2O2 was added 30 min before the commencement of light illumination. Carnosine was added 40 min before the light illumination. The stomatal aperture in the abaxial epidermis was measured microscopically (Eclipse TS100; Nikon, Tokyo, Japan).
Isolation of guard cell protoplasts and measurement of H+ pumping
Guard cell protoplasts were enzymatically isolated as described previously (Ueno et al. 2005, Takemiya et al. 2013b). BL- and Fc-dependent H+ pumping were determined using a glass pH electrode (Ueno et al. 2005). The guard cell protoplasts were incubated in 0.125 mM MES-NaOH (pH 6.0), 1 mM CaCl2, 0.4 M mannitol and 10 mM KCl under RL (300 μmol m−2 s−1) for 2 h at 24°C, and then a pulse of BL (100 μmol m−2 s−1, 30 s) was superimposed. Fc was added to a final concentration of 10 µM. Acrolein was added 30 min before the BL exposure.
Immunoblot analysis
Phosphorylation of phototropins, BLUS1 and H+-ATPase was detected as described previously (Takemiya et al. 2013a). Briefly, the guard cell protoplasts were illuminated with RL (300 μmol m−2 s−1) for 30 min, and a pulse of BL (100 μmol m−2 s−1, 30 s) was superimposed. Fc was added to a final concentration of 10 µM. The phosphorylation was terminated by mixing the guard cell protoplasts with trichloroacetic acid. After precipitation, the protein was subjected to SDS-PAGE and blotted onto a nitrocellulose membrane. Antibodies against phot1, phot2, BLUS1, pSer348 BLUS1, H+-ATPase and pThr947 AHA2 were described previously (Kinoshita and Shimazaki 1999, Ueno et al. 2005, Takemiya et al. 2013a, Yamauchi et al. 2019). The band intensity was quantified using ImageJ 1.48 software (National Institutes of Health, Bethesda, MD, USA).
In vitro kinase assay
The glutathione S-transferase gene in pGEX-2T (GE Healthcare, Chicago, IL, USA) was replaced with a His-tagged MBP coding sequence, and the resulting vector was designated as pPCS-MBP. The full-length BLUS1 sequence was inserted downstream of His-MBP and introduced into E. coli strain Rosetta 2DE3 (Novagen, Gibbstown, NJ, USA). The recombinant His-MBP-BLUS1 protein was purified using TALON metal affinity resin (Clontech, Mountain View, CA, USA). The in vitro kinase assay was performed in 20 mM HEPES-NaOH (pH 7.4), 5 mM MgCl2, 5 mM MnCl2, 3.3 µM ATP, 20 µCi [γ-32P] ATP (3000 Ci mmol−1; PerkinElmer, Waltham, MA, USA) and 5 µg His-MBP-BLUS1 for 3 h at 15°C. The phosphorylation was detected using Typhoon FLA 9500 (GE Healthcare).
In vitro phosphatase assay
Full-length type one protein phosphatase 4 (TOPP4) was subcloned into pGEX-2T (GE Healthcare), and the recombinant protein was prepared as described previously (Takemiya et al. 2006). PP1 phosphatase activity was measured using 32P-labeled myelin basic protein as a substrate (Takemiya et al. 2013b).
FDA staining
The viability of guard cells treated with acrolein was examined using FDA. Epidermal tissues were incubated in stomatal opening buffer under RL (50 μmol m−2 s−1) and BL (10 μmol m−2 s−1) for 2 h at 24°C, and then 2.5 µM FDA was loaded for 20 min in darkness. Acrolein was applied 30 min before light illumination. For FDA staining of the guard cell protoplasts, the protoplasts were incubated in 0.4 M mannitol and 1 mM CaCl2 in the presence of acrolein for 20 min at 24°C under RL (300 μmol m−2 s−1) and then 2.5 µM FDA was loaded for 10 min in darkness. After washing to remove excess dye, the fluorescence was visualized using a confocal laser scanning microscope (Olympus, FV-1200, Tokyo, Japan). The wavelengths of excitation and emission were 473 and 485–585 nm, respectively.
Supplementary Data
Supplementary data are available at PCP online.
Data Availability
The data underlying this article will be shared on reasonable request to the corresponding author.
Funding
Japan Society for the Promotion of Science KAKENHI (JP20H05420, JP21H02511, JP21H05665 and JP22H04726 to A.T.; JP20H03278 to J.M.); Japan Foundation for Applied Enzymology (to A.T.); Yamaguchi University Project for Formation of the Core Research Project (to A.T. and J.M.).
Disclosures
The authors have no conflicts of interest to declare.