-
PDF
- Split View
-
Views
-
Cite
Cite
Daniel A Careno, Soledad Perez Santangelo, Richard C Macknight, Marcelo J Yanovsky, The 5ʹ-3ʹ mRNA Decay Pathway Modulates the Plant Circadian Network in Arabidopsis, Plant and Cell Physiology, Volume 63, Issue 11, November 2022, Pages 1709–1719, https://doi.org/10.1093/pcp/pcac126
- Share Icon Share
Abstract
Circadian rhythms enable organisms to anticipate and adjust their physiology to periodic environmental changes. These rhythms are controlled by biological clocks that consist of a set of clock genes that regulate each other’s expression. Circadian oscillations in messenger RNA (mRNA) levels require the regulation of mRNA production and degradation. While transcription factors controlling clock function have been well characterized from cyanobacteria to humans, the role of factors controlling mRNA decay is largely unknown. Here, we show that mutations in SM-LIKE PROTEIN 1 (LSM1) and exoribonucleases 4 (XRN4), components of the 5ʹ-3ʹ mRNA decay pathway, alter clock function in Arabidopsis. We found that lsm1 and xrn4 mutants display long-period phenotypes for clock gene expression. In xrn4, these circadian defects were associated with changes in circadian phases of expression, but not overall mRNA levels, of several core-clock genes. We then used noninvasive transcriptome-wide mRNA stability analysis to identify genes and pathways regulated by XRN4. Among genes affected in the xrn4 mutant at the transcriptional and posttranscriptional level, we found an enrichment in genes involved in auxin, ethylene and drought recovery. Large effects were not observed for canonical core-clock genes, although the mRNAs of several auxiliary clock genes that control the pace of the clock were stabilized in xrn4 mutants. Our results establish that the 5ʹ-3ʹ mRNA decay pathway constitutes a novel posttranscriptional regulatory layer of the circadian gene network, which probably acts through a combination of small effects on mRNA stability of several auxiliary and some core-clock genes.
Introduction
The circadian clock is an endogenous time-keeping mechanism found in organisms as diverse as bacteria, plants and animals (Young and Kay 2001). The clock generates biological oscillations known as circadian rhythms that regulate many aspects of an organism’s behavior, physiology and development. The clock helps organisms to cope with daily changes in the environment, increasing their fitness (Dodd et al. 2005, Yerushalmi and Green 2009). In Arabidopsis thaliana plants, the circadian clock regulates more than 30% of gene expression (Romanowski et al. 2020). Genes regulated by the circadian clock are essential to many important physiological processes, including growth, flowering, metabolic regulation and stress responses (Greenham and McClung 2015).
The Arabidopsis central oscillator is a gene network based on multiple interconnected feedback loops, in which a set of core-clock genes acting as transcriptional repressors and activators regulate each other’s expression, as well as the expression of thousands of clock-controlled genes (CCGs; Nakamichi 2020). At dawn, LATE ELONGATED HYPOCOTYL (LHY) and CIRCADIAN CLOCK ASSOCIATED 1 (CCA1)—two MYB domain-containing transcription factors—reach their peak of expression and repress their own transcription as well as that of the afternoon and evening genes comprised in the PSEUDO-RESPONSE REGULATOR (PRR) family and the Evening Complex (EC) (Nagel et al. 2015). Later in the morning, the coactivators NIGHT LIGHT-INDUCIBLE AND CLOCK-REGULATED GENE 1 (LNK1) and LNK2 interact with the single MYB transcriptional activators REVEILLE 8 (RVE8), RVE6 and RVE4 (Xie et al. 2014, Pérez-García et al. 2015) and promote the expression of PRR5, TIMING OF CAB EXPRESSION 1 (TOC1, also known as PRR1) and the EC member EARLY FLOWERING 4 (ELF4). Throughout the day, the members of the PRR family, starting with PRR9 and ending with TOC1, reach their respective peak of expression and sequentially suppress the expression of LHY and CCA1. At night, ELF4, ELF3 and LUX ARRHYTHMO (LUX) associate forming the EC. The EC maintains the repression of the CCA1, LHY, PRR9, PRR5 and LUX itself. At the end of the night, CCA1 and LHY repress the EC and the cycle starts again.
Increasing evidence indicates that besides the transcriptional control of the clock gene network, posttranscriptional mechanisms also play a key role in modulating the function of core-clock genes (Beckwith and Yanovsky 2014). However, while we know in detail the factors regulating the circadian network at the transcriptional level, we are only starting to identify and characterize the factors and pathways controlling this network at the posttranscriptional level.
Alternative splicing (AS), alternative polyadenylation, nonsense-mediated decay (NMD), N6 methyladenosine (m6A) modification and nuclear export of messenger RNAs (mRNAs) are among the posttranscriptional mechanisms that regulate circadian clock gene expression (Beckwith and Yanovsky 2014). For example, TOC1, ELF3, CCA1, LHY, PRR7 and RVE8 are some of the clock genes undergoing temperature-regulated AS. NMD controls the levels of TOC1 and ELF3 mRNA (James et al. 2012, Kwon et al. 2014), and the light perceived by cryptochromes controls m6A deposition in CCA1 mRNA (Wang et al. 2021). Several mutants involved in the nuclear exportation of mRNA are defective in clock function (Ziemienowicz et al. 2003, MacGregor et al. 2013, de Leone et al. 2020). In addition, splicing factors or modulators that impact clock gene expression and function have also been characterized. Indeed, we previously described that mutations in two splicing factors, SM-LIKE PROTEIN 4 (LSM4) and LSM5, which are part of the LSM2–LSM8 nuclear spliceosome complex, affect clock function in Arabidopsis, most likely by regulating the AS of a subset of core-clock genes (Perez-Santángelo et al. 2014). LSM4 and LSM5 are also part of a cytoplasmic complex composed of seven LSM proteins (LSM1–LSM7). LSM1 is the key distinguishing component of the LSM1–LSM7 cytoplasmic complex, which is localized in processing bodies (p-bodies) and is involved in mRNA decapping followed by 5ʹ-3ʹ mRNA degradation (Golisz et al. 2013). There are two LSM1 homolog genes in the Arabidopsis genome, LSM1A and LSM1B. The single lsm1a and lsm1b mutants do not show phenotypic alterations, but the lsm1a lsm1b (lsm1a1b) double mutant displays severe growth defects (Golisz et al. 2013). Since LSM4 and LSM5 are part of both the LSM1–LSM7 decapping complex and the LSM2–LSM8 spliceosomal complex, their circadian phenotype could be due, at least in part, to their effect on mRNA decay.
High-amplitude mRNA oscillations, such as those observed for core-clock genes and several clock-controlled output genes, require the regulation of both mRNA stability and mRNA synthesis. Indeed, in a study seeking to identify unstable mRNAs using microarrays, Gutierrez et al. (2002) showed that genes controlled by the circadian clock are particularly unstable. Also, the clock-controlled CCR-LIKE and SENESCENCE-ASSOCIATED GENE 1 transcripts are differentially regulated at the level of mRNA stability at different times of day (Lidder et al. 2005). Furthermore, light modulates CCA1 mRNA stability, and this contributes to clock entrainment (Yakir et al. 2007, Wang et al. 2021).
Two main mRNA decay pathways shape transcript stability. Both of these pathways begin with the conversion of polyadenylated to oligoadenylate mRNA through deadenylation or shortening of the poly-A tail. Then, mRNAs can be the substrates of the 3ʹ-5ʹ decay pathway and be degraded by the highly conserved multi-subunit complex called exosome. Alternatively, the 5ʹ cap can be removed, and decapped transcripts can be degraded by 5ʹ-3ʹ exoribonucleases (XRNs) in the cytoplasm (reviewed in Zhang and Guo 2017). In Neurospora, a role for the catalytic component of the exosome complex in the control of circadian rhythms was described several years ago (Guo et al. 2009). Whether the cytoplasmic 5ʹ-3ʹ mRNA decay pathway controls clock function in eukaryotic organisms has yet to be shown.
The Arabidopsis genome encodes three XRNs: the nuclear XRN2 and XRN3, which are involved in the processing of several classes of nuclear RNAs and transcription termination, and the cytoplasmic XRN4, which is the functional homolog of yeast and mammalian XRN1 and ortholog of the nuclear XRN2/RAT1 (Kastenmayer and Green 2000). The XRN4’s main role is mRNA degradation in the cytoplasm, which must be preceded by decapping or endonucleolytic cleavage. Mutants for XRN4 were independently isolated in two screening for mutants insensitive to ethylene (Van Der Straeten et al. 1993, Ecker 1995), which were later associated with the 5ʹ-3ʹ XRN4 gene (Olmedo et al. 2006, Potuschak et al. 2006). A triple mutant for the three Arabidopsis XRN genes, xrn2, xrn3 and xrn4, has been shown to have a long-period phenotype for the expression of several core-clock genes (Litthauer et al. 2018). However, whether the circadian phenotype is specifically associated with loss of XRN4 function, the only 5ʹ-3ʹ XRN involved in cytoplasmic mRNA decay, is not known.
Mutants for XRN4 show an accumulation of deadenylated uncapped transcripts and 3ʹ mRNA fragments that are subject to NMD (Nagarajan et al. 2019). It was also found that poly-A+ mRNA targets of XRN4 are subject to co-translational decay, and this modulates their translation efficiency during plant development (Carpentier et al. 2020). Although targets for XRN4 have been discovered, the overall overlap of target genes between the different studies is low (Rymarquis et al. 2011, Nagarajan et al. 2019, Carpentier et al. 2020). This low overlap could be due to differences in XRN4 activity at different developmental stages.
Here, we show that mutations in LSM1A/LSM1B and XRN4, which affect decapping and 5ʹ-3ʹ mRNA degradation, respectively, lengthen the circadian period in Arabidopsis. We observed changes in the circadian phases rather than in the overall mRNA levels of canonical core-clock genes in xrn4 mutants compared to wild-type (WT) plants. By performing a noninvasive genome-wide analysis of posttranscriptional regulation in xrn4 mutants and WT plants, we found an enrichment of high-amplitude clock controlled genes (CCGs) among the differentially expressed genes (DEGs), suggesting a role for 5ʹ-3ʹ XRN4 in the control of mRNA stability of clock-regulated transcripts. Indeed, some members of the BBX gene family that control clock function showed enhanced mRNA stability in the xrn4 mutant and could mediate, in part, the effects of XRN4 on the clock. Although the exact molecular mechanisms through which mRNA decay affects the biological clock remain elusive, our results support an important role for the 5ʹ-3ʹ mRNA decay pathway controlled by LSM1 and XRN4 in the fine-tuning of circadian oscillations in Arabidopsis plants.
Results
Lack of Arabidopsis mRNA decay components LSM1 and XRN4 alters circadian rhythms
The LSM1-7 cytoplasmic complex is involved in mRNA decapping followed by 5ʹ-3ʹ mRNA degradation. LSM1 is the distinguishing component of the cytoplasmic LSM1–LSM7 complex and, in Arabidopsis, is encoded by two genes, LSM1A and LSM1B. Leaf movement analysis showed no circadian phenotypes in either single lsm1a or lsm1b mutants or homozygous lsm1a and heterozygous lsm1b mutant plants (Supplementary Fig. S1). Homozygous lsm1a1b mutants, like lsm4 (Perez-Santángelo et al. 2014), display severe growth defects compared to WT plants (Supplementary Fig. S1C). Therefore, circadian rhythms in lsm1a1b mutants were evaluated using bioluminescent reporters rather than leaf movement assays. For this, we transformed plants that were homozygous for lsm1a and heterozygotes for lsm1b with two different circadian bioluminescent reporter genes, pCCA1:LUC and pCCR2:LUC, and analyzed circadian rhythms in homozygous lsm1a1b plants in the next generation. The circadian period of bioluminescence was lengthened, and the phase was delayed in lsm1a1b homozygous mutants relative to WT plants for both pCCA1:LUC and pCCR2:LUC reporters, suggesting that the 5ʹ-3ʹ mRNA decay pathway controls clock function in Arabidopsis (P < 0.001; Fig. 1, Supplementary Fig. S2). We then evaluated if LSM1a and LSM1b mRNA levels were under the control of the circadian clock, using publicly available data sets. We found that LSM1b displays circadian oscillations in the DIURNAL data set (Mockler et al. 2007), but none of the LSM1 genes cycle in the recently published RNA-seq circadian time-course (Romanowski et al. 2020), suggesting weak control of LSM1 expression by the circadian oscillator.
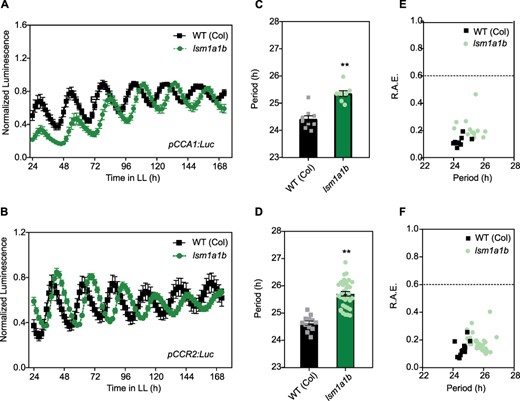
Mutations in the plant LSM1a/LSM1b genes affect the clock. The expression of the morning core-clock reporter pCCA1:LUC (A) and afternoon clock output reporter pCCR2:LUC (B) was measured for 7 d in LL after entrainment under LD conditions. WT (Col-0), WT Columbia ecotype (squares); lsm1ab mutant (circles). Bioluminescence was recorded every 2 h over the 7 d and analyzed by FFT-NLLS. (C, D) Period values and (E, F) relative amplitude error (R.A.E.) were plotted for each genotype. Error bars represent the mean with range. **P < 0.001 (Student’s t-test).
After removal of the 5ʹ 7mG cap by the decapping complex, the unprotected 5ʹ end of mRNAs can be degraded by specific 5ʹ-3ʹ XRNs. In Arabidopsis, XRN4 is the only 5ʹ-3ʹ XRN with a cytoplasmic localization. To analyze the effect of cytoplasmic mRNA decay on clock function, we characterized circadian rhythms in xrn4-3 and xrn4-5 single mutants, two strong transfer DNA insertion alleles expressing low levels of truncated XRN4 mRNAs (Supplementary Fig. S3, Souret et al. 2004, Potuschak et al. 2006). Using a bioluminescent reporter, we found that the circadian period of TOC1 expression was lengthened in both xrn4 alleles relative to WT plants (25.59 ± 0.52 h for xrn4-3 compared to 24.14 ± 0.42 h for WT plants; P < 0.001; Fig. 2A–C; and 25.87 ± 0.08 h for xrn4-5 vs 25.17 ± 0.26 h for the WT; P < 0.05; Supplementary Fig. S4). We also observed a longer circadian period for the rhythm of leaf movement in the two different xrn4 mutants compared to WT plants (25.27 ± 0.33 h for xrn4-3, 24.76 ± 0.29 h for xrn4-5 and 24.00 ± 0.26 h for WT plants; P < 0.001; Fig. 2D–F). No differences in the circadian phase of leaf movement were observed between both xrn4 alleles and WT plants, and a slight phase delay was observed for pTOC1:LUC in xrn4-3 vs WT (Supplementary Fig. S5). Altogether, these results provide compelling evidence that the canonical 5ʹ-3ʹ mRNA decay pathway, which includes the LSM1–LSM7 complex and the 5ʹ-3ʹ XRN4, plays an important role in the control of the circadian period in Arabidopsis plants.
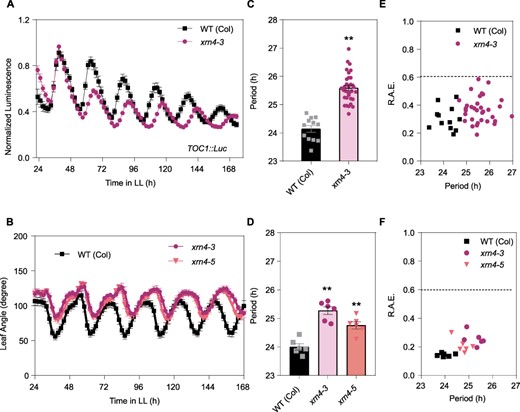
Mutations in the exoribonuclease 5ʹ-3ʹ XRN4 cause a delay in the clock period. (A) Expression of the night core-clock reporter pTOC1:LUC was measured for 7 d in LL after entrainment under LD conditions. WT (Col-0), WT Columbia ecotype (squares); xrn4-3 mutant (circles). (B) Circadian rhythm of leaf movement. Leaf angles were measured for the first pair of leaves in seedlings entrained under LD conditions, and plants were then transferred to LL. WT (Col-0) (squares). xrn4-3 mutant (circles, n = 6); xrn4-5 mutant (triangle). (C, D) The period length of bioluminescence and leaf movement rhythms was estimated by the fast Fourier transform nonlinear least squares (FFT-NLLS) test. (E, F) Relative amplitude error (R.A.E.) were plotted for each genotype. Error bars represent the mean with range. **P < 0.001 (Student’s t-test).
Loss of Arabidopsis XRN4 alters the timing of expression of core-clock genes
To further study the effect of the mRNA decay pathway on clock function, and since XRN4 is the only cytoplasmic enzyme involved in the 5ʹ-3ʹ mRNA decay, we decided to analyze the expression of several core-clock genes in the xrn4-3 mutant. For this, plants were grown under light/dark cycles for 10 d and then transferred to continuous light (LL) for 2 d. Samples were collected every 4 h for 1 d. Phase changes were observed for most of the core-clock genes evaluated in xrn4-3 mutants compared to the WT plants. For the morning genes LHY and CCA1, reduced and delayed expression were observed in xrn4-3 mutants compared to WT plants, resulting in the loss of the sharp morning peak (P < 0.05; Fig. 3A, B). In the case of the midday/evening genes PRR9, PPR5 and ELF3, a clear 4-h phase delay was also observed in xrn4-3 compared to WT plants (Fig. 3C, E, H), resulting in a higher and broader peak of expression for PRR5 in xrn4-3 than in WT plants. For TOC1, no differences in the phase of expression were found between genotypes, but a higher peak of expression was detected in xrn4-3 (Fig. 3F). For the morning gene RVE8, we observed slightly higher mRNA levels in the xrn4 mutant compared to WT plants during subjective daytime (P < 0.05; Fig. 3G). Last, no differences in PRR7 expression were observed between xrn4-3 mutants and WT plants under these conditions (Fig. 3D). In summary, most genes showed the loss of a sharp peak of expression, resulting in a wider peak of expression during the third day under continuous light conditions, which is compatible with the approximately 1-h period difference observed between xrn4-3 mutants and WT plants. Strong differences in overall mRNA levels were not observed for most clock genes between genotypes, although a slight enhancement in mRNA levels was observed for PRR5 and RVE8 in the decaying phase of expression. These results, taken together, suggest that the XRN4 effect on the clock could be in part the result of subtle effects on mRNA levels of several clock genes, rather than the result of a strong effect on the mRNA levels of a few target genes.
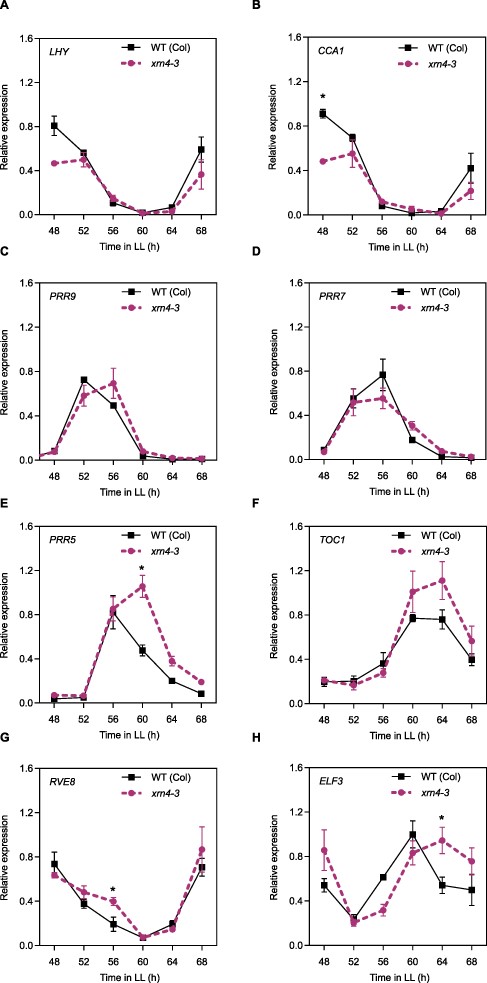
Mutations in the exoribonuclease 5ʹ-3ʹ XRN4 affect core-clock expression patterns. Gene expression of core-clock genes measured by RT-qPCR in plants entrained under 12-h light/12-h dark cycles, before being moved to LL for 3 d. WT (Col-0) (solid line, squares), xrn4-3 mutant (dashed line, circles). (A) LHY, (B) CCA1, (C) PRR9, (D) PRR7, (E) PRR5, (F) TOC1, (G) RVE8 and (H) ELF3. Data are the average of three biological replicates normalized to the maximum value. Error bars represent SEM. *P < 0.05 (Student’s t-test).
Flowering time is affected in the xrn4 mutant
To study if flowering time, an output of the circadian clock, was affected by mutations in the cytoplasmic 5ʹ-3ʹ XRN, xrn4 mutant plants were grown under inductive photoperiodic conditions [long days (LDs), 16-h light and 8-h dark cycles]. We observed a late flowering phenotype for xrn4, evaluated as the number of rosette leaves at bolting. The xrn4-3 mutant allele flowered with 22.58 ± 1.03 leaves and the xrn4-5 allele with 20.0 ± 0.74 leaves, both compared to 15.83 ± 0.56 leaves in WT plants (P < 0.05; Supplementary Fig. S6). The late flowering phenotype observed for these mutants under long-day (LD) conditions is consistent, with this defect being, at least in part, the result of the longer circadian period of the mutants, since day-length perception depends on the coincidence of light with a sensitive phase of the circadian cycle (Roden et al. 2002, Yanovsky and Kay 2003, Niwa et al. 2009).
Genome-wide analysis of transcriptional and posttranscriptional regulation by XRN4
Differences in the circadian phase have been shown to strongly affect the analysis of differential gene expression between the WT and clock mutant plants, even in mutants with mild circadian period alterations (Hsu and Harmer 2012). Our finding that xrn4 mutants display an approximately 1 h longer circadian period phenotype compared to WT plants suggests that many of the mRNA previously identified as differentially accumulated in xrn4 mutants could have simply been selected because of differences in their phase of expression, rather than differences in their overall mRNA levels. Here, we performed a transcriptomic analysis on total RNA using a protocol designed specifically to explore the effects of xrn4 mutation on overall mRNA levels and minimize the possible impact of period length differences between genotypes. For this, we grew plants for 15 d under LD conditions and transferred them to continuous light conditions. Then we harvested samples every 4 h, from Circadian Time 2 (CT2, i.e. 2 h after lights on under continuous light) to CT22. Finally, to conduct a comparative transcriptomic analysis, we pooled equal amounts of samples obtained at different times of day into one sample for each biological replicate for each genotype. The pooling of samples harvested at different times of the day during a circadian cycle should attenuate differences in mRNA levels caused by possible changes in the phase of expression of CCGs between genotypes and, therefore, should allow the identification of genes showing different overall mRNA levels between xrn4 mutants and WT plants.
The analysis of the transcriptomic data revealed 211 DEGs between WT plants and xrn4 mutants (Fig. 4A; Supplementary Dataset S1). The majority of these genes, 80% (167/211), were upregulated in the mutant compared to WT plants, as expected for possible targets of the 5ʹ-3ʹ mRNA decay pathway (Supplementary Dataset S1) (Nagarajan et al. 2019, Carpentier et al. 2020). Interestingly, the group of genes upregulated in xrn4 mutants showed a 2-fold statistically significant enrichment in high-amplitude CCGs (top 500 clock controlled genes sorted by amplitude, Romanowski et al. 2020, Supplementary Fig. S7A). As expected, gene ontology (GO) analysis of DEGs also showed enrichment in terms that correspond to previously reported XRN4-regulated processes (Fig. 4B, Supplementary Dataset S3A). This includes responses to the hormones ethylene and auxin, water deprivation and hypoxia (Van Der Straeten et al. 1993, Ecker 1995, Weber et al. 2008, Hirsch et al. 2011, Windels and Bucher 2018).
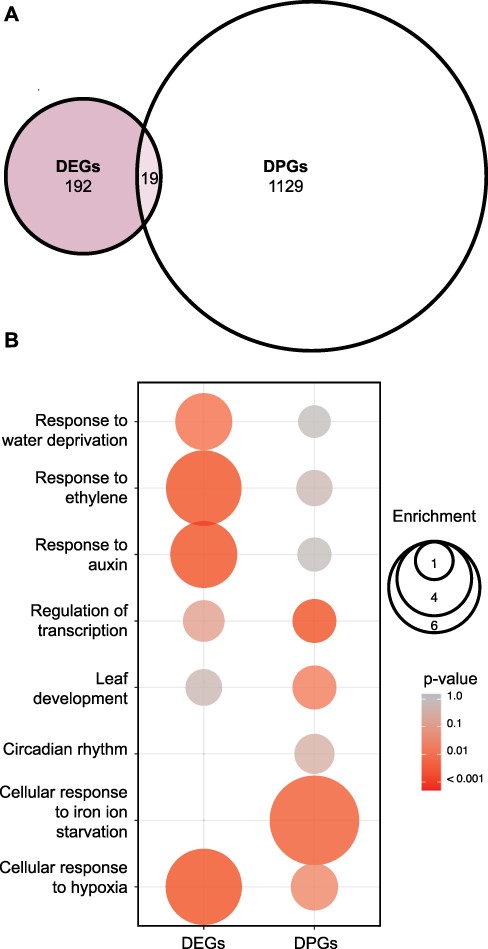
Global analysis of differentially regulated genes at the transcriptional and posttranscriptional level in the 5ʹ-3ʹ XRN, xrn4-3 mutant. (A) Overlap between DEGs and DPGs between WT (Col-0) and xrn4-3 (EF 1.5, P = 0.05). (B) EF and P-value of selected GOs among DEGs and DPGs. The color gradient represents adjusted P-values and the differences in bubble size correlate with the EF.
Next, to investigate the impact of xrn4 mutations on mRNA stability, we performed a noninvasive analysis of differential posttranscriptional regulation using the R package INference of Synthesis, Processing and dEgradation rates from Transcriptomic data (INSPEcT) (Furlan et al. 2020). Briefly, INSPEcT detects changes in posttranscriptional regulation by comparing the relationship between intronic and exonic reads, which are used as proxies for pre-mRNA and mature mRNA. Thus, differences in mRNA degradation rates between genotypes can be inferred from changes in the ratio between premature and mature RNA for each gene. Using this approach, we found 1,148 differentially posttranscriptionally regulated genes (DPGs) in xrn4 compared to WT plants (Fig. 4A; Supplementary Dataset S2).
No canonical core-clock genes were identified among the 1,148 genes differentially regulated at the posttranscriptional level for the xrn4 mutants. An enrichment in GO terms related to phenotypes previously reported for xrn4 mutants, such as response to iron ion or root formation, was observed (Fig. 4B; Supplementary Dataset S3B) (Nagarajan et al. 2019, Balparda et al. 2021). The minor and subtle effects observed at the posttranscriptional level on core-clock genes in xrn4 mutants compared to WT plants using INSPEcT are consistent with the rather similar mRNA decay rates observed for selected core-clock genes in xrn4 mutants and WT plants after the addition of the transcriptional inhibitor cordycepin (Supplementary Fig. S8). This suggests that XRN4 might control clock function, in part, through very subtle effects on mRNA decay rates of some core-clock genes. Alternatively, or in addition, XRN4 could be a controlling clock function through posttranscriptional regulation of auxiliary clock factors rather than canonical core-clock components. Indeed, among the DPGs identified in xrn4 mutants, we found five B-BOX domain-containing genes, BBX2 (COL1), BBX3 (COL2), BBX11, BBX15 and BBX19. These genes display high-amplitude circadian rhythms in mRNA levels, and some of them are known to control circadian period length (Ledger et al. 2001). We analyzed the mRNA decay rates of some of these candidate genes in WT and xrn4 plants, with the cordycepin-induced transcription arrest assay, and observed a tendency toward slower mRNA decay rates for the BBX3 gene in the mutant plants (Fig. 5A). For the BBX15 gene, we found weak stabilization similar to that observed for ELF3 and PRR5 (Fig. 5B, Supplementary Fig. S8C, D).
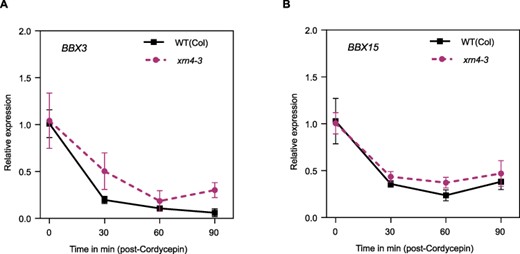
mRNA decay rates for the candidates BBX3 and BBX15 in xrn4-3. Analysis of mRNA decay with a cordycepin-induced transcription arrest assay. Plants were grown for 10 d in continuous light, and samples were taken every 30 min from 0 to 90 min. (A) BBX3 and (B) BBX15.
An examination of the ratio of mature (M) to premature (P) mRNA levels (M/P) in genes that were characterized as DPGs in xrn4 mutant plants revealed that 90% of the genes (972 out of 1,148) exhibited greater M/P ratios in xrn4 mutants than in WT plants (Supplementary Fig. S9). This is the expected behavior for true targets of the XRN4, since reduced degradation of an XRN4 target in xrn4 mutants should result in higher M/P ratios. This trend extends to the DPGs included in the GO term circadian rhythms (Supplementary Fig. S10). Altogether, these results support the conclusion that mRNA decay is an important posttranscriptional regulatory layer of the Arabidopsis circadian network.
Discussion
Although the importance of posttranscriptional regulation in shaping circadian oscillations of gene expression is well recognized, the role of mRNA decay has been largely unexplored (Beckwith and Yanovsky 2014, Lück et al. 2014, Romanowski and Yanovsky 2015, Mateos et al. 2018). So far, only one study has revealed a connection between the 3ʹ-5ʹ mRNA decay machinery and the control of circadian rhythms in Neurospora (Guo et al. 2009). Here, we show for the first time the evidence that the 5ʹ-3ʹ mRNA decay pathway is also involved in fine-tuning circadian rhythms.
In previous work, the splicing modulator PROTEIN ARGININE METHYLTRANSFERASE 5 (PRMT5) was found to affect clock function. Among its methylation targets, there are core spliceosomal proteins, whose activity is necessary for the correct splicing of PRR9 mRNA (Deng et al. 2010, Sanchez et al. 2010). One of the PRMT5 splicing-related target proteins is indeed LSM4, which is required for the correct clock function (Perez-Santángelo et al. 2014). LSM4 and LSM5—which are also required for clock function (Perez-Santángelo et al. 2014)— not only are involved in nuclear RNA processing but also modulate mRNA decapping in the cytoplasm. So, we asked whether the circadian phenotypes could be due, in part, to the effects of LSM4 and LSM5 on mRNA decay. To address this, we took advantage of the fact that LSM1 is the distinguishing component of the cytoplasmic LSM1–LSM7 decapping complex that modulates mRNA decay since LSM1 is not present in the LSM2–LSM8 nuclear spliceosomal complex. We found that mutations in the Arabidopsis LSM1A/LSM1B genes resulted in a long circadian period phenotype (Fig. 1). In human cells, ribosome profiling revealed that the LSM1 protein is rhythmically translated and that cytoplasmic p-body formation was rhythmic in these cells (Jang et al. 2015), which could be contributing rhythmicity to the 5ʹ-3ʹ mRNA decay process. The lsm4 and lsm5 long-period phenotypes (∼2 and ∼4 h longer in the mutants than in WT plants; Perez-Santángelo et al. 2014) are larger than the period phenotype observed in lsm1a1b mutants (∼1 h longer than in the WT). This is consistent with an additive effect of both the splicing and the mRNA decay pathways on the circadian period of lsm4 and lsm5 mutant plants.
The lsm1a1b double mutant showed a lethal phenotype at the seedling stage, similar to that observed in mutants of other genes involved in mRNA decapping (Xu et al. 2007). In contrast, xrn4 mutants are completely viable and display only mild morphological phenotypes (Olmedo et al. 2006). This suggests that the accumulation of capped mRNAs might be more critical for plant survival than that of uncapped mRNAs. In the future, it would be interesting to analyze the overlap between the substrates of the decapping machinery and those of XRN4 to gain further understanding of the extent of the connection between mRNA decapping and 5ʹ-3ʹ mRNA degradation.
Supporting a role for the canonical cytoplasmic mRNA decay pathway in the control of clock function, we found that mutations in XRN4 cause an approximately 1-h delay in the circadian period of the rhythm of leaf movement and TOC1 promoter activity (Fig. 2). We also observed a shift in the expression profiles of the majority of core-clock genes analyzed under free-running conditions (Fig. 3). Particularly for ELF3 and PRR5, the approximately 4-h phase delay, widening of the peak of expression observed on the third day under continuous light, is consistent with the approximately 1-h increase in period length present in xrn4 mutants and could be interpreted as the result of slower mRNA decay of these clock genes.
Several genome-wide analyses of XRN4 effects on the Arabidopsis transcriptome have been conducted before (Rymarquis et al. 2011, Nagarajan et al. 2019, Carpentier et al. 2020). The transcriptomic analysis of mutant plants with circadian period phenotypes requires special care to avoid identifying genes affected in their circadian phases of expression, rather than in their overall mRNA levels, which happens when samples collected at a single time point during a daily cycle are evaluated (Hsu and Harmer 2012). This prompted us to conduct a new genome-wide analysis to study the overall changes in mRNA levels in the Arabidopsis xrn4 mutants by comparing mRNAs from samples collected throughout a full circadian cycle, rather than at a single time point. This approach revealed 167 genes whose overall mRNA levels were higher in xrn4 mutants than in WT plants. Several of these genes were previously reported to be regulated by XRN4, as expected. We observed enrichment in genes associated with auxin and ethylene signaling pathways that are already known to be modulated by XRN4. Indeed, the role of XRN4 (ein5, ethylene insensitive 5) in the regulation of ethylene responses is well known (Olmedo et al. 2006, Potuschak et al. 2006). Furthermore, ethylene emissions display circadian oscillations (Thain et al. 2004), and the ein3 mutant, which is also involved in the ethylene pathway, presents a long circadian period phenotype (Haydon et al. 2017). Therefore, it is possible that at least part of the xrn4 circadian phenotypes could be associated with the role of XRN4 in modulating the ethylene pathway. It is important to mention that the precise mechanisms through which ethylene signaling controls the clock are not yet known.
The DEG analysis on overall mature mRNAs showed no core-clock genes among those upregulated in xrn4 mutants, suggesting that XRN4 effects on mRNA levels of core-clock genes are subtle, as observed in our real-time quantitative reverse transcription (RT-qPCR) time-course analysis. Interestingly, we did find a similar and significant enrichment of genes displaying high-amplitude circadian oscillations in mRNA levels among those upregulated in the xrn4 mutant (Supplementary Fig. S7). Finally, using the same RNA-seq data set generated here, we performed an analysis of XRN4 effects on posttranscriptional regulation. For this, we used the Bioconductor package INSPEcT, a noninvasive approach that allows the detection of changes in transcription and decay rates of mRNA levels by comparing the relationship between intronic and exonic reads from RNA-seq data generated using total RNA (Furlan et al. 2020). This analysis showed 1,148 DPGs for xrn4 (Fig. 4A). Noteworthy, we found more DPGs than DEGs in our RNA-seq data set, consistent with a predominant role for XRN4 in posttranscriptional regulation. Many DPGs were not identified as DEGs (Fig. 4; Supplementary Datasets S1 and S2). This might be due to a buffering system involving interactions between posttranscriptional and transcriptional regulatory mechanisms. For example, the deletion of XRN1 in yeast has been shown to alter transcription rates (Braun and Young 2014). On the other hand, xrn4 mutant plants show an increase in posttranscriptional gene silencing (Gazzani et al. 2004).
Among new genes identified as potential targets of XRN4 in our analysis, we focused our attention on the family of BBX transcription factors, due to the appearance of multiple of its members among DEGs and DPGs (Supplementary Datasets S1 and S2). For example, BBX15, BBX2 (COL1) and BBX3 (COL2) were identified as DPGs in the xrn4 mutant, and the overexpression of COL1 and COL2 alters the circadian period (Ledger et al. 2001). Moreover, we observed a tendency toward a slower mRNA decay rate for BBX3 after the cordycepin-induced arrest of transcription in the xrn4 mutant (Fig. 5A). Last, BBX19 was also identified as a DPG in xrn4 and has recently been found to interact with the PRR family of core-clock genes forming a transcriptional regulatory complex that acts in fine-tuning the circadian clock (Yuan et al. 2021). Whether part of the xrn4 circadian phenotype is due to altered regulation of these BBX genes remains to be determined.
In conclusion, circadian regulation of mRNA levels requires regulation of both mRNA synthesis and mRNA degradation, but the specific mRNA decay factors involved in the control of circadian rhythms in multicellular organisms were not known. Here, we provide strong evidence that LSM1 and XRN4, components of the 5ʹ-3ʹ mRNA decay pathway, contribute to fine-tuning the Arabidopsis circadian clock. This effect on circadian clock function is likely the result of a combination of small effects on mRNA stability of some auxiliary clock genes, such as BBX gene family members, and some core-clock genes, such as PRR5. Further studies are needed to understand the precise mechanisms through which the 5ʹ-3ʹ mRNA decay pathway regulates this key biological process.
Materials and Methods
Growth conditions
Plants were grown in pots containing a mixture of organic substrate, vermiculite and perlite [0.5:1:1 (vol/vol)] at 22°C under LDs (16-h light/8-h dark cycles), 12:12 (12-h light/12-h dark cycles) or continuous light (LL), depending on the experiment.
Plant material
The mutants used, including lsm1a (SALK_106536), lsm1b (SAIL_898_D06), xrn4-3 (SALK_014209) and xrn4-5 (SAIL_681_E01), were obtained from the Arabidopsis Biological Resource Center.
Circadian leaf movement analysis
For leaf movement analysis, plants were entrained under a 16-h light/8-h dark cycle, transferred to continuous white fluorescent light, and the position of the first pair of leaves was recorded every 2 h for 5–6 d using Image J software (imagej.nih.gov/ij/). Period estimates were calculated using Biological Rhythms Analysis Software System 3.0 (BRASS) (www.amillar.org) and analyzed using Fast Fourier transform nonlinear least squares (FFT-NLLS). The statistical analysis was done using a two-tailed Student’s t-test or one-way analysis of variance, depending on the case.
Flowering time analysis
For flowering time analysis, plants were grown under LDs (16-h light/8-h dark cycles) at a constant temperature of 22°C. Flowering time was estimated by counting the number of rosette leaves at the time of bolting. The statistical analysis was done using a two-tailed Student’s t-test.
Plant bioluminescence recording and data analysis
The seedlings were grown directly on Murashige and Skoog 0.8% agar supplemented with 1% sucrose in a 96-well plate. One seed was placed per well, and the plate was placed in darkness at 4°C for 3 d. Then seedlings were entrained under LDs (16-h light/8-h dark cycles). After 7 d, the entire plate was transferred to LL and temperature (22°C) conditions and placed in a luminometer (Berthold Technologies, Bad Wildbad, Germany) to measure bioluminescence emitted by each seedling every 2 h. After 5–6 d, an analysis of the data was done using the software Mikrowin 2000 (version 4.29) (Mikrotek Laborsysteme, Overath, Germany). The luminometer model used was the microplate reader Centro LB-960 (Berthold Technologies). Period estimates were calculated with Brass 3.0 and analyzed using FFT-NLLS. The statistical analysis was done using a two-tailed Student’s t-test.
Cordycepin treatment
Plants were grown in continuous light (LL) since germination, with no entrainment. Under LL, gene oscillations are flattened, and changes in overall expression can be seen no matter the time of the day. After 2 weeks, seedlings were transferred to flasks containing a buffer (1 mM 1,4-piperazinediethanesulfonic acid, pH 6.25, 1 mM sodium citrate, 1 mM KCl and 15 mM sucrose), and after a 30-min incubation, cordycepin (150 mg/l) was added and the samples were taken at 0, 30, 60 and 90 min. Total RNA was extracted using TRIzol reagent (Invitrogen) for quantitative RT-qPCR.
Quantitative RT-qPCR
For time-course analysis, 14-day-old plants were grown under 12-h light/12-h dark cycles and then transferred for 3 d to continuous white light at 22°C. Samples were collected every 4 h for 1 d. Total RNA was obtained from these samples using TRIzol reagent (Invitrogen). One microgram of RNA was treated with RQ1 RNase-Free DNase (Promega) and subjected to retro-transcription with Moloney Murine Leukemia Virus Reverse Transcriptase (Invitrogen) and oligodT according to the manufacturer’s instructions. Synthesized complementary DNAs were amplified with FastStart Universal SYBR Green Master (Roche) using the Mx3000P RT-PCR system (Agilent Technologies) cycler. The PP2A (AT1G13320) transcript was used as a housekeeping gene. Quantitative RT-PCR quantification was conducted using the standard curve method as described in the Methods and Applications Guide from Agilent Technologies. Primers used for amplification of each gene are described in Supplementary Table S1.
Growth conditions and protocol used for total RNA library preparation and high-throughput sequencing
For biological replicates of WT (Col-0), xrn4-3 seeds were sown onto Murashige and Skoog medium containing 0.8% (w/v) agarose, stratified for 3 d in the dark at 4°C and then grown at 22°C in LD conditions (16-h light/8-h dark cycles). After 2 weeks, plants were transferred to continuous light. During the first day of continuous light, whole seedlings were harvested every 4 h starting at CT = 2 h up to CT = 22 h. Total RNA was extracted with TRIzol (Invitrogen) following the manufacturer’s protocols. Samples were pooled across the time series before library preparation to estimate the difference in absolute RNA levels among genotypes, rather than differences resulting from alterations in the timing of expression of each gene.
For library preparation, ribosomal RNA (rRNA) was removed by Ribo-ZeroTM Magnetic Kit (Illumina), and the rRNA-free residue was cleaned up by ethanol precipitation. Subsequently, sequencing libraries were generated using the Directional NEBNext Ultra RNA Library Prep Kit (Novogene, Singapore). Samples were sequenced on the Illumina NovaSeq 6,000 platform, providing 150-bp paired-end reads (Novogene, Singapore). On average, 108 million 150-nt paired-end reads were obtained for each sample.
Processing of RNA sequencing reads
Sequence reads were mapped to Arabidopsis thaliana TAIR10 (Lamesch et al. 2012) genome using STAR 2.7.0 (Dobin et al. 2013) with the following parameters: twopassMode Basic, outFilterMultimapNmax 2, outFilterType BySJout, outSJfilterReads Unique, sjdbOverhang 149, alignSJoverhangMin 6, alignSJDBoverhangMin 3, alignIntronMin 20 and alignIntronMax 5000.
Differential gene expression analysis
Before differential expression analysis, we discarded genes with fewer than 10 reads, on average, per condition (minRds ≥ 0.05). Differential gene expression was estimated using the ASpli package version 2.0.0 (Mancini et al. 2021) using custom R scripts, and the resulting P-values were adjusted using a false discovery rate (FDR) criterion (Benjamini and Hochberg 1995). In order to detect the subtle effects of XRN4, we filtered genes based on a low stringency FDR and log2-fold change. Genes with FDR values lower than 0.1 and an absolute log2-fold change greater than 0.58 were deemed differentially expressed. DEGs in the xrn4-3 mutant compared to WT (Col-0) can be found in Supplementary Dataset 1.
Differential gene posttranscriptional regulation analysis
DPGs were estimated using the R package INference of Synthesis, Processing and dEgradation rates from Transcriptomic data (INSPEcT) version 1.22.0 (Furlan et al. 2020). Expression was quantified from Binary Alignment Map files using the built-in function with default parameters. Genes with a log2-normalized expression lower than 0.25 were discarded. DPGs were determined using compareSteadyNoNascent() using a log2 distance threshold of 0.58. DPGs in the xrn4-3 mutant compared to WT (Col-0) can be found in Supplementary Dataset 2.
GO analysis
The assignment of GO terms for the DEGs and DPGs data sets was obtained using the Bioconductor packages topGO version 2.44.0 and org.At.tair.db version 3.13.0. An enrichment test was performed for the biological process category. P-values were obtained using the Fisher exact test. The enrichment factor (EF) was estimated as the ratio between the proportions of genes associated with a particular GO category present in the data set under analysis relative to the proportion of the number of genes in this category in the whole genome that have passed the expression filters. For choosing the GO terms plotted in Fig. 4, we ruled out categories with too few (less than 5) or too many (more than 1,500) genes, in order to avoid terms too specific or too general. In addition, we selected a few categories that had a significant P-value (<0.05) for enrichment among either DEGs or DPGs, which were associated with phenotypes previously reported for xrn4 mutants, plus the term circadian rhythms.
Overrepresentation analysis
Similar to the GO analysis, for the DEGs and DPGs data sets, we calculated an EF between these groups of genes and with the 500 CCGs with the biggest relative expression amplitude (Romanowski et al. 2020). The probability of each overlapping was determined using the hypergeometric probability formula.
Supplementary Data
Supplementary data are available at PCP online.
Data Availability
Sequencing data have been uploaded to the BioProject collection and are available under accession number PRJNA775638.
Accession numbers are LSM1a, AT1G19120; LSM1b, AT3G14080; XRN4, AT1G54490; PP2A, AT1G13320; CCA1, AT2G46830; LHY, AT1G01060; PRR9, AT2G46790; PRR7, AT5G02810; PRR5, AT5G24470; TOC1, AT5G61380; RVE8, AT3G09600 and ELF3, AT2G25930.
Funding
Agencia Nacional de Promoción Científica y Tecnológica of Argentina (PICT-2020-SERIEA-03390 to M.J.Y.); Trust Dean’s Bequest Round, University of Otago (to S.P.S); New Zealand Marsden Fund (to R.C.M.).
Author Contributions
D.A.C., M.J.Y. and S.P.S. conceived the project and designed the experiments; D.A.C. and S.P.S. carried out the experiments and analyzed the data; R.C.M. contributed to the genome-wide analysis; D.A.C., M.J.Y. and S.P.S. wrote the article and all authors approved the article for publication.
Disclosures
The authors have no conflicts of interest to declare.
References
Author notes
contributed equally to this work.