-
PDF
- Split View
-
Views
-
Cite
Cite
Natsumi Aoki, Songkui Cui, Satoko Yoshida, Cytokinins Induce Prehaustoria Coordinately with Quinone Signals in the Parasitic Plant Striga hermonthica, Plant and Cell Physiology, Volume 63, Issue 10, October 2022, Pages 1446–1456, https://doi.org/10.1093/pcp/pcac130
- Share Icon Share
Abstract
Orobanchaceae parasitic plants are major threats to global food security, causing severe agricultural damage worldwide. Parasitic plants derive water and nutrients from their host plants through multicellular organs called haustoria. The formation of a prehaustorium, a primitive haustorial structure, is provoked by host-derived haustorium-inducing factors (HIFs). Quinones, including 2,6-dimethoxy-p-benzoquinone (DMBQ), are of the most potent HIFs for various species in Orobanchaceae, but except non-photosynthetic holoparasites, Phelipanche and Orobanche spp. Instead, cytokinin (CK) phytohormones were reported to induce prehaustoria in Phelipanche ramosa. However, little is known about whether CKs act as HIFs in the other parasitic species to date. Moreover, the signaling pathways for quinones and CKs in prehaustorium induction are not well understood. This study shows that CKs act as HIFs in the obligate parasite Striga hermonthica but not in the facultative parasite Phtheirospermum japonicum. Using chemical inhibitors and marker gene expression analysis, we demonstrate that CKs activate prehaustorium formation through a CK-specific signaling pathway that overlaps with the quinone HIF pathway at downstream in S. hermonthica. Moreover, host root exudates activated S. hermonthica CK biosynthesis and signaling genes, and DMBQ and CK inhibitors perturbed the prehaustorium-inducing activity of exudates, indicating that host root exudates include CKs. Our study reveals the importance of CKs for prehaustorium formation in obligate parasitic plants.
Introduction
Plants that deprive other plants of nutrients are collectively called parasitic plants. Parasitic plants represent approximately 1% of angiosperms, including about 4,500 species, and the levels of host dependency and photosynthetic ability are varied among them (Yoshida et al. 2016). Those who cannot complete their life cycles without host plants in natural conditions are called obligate parasites, whereas those who can grow without host plants with their own photosynthetic activity are called facultative parasites. The obligate parasites can be further classified into hemi- and holoparasites for those with or without photosynthesis ability, respectively, while all facultative parasites are photosynthetic hemiparasites. Parasitic plants in the Orobanchaceae family include all ranges of parasitic plants, from facultative parasites to obligate hemi- and holoparasites. Among them, the obligate hemiparasite, such as Striga, and obligate holoparasites, such as Orobanche and Phelipanche, are noxious weeds that cause significant reductions in crop yields (Mutuku et al. 2021). The development of efficient control methods for these weedy parasitic plants is of urgent issues, but the fundamental knowledge of plant parasitism is still unraveled.
Host infection and nutrient acquisition from hosts by parasitic plants are achieved by a specialized invasive organ named the haustorium. All Orobanchaceae parasitic plants form haustoria on their roots. Haustorium initiation requires external haustorium-inducing factors (HIFs) produced by host plants (Goyet et al. 2019, Furuta et al. 2021). In response to HIFs, obligate hemi- and holoparasites, such as Striga and Phelipanche, terminate radicle elongation and induce radial cell expansion and cell division to form a swollen-tip structure, termed a terminal prehaustorium (Fernández-Aparicio et al. 2016, Cui et al. 2018, Furuta et al. 2021). Facultative hemiparasites that do not rely on a host to complete their life cycle, including Triphysaria versicolor and Phtheirospermum japonicum, maintain root apical meristems even after HIF recognition and induce prehaustoria in the upper root transition zone, resulting in multiple lateral prehaustoria along a root (Yoshida et al. 2016). Prehaustoria further invade host roots and develop xylem bridges between the host and parasite, leading to vascular connections for material transfer via the development of mature haustorium structures (Masumoto et al. 2021).
Several HIFs and their structure–activity specificities have been reported in Orobanchaceae members (Goyet et al. 2019). A quinone, 2,6-dimethoxy-p-benzoquinone (DMBQ), is a well-known HIF that can induce prehaustoria of many Orobanchaceae hemiparasites, including the obligate hemiparasite Striga spp. and facultative hemiparasites Triphysaria and Phtheirospermum. However, quinone HIFs are not highly active HIFs in obligate holoparasitic Phelipanche species (Westwood et al. 2010, Goyet et al. 2017). DMBQ can induce prehaustoria in Phelipanche ramosa but only at a concentration higher than 500 µM (Fernandez-Aparicio et al. 2021). Instead, cytokinin (CK) phytohormones, structurally unrelated to quinones, reportedly induce early haustorial structures in P. ramosa. Furthermore, their Brassica napus host plants exude CK-like allelochemicals with prehaustorium-inducing activity (Goyet et al. 2017). Other studies have reported that CKs induce prehaustorium-like structures in Striga asiatica and T. vesicolor (Keyes et al. 2000, Wrobel and Yoder 2001); however, no additional information has been reported to date. Nevertheless, these observations have raised the possibility that CKs may be common HIFs for both hemiparasites and holoparasites.
Genes mediating quinone-type HIF signals were reported in several Orobanchaceae species. Genes encoding quinone oxidoreductase (QR) and the general transcription factor PIRIN were upregulated after the application of DMBQ or host root exudates to T. versicolor and P. japonicum (Bandaranayake et al. 2010, 2012, Ishida et al. 2017). In T. versicolor, QR1 catalyzes the reduced form of nicotinamide adenine dinucleotide phosphate-dependent quinone reduction, and this process was proposed to be important for transducing quinone signals for prehaustorium induction (Wrobel et al. 2002, Bandaranayake et al. 2010). The knockdown of QR1 or PIRIN resulted in reduced prehaustorium induction in T. versicolor (Bandaranayake et al. 2010, 2012). The auxin biosynthesis gene, YUCCA3, is expressed at the prehaustorium formation site, providing the necessary auxin required for prehaustorium formation in P. japonicum (Ishida et al. 2016). Expansin genes are upregulated by DMBQ treatment in S. asiatica (O’Malley and Lynn 2000). Moreover, the DMBQ-sensing genes were recently identified. DMBQ is recognized via a receptor-like kinase, CANNOT RESPOND TO DMBQ 1 (CARD1) in Arabidopsis thaliana, leading to an increase in intercellular [Ca2+] and the regulation of stomatal closure and immunity (Laohavisit et al. 2020). Phtheirospermum japonicum and S. asiatica have three CARD1 homologs that can complement [Ca2+] elevation defects in Arabidopsis card1, indicating the functional conservation of parasitic plant CARD1 in DMBQ perception (Laohavisit et al. 2020). Inhibitor assays suggest that [Ca2+] regulation is important for DMBQ-induced prehaustorium formation in P. japonicum (Laohavisit et al. 2020). Whether CARD1 homologs are present and functional in obligate holoparasites Phelipanche and Orobanche spp. remains unclarified.
CKs are well-known plant growth regulators. In plant roots, CKs promote vascular differentiation and nodulation, regulate nutrient uptake and inhibit lateral root formation and root elongation (Werner and Schmülling 2009, Kieber and Schaller 2018). CK signals are transduced via a two-component system. In A. thaliana, ARABIDOPSIS HISTIDINE KINASE 2 (AHK2), AHK3 and AHK4 were identified as CK receptors (Inoue et al. 2001, Nishimura et al. 2004). These histidine kinases have cyclase/histidine kinase-associated sensing extracellular domains that bind to CKs (Kakimoto 2003). Binding to CK activates the phosphorylation of histidine in the kinase domain, and the phosphorylation signal is transferred to histidine on ARABIDOPSIS HIS PHOSPHOTRANSFER PROTEINS, which then transfers the phosphoryl group to the receiver domains of ARABIDOPSIS RESPONSE REGULATORS (ARRs) (Ferreira and Kieber 2005, To and Kieber 2008).
Orobanchaceae parasitic plants can respond to various types of molecules as HIFs, yet the signaling pathways for each HIF and their relationships are largely unexplored. Moreover, although DMBQ was isolated from Sorghum extracts (Chang and Lynn 1986), DMBQ is barely detected in A. thaliana root exudates, which have high prehaustorium induction activities (Wang et al. 2020). Therefore, the actual molecules in host root exudates responsible for prehaustorium induction are still unknown. In this study, we show that CKs function in prehaustorium induction in S. hermonthica but not in P. japonicum. Taking advantage of the available inhibitors, we evaluated the relationship of their signaling pathways in prehaustorium formation and demonstrated the presence of potential HIFs in host root exudates.
Results
CKs induce prehaustoria in Striga hermonthica
Previous studies showed that CKs induce the formation of prehaustoria (early haustorial structures) in P. ramosa and prehaustorium-like structures in S. asiatica (Keyes et al. 2000, Goyet et al. 2017), implying that CKs might be common HIFs in Orobanchaceae. Therefore, we tested the prehaustorium-inducing activity of five natural or synthetic CKs, including 6-benzylaminopurine (BA), kinetin (KIN), N6-isopentenyladenine (2iP) and trans-zeatin (tZ), and a synthetic derivative thidiazuron (TDZ), on the obligate hemiparasite S. hermonthica and the facultative hemiparasite P. japonicum. We tested a range of CK concentrations on germinated S. hermonthica seedlings and measured prehaustorium formation (Figs. 1, 2). For comparison, a well-known HIF, DMBQ and host root exudates from rice or Arabidopsis were tested using the same conditions. All tested CKs induced root tip swelling and the proliferation of haustorial hairs on the surface of swelling sites, a typical prehaustorial structure observed after treatment with previously known HIFs or host root exudates (Fig. 1B). Dose–response experiments showed that all tested CKs had higher prehaustorium-inducing activities than DMBQ, of which TDZ had the highest and KIN had the lowest activities (Fig. 2). Prehaustorium formation stimulated by DMBQ reached about 80% at 3 µM (Fig. 2A), whereas similar levels were achieved by nanomolar ranges of CKs (10, 30, 80, 250 and 400 nM by TDZ, tZ, 2iP, BA and KIN, respectively; Fig. 2B).
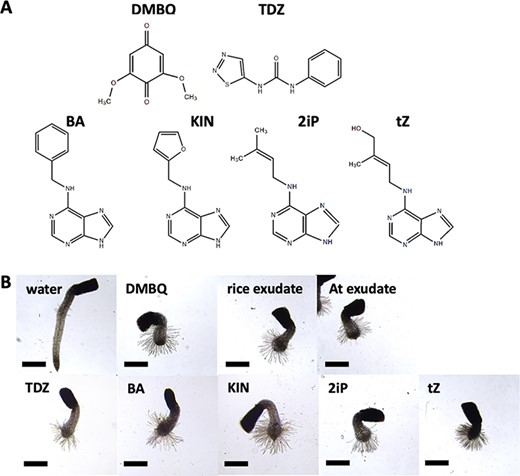
Prehaustorium-inducing activity of several HIFs including quinone-type, phenolic-type and CK-type HIFs. (A) The chemical structures of HIFs tested in this study. (B) Striga hermonthica prehaustoria induced by rice root exudate, A. thaliana (At) root exudate, DMBQ (10 μM), TDZ (200 nM), BA (500 nM), KIN (500 nM), 2iP (200 nM) and tZ (200 nM). Scale bars = 500 μm.
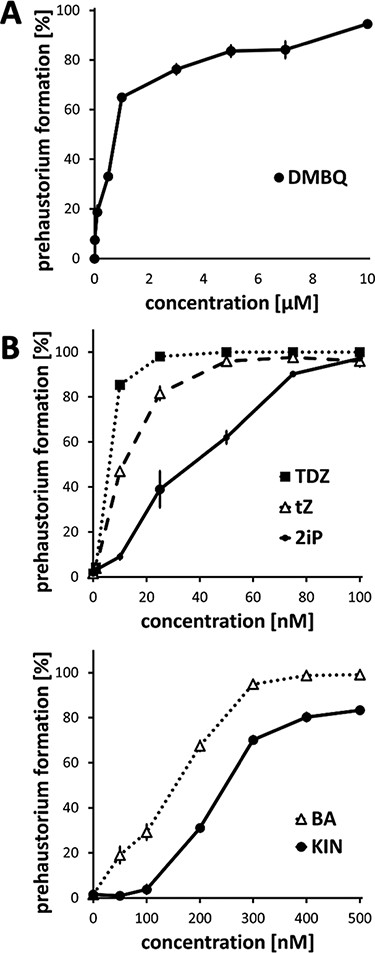
Concentration-dependent prehaustorium-inducing activity of HIFs.( A) Prehaustorium-inducing activity of DMBQ. (B) Prehaustorium-inducing activity of CKs (BA, KIN, tZ, TDZ and 2iP). Striga hermonthica seedlings were treated with HIFs for 24 h. Data represent the mean ± standard error (n = 3).
CKs do not induce prehaustoria in P. japonicum
We next tested the effects of CKs on the facultative parasite P. japonicum, but none of the tested CKs induced prehaustoria after 7 d of treatment at 500 nM (Supplementary Fig. S1A, B). To confirm that P. japonicum can respond to CKs, we observed P. japonicum morphological changes after CK treatments. As was the case for Arabidopsis (Werner et al. 2001, Smets et al. 2005), CKs altered root morphology in P. japonicum (Supplementary Fig. S1). TDZ suppressed lateral root formation by one-half compared to that of the water treatment (Supplementary Fig. S1C), and tZ, TDZ and 2iP significantly inhibited primary root growth (Supplementary Fig. S1D). Moreover, BA or KIN treatments increased root hair proliferation, whereas tZ or TDZ induced root greening (Supplementary Fig. S1B). These observations indicate that P. japonicum can respond to exogenously applied CKs as growth regulators, but not as HIFs.
Dissection of signaling pathways for prehaustorium induction in S. hermonthica
The above-described experiments indicate that S. hermonthica can induce prehaustoria in response to both quinones and CKs. However, how these HIF signals are transduced and interact with each other in inducing prehaustorium are unknown. To understand the relationships of haustorium induction signaling pathways, we investigated the effect of chemical inhibitors on S. hermonthica prehaustorium formation. Tetrafluoro-1,4-benzoquinone (TFBQ) (Fig. 3A), a DMBQ analog, has been used as an inhibitor for DMBQ-induced prehaustorium formation in S. asiatica, T. versicolor and P. japonicum (Smith et al. 1996, Wang et al. 2019, Laohavisit et al. 2020). We found that TFBQ inhibits not only DMBQ-induced prehaustorium formation but also CK-induced prehaustorium formation (Fig. 3C, Supplementary Figs. S2A, S3). Prehaustorium formation was gradually restored by increasing the concentration of DMBQ or tZ, indicating that TFBQ perturbs the activity of these HIFs (Fig. 3C). As CK inhibitors, we tested two CK analogs, LGR-991 (Fig. 3B) and PI-55 (Supplementary Fig. S4A), that block CK binding to AHKs, the CK receptors in Arabidopsis, in a CK-competitive manner (Spíchal et al. 2009, Nisler et al. 2010). Although PI-55 was reported to suppress prehaustorium formation in P. ramosa (Goyet et al. 2017), we found that PI-55 itself induced prehaustoria in S. hermonthica at low concentration (1 µM) and did not reduce the prehaustorium-inducing activity of DMBQ or tZ (Supplementary Fig. S4B), most likely due to their weak CK agonist activity (Spíchal et al. 2009). In contrast, LGR-991 has lower agonistic activity (Nisler et al. 2010), and it does not induce prehaustoria at less than 20 µM concentration (Supplementary Fig. S4C). Thus, we used 10 µM LGR-991 to test the effects of a CK inhibitor on prehaustorium induction. LGR-991 at 10 µM inhibited prehaustorium formation induced by CKs (Fig. 3D), suggesting that CK perception by AHK homologs is necessary for prehaustorium induction by CKs in S. hermonthica. However, LGR-991 did not affect prehaustoria formation induced by DMBQ (Fig. 3D), indicating that CK perception per se is dispensable in DMBQ-induced prehaustorium formation in S. hermonthica.

Inhibitory effects on S. hermonthica prehaustorium induction by DMBQ and tZ. (A, B) The chemical structures of inhibitors: TFBQ, a DMBQ analog; LGR-991, a CK inhibitor. (C, D) The inhibitory effect of 20 µM TFBQ (C) and 10 µM LGR-991 (D) on DMBQ or tZ. Striga hermonthica was treated with HIFs and inhibitors for 24 h. Data represent the mean ± standard error (n = 3).
Gene expression downstream of each HIF
Inhibitor assays indicated that CKs may activate the DMBQ-dependent pathway, because TFBQ suppressed CK-dependent prehaustorium formation. To test this hypothesis, we analyzed the expressions of DMBQ-responsible genes in response to CKs. We used reverse transcription-quantitative polymerase chain reaction (RT-qPCR) to measure the expression of four genes, QR2, PIRIN, YUCCA3 (YUC3) and EXPANSIN B1 (EXPB1), which were previously reported to be DMBQ-inducible genes in Orobanchaceae parasitic plants (O’Malley and Lynn 2000, Bandaranayake et al. 2010, 2012, Ishida et al. 2016, 2017). In addition, we analyzed the expression of chitinases (chitinase and a class iv chitinase), the plant defense-related genes (Sharma et al. 2011) reported to be highly expressed at the early stages during host infection and by DMBQ treatment in S. hermonthica (Yoshida et al. 2019). Both ShQR2 and ShPIRIN were upregulated at early time points (1–6 h) after DMBQ treatments (Fig. 4A). In contrast, tZ treatment induced the expression of ShPIRIN but not ShQR2 (Fig. 4A). ShYUC3 expression increased at 6 h by both HIFs (Fig. 4A). The expression of ShEXPB1 was upregulated by DMBQ at 24 h but not by tZ (Fig. 4A). The chitinase homolog was upregulated by DMBQ at 6 h, whereas it was slightly upregulated by tZ at 6 h (Fig. 4A). The class iv chitinase gene expression was upregulated by DMBQ at 24 h but not by tZ (Fig. 4A). These results indicate that CKs do not activate the entire DMBQ-dependent pathway but partially overlap with the DMBQ pathway.
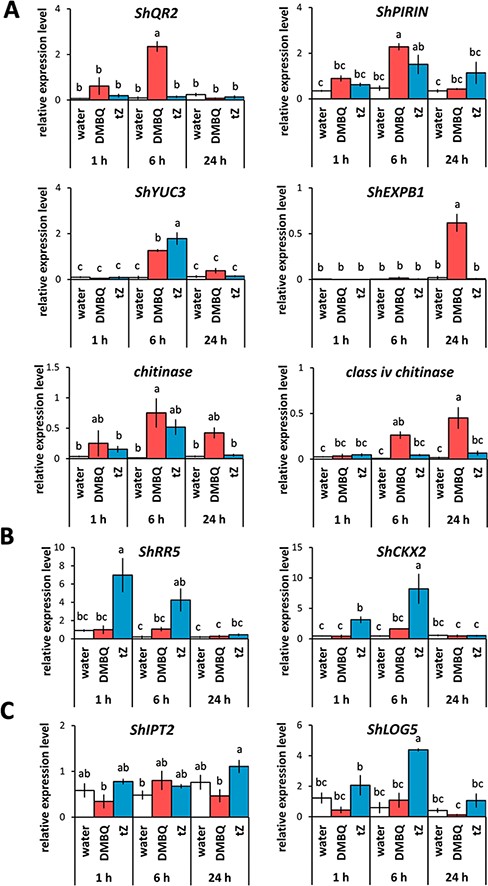
Expression of genes involved in prehaustorium formation, CK signaling and CK biosynthesis in response to several HIFs. (A) Expression of DMBQ marker genes (ShQR2, ShPIRIN, ShYUC3, ShEXPB1, chitinase and class iv chitinase). (B) Expression of genes involved in CK signaling (ShRR5 and ShCKX2). (C) Expression of genes involved in CK biosynthesis (ShLOG5 and ShIPT2). Striga hermonthica was treated with DMBQ (10 µM) or tZ (200 nM) for 24 h. Data represent the mean ± standard error (n = 3). Letters indicate the statistically significant differences (Tukey’s test, P < 0.05).
Next, we tested how DMBQ affects the CK signaling. The expression of S. hermonthica genes homologous to the CK-responsive ARR5 and CYTOKININ OXIDASE/DEHYDROGERASE 2 (CKX2) in A. thaliana (Bhargava et al. 2013) was investigated by RT-qPCR. As expected, the expression of ShRR5 and ShCKX2 was significantly increased at the early time points (1 and 6 h post-treatment) by tZ, but DMBQ did not significantly affect the expression of these genes (Fig. 4B). These findings imply that quinones induce prehaustorium formation without activating the canonical CK signaling pathway. To investigate whether quinones affect CK biosynthesis, we measured the expression of CK biosynthesis genes in S. hermonthica in response to DMBQ (Fig. 4C). Adenosine phosphate-isopentenyltransferases (IPTs) and CK riboside 5ʹ-monophosphate phosphoribohydrolases encoded by LONELY GUY (LOG) are known as key genes in the CK biosynthesis pathway (Kamada-Nobusada and Sakakibara 2009). We searched for homologous genes of IPTs and LOGs in the S. hermonthica transcriptome (Yoshida et al. 2019) and found 9 and 10 homologs, respectively (Supplementary Fig. S5). Because AtLOG5 and AtIPT2 encode functional enzymes responsible for major CK biosynthesis activity in root apical meristems and vascular tissues (Miyawaki et al. 2004, Kuroha et al. 2009), we chose their orthologues, ShLOG5 and ShIPT2, for expression analyses. ShIPT2 expression was slightly induced by tZ at 24 h but not by DMBQ (Fig. 4C). ShLOG5 was highly upregulated by tZ at 6 h by approximately 5-fold compared to the control treatment, but no significant change was observed by DMBQ treatment (Fig. 4C). Therefore, our results do not provide apparent evidence for the activation of CK biosynthesis and signaling upon DMBQ treatment.
HIFs in host root exudates
In natural conditions, S. hermonthica initiates haustorium formation in response to nearby host roots. Therefore, host root exudates must contain HIFs, but the identities of host-derived HIFs are unclear. To answer this question, we took advantage of available inhibitors and investigated the effects of TFBQ and LGR-991 on prehaustorium formation stimulated by host root exudates (Fig. 5A). We choose two plant species, rice and Arabidopsis; rice as a representative of natural hosts for S. hermonthica and Arabidopsis as a plant having prehaustorium-inducing activity for S. hermonthica although it is non-host in natural conditions (Yoshida and Shirasu 2009). Both TFBQ and LGR-991 reduced prehaustorium formation when treated with rice or Arabidopsis root exudates. Whereas rice or Arabidopsis root exudates led to prehaustorium induction levels of more than 80%, the addition of TFBQ reduced prehaustoria by less than 10% in both (Fig. 5A, Supplementary Fig. S2C). The treatment of LGR-991 decreased the prehaustorium formation rates by either exudate to 40–60% (Fig. 5A, Supplementary Fig. S2D). Because LGR-991 showed little inhibition of DMBQ-induced prehaustoria formation in S. hermonthica (Fig. 3D), these results indicate that root exudates of both rice and Arabidopsis not solely contain quinone-type HIFs but may also contain CK-type HIFs.
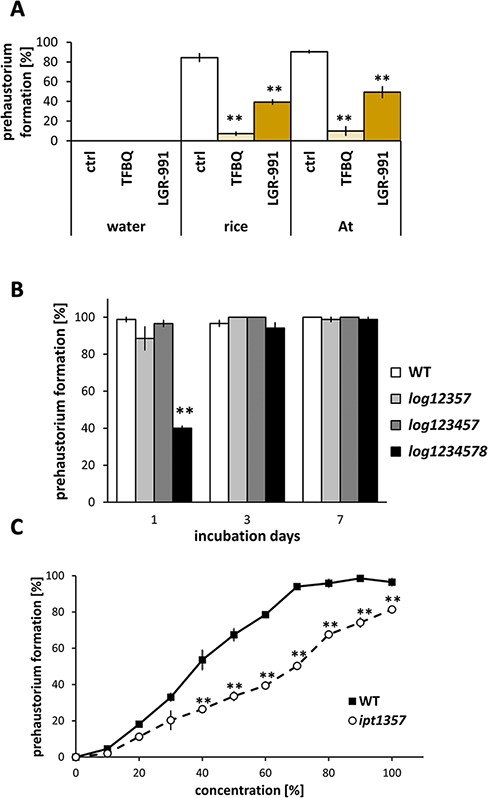
Prehaustorium-inducing activity of host plant exudates on S. hermonthica. (A) The effect of inhibitors on S. hermonthica prehaustorium induction by rice or A. thaliana (At) exudates. TFBQ (20 µM); LGR-991 (10 µM). Plant exudates were diluted to a 40% concentration with water. (B) Prehaustorium-inducing activities of root exudates from Arabidopsis log12357, log123457 and log1234578 mutants on S. hermonthica. Root exudates collected from A. thaliana after 1, 3 or 7 d. (C) Prehaustorium-inducing activities of root exudates from Arabidopsis ipt1357 mutant on S. hermonthica. Root exudates collected from A. thaliana after 7 d were diluted with water to the concentration indicated on the x-axis and tested for prehaustorium-inducing activity. The number of prehaustoria after a 24-h treatment was measured. Data represent the mean ± standard error (n = 3). Asterisks indicate statistically significant differences compared to the control (t-test, **: P < 0.01, *: 0.01 < P < 0.05).
To examine whether host root exudates contain CKs, we analyzed prehaustorium-inducing activities of S. hermonthica in the presence of root exudates from A. thaliana mutants defective in CK biosynthesis (Fig. 5B, C, Supplementary Fig. S6). Arabidopsis thaliana has nine LOG genes (LOG1–LOG9), of which LOG1–LOG5, LOG7 and LOG8 have phosphoribohydrolase activity (Kuroha et al. 2009). Seedlings of the hextuple mutant log123457 were reported to have reduced tZ and iP levels compared with wild type (WT) seedlings (Tokunaga et al. 2012). In our study, we used log12357, log123457, log1234578 and ipt1357 mutants. For the exudates collected after 7 d of incubation, all of the log12357, log 123457 and log1234578 mutants did not show significant differences from WT in inducing prehaustorium formation in S. hermonthica (Fig. 5B, Supplementary Fig. S6). Hence, we compared the prehaustorium-inducing activity of root exudates collected with shorter incubation days. Pentuple log12357 and hextuple log123457 did not show significant differences from WT in inducing prehaustorium formation in S. hermonthica (Fig. 5B). By contrast, the heptuple log1234578 mutant had lower prehaustorium-inducing activity than WT when the exudates were compared at a lower concentration (20–40% dilution) (Fig. 5B). To further confirm the effects of CK biosynthesis on the HIF activity, we investigated the exudates of Arabidopsis ipt mutants. Arabidopsis thaliana has seven IPT genes (AtIPT1 and AtIPT3–AtIPT8), and the seedling of the quadruple mutant ipt1357 contains significantly less CK than WT (Miyawaki et al. 2006). The exudate of the quadruple mutant ipt1357 collected after 7 d of incubation showed reduced prehaustorium-inducing activity compared with WT at various dilution ratios (Fig. 5C). These results suggest that CKs from the host contribute to HIF activity at least partially.
Gene expression induced by host root exudates
Finally, we analyzed the expression of the same genes analyzed in Fig. 4 in response to host root exudates. We reasoned that if host root exudates contain a mixture of HIFs, upregulation of any of these genes would also occur. Indeed, ShQR2, ShPIRIN, ShYUC3, ShEXPB1 and two homologous chitinase genes were markedly upregulated at 1 and 6 h after rice exudate treatment (Fig. 6A). Although the response was not as extreme as that in rice, the treatment of Arabidopsis root exudates also upregulated this set of genes, particularly at 1 h after treatment (Fig. 6A). Importantly, two CK-responsive genes, ShRR5 and ShCKX2, which were upregulated only by CK tZ but not by quinones (Fig. 4B), were also upregulated by the rice and Arabidopsis root exudates after 1 and 6 h (Fig. 6B). This result indicates that host exudates trigger CK responses in S. hermonthica. ShIPT2, a CK biosynthesis gene, was upregulated by rice root exudate at 1 and 6 h. Similarly, ShLOG5 was significantly upregulated at 1 h and to a greater extent at 6 h by rice root exudate. Both ShIPT2 and ShLOG5 showed slightly higher expression levels with the treatment of Arabidopsis root exudates at 1 and 6 h, although without statistical significance. These results suggest that, unlike DMBQ, host root exudates promote CK biosynthesis and signaling (Fig. 6D). In summary, the expression of all prehaustorial marker genes and CK-related genes was induced by host root exudates, suggesting the possibility that host root exudates contain a mixture of HIFs including quinones and CKs.
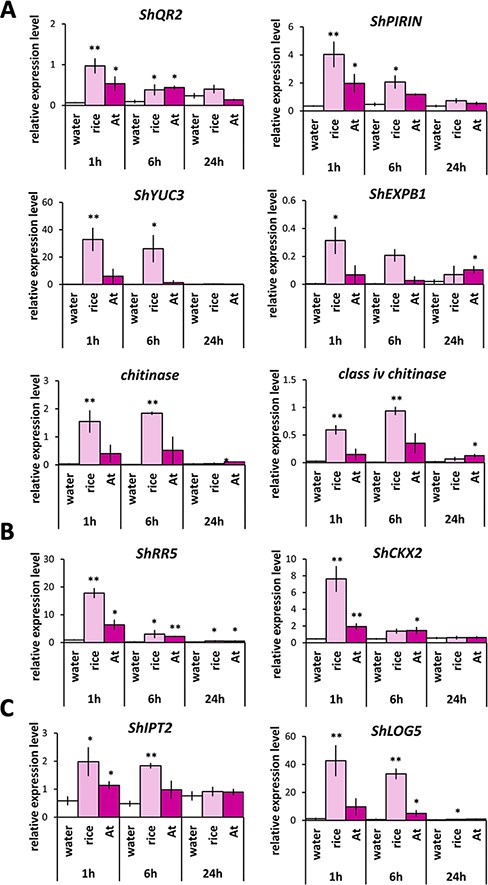
Expression analyses of genes involved in prehaustorium formation, CK signaling and CK biosynthesis in response to treatments with host root exudates. (A) Expression of DMBQ marker genes (ShQR2, ShPIRIN, ShYUC3, ShEXPB1, chitinase and class iv chitinase). (B) Expression of genes involved in CK signaling (ShRR5 and ShCKX2). (C) Expression of genes involved in CK biosynthesis (ShLOG5 and ShIPT2). Striga hermonthica was treated with rice or A. thaliana (At) exudates for 24 h. Data represent the mean ± standard error (n = 3). Asterisks indicate significant differences compared to the control (t-test, **: P < 0.01, *: 0.01 < P < 0.05).
Discussion
CKs function in inducing terminal prehaustoria
CKs were reported to induce prehaustorium formation in P. ramosa (Goyet et al. 2017). This work showed that CKs function as HIFs in S. hermonthica even at a lower concentration than the well-known HIF, DMBQ (Fig. 7A). Although they differed in their photosynthetic ability, e.g. Striga spp. are hemiparasites and P. ramosa is a holoparasite, these plants are both obligate parasitic plants that primarily form terminal haustoria at the radicle tip resulting from the deformation of root apical meristems. We also found that P. japonicum does not form prehaustoria in response to CKs. Phtheirospermum japonicum is a facultative hemiparasitic plant that forms lateral haustoria on the sides of roots without disturbing the root growth. Previously, T. versicolor, another facultative parasite, was reported to form prehaustoria-like structures with CK (BA) treatment (Wrobel and Yoder 2001). However, BA treatment of T. versicolor roots caused root tip swelling and hair proliferation, which resembles the morphology of a terminal prehaustorium. When the roots were transferred to media without BA after 4 h of BA treatment, the morphology of prehaustoria resembled the lateral prehaustoria formed in DMBQ-treated T. versicolor (Wrobel and Yoder 2001). Thus, we infer that CK is an inducer of terminal prehaustoria but not lateral prehaustoria. Accumulative knowledge from previous studies suggested that quinones, phenolic compounds and flavonoids are common HIFs for hemiparasitic Orobanchaceae, including Striga, Phtheirospermum, Triphysaria, Castilleja and Agalinis (Goyet et al. 2019). On the other hand, holoparasitic Phelipanche and Orobanche are not highly responsive to those compounds (Goyet et al. 2017, Fernández-Aparicio et al. 2021). Therefore, it was hypothesized that the species specificity of HIFs could be determined by the photosynthesis ability. Our study adds a new insight for specificities of CK-type HIFs as those could be determined by haustorial morphology that is either terminal or lateral. Because only a few parasite species were analyzed for various HIFs so far, it would be necessary to analyze more parasite species to validate this hypothesis.
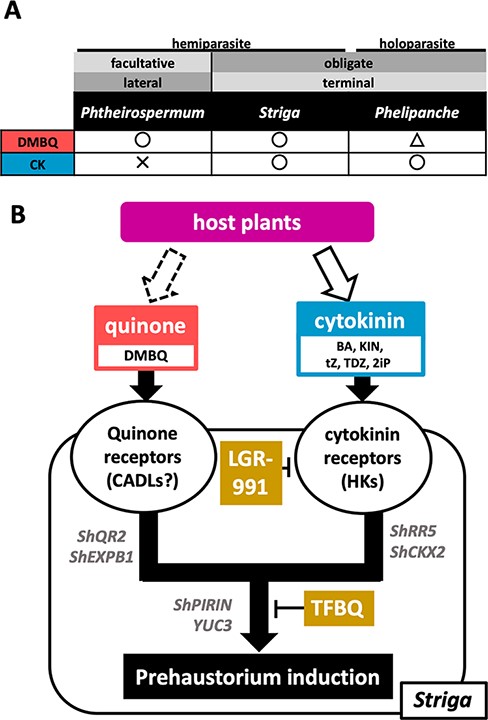
Classification and model of the HIF responses in Orobanchaceae. (A) The classification of Orobanchaceae parasitic plants and the prehaustorium-inducing activities of DMBQ and CK. (B) A model for the signaling pathways of prehaustorium induction in S. hermonthica in response to external quinone and CKs.
Interactions between CK and auxin may explain different HIF activities in S. hermonthica and P. japonicum. During early prehaustorium formation in P. japonicum, auxin biosynthesis mediated by YUC3 at the prehaustorium apex is crucial for prehaustorium development (Ishida et al. 2016). The external application of auxin also increases the number of prehaustoria induced by DMBQ in P. japonicum (Supplementary Fig. S7). CK was shown to suppress asymmetric cell division promoted by auxin and inhibit lateral root initiation (Laplaze et al. 2007). Therefore, exogenous CK may repress auxin signaling and inhibit prehaustorium formation in P. japonicum. In addition, CKs were reported to be negative regulators of primary root growth by modulating root meristems (Werner et al. 2010). In this regard, CKs are proposed to suppress root growth by terminating the root meristematic activity, a role important for forming terminal prehaustoria that require the deformation of a growing root tip.
The relationships of signaling pathways for prehaustorium induction by HIFs
The finding that S. hermonthica prehaustoria can be induced by both DMBQ and CKs enabled us to investigate the relationships between the signaling pathways. TFBQ is a known DMBQ inhibitor of prehaustorium induction in parasitic plants (Smith et al. 1996, Wang et al. 2019) and inhibits DMBQ-induced Ca2+ uptake mediated by CARD1 receptor kinase in Arabidopsis (Laohavisit et al. 2020). However, although TFBQ has been considered as a competitive inhibitor for DMBQ due to its structural similarity, the mode of action of TFBQ has not been clarified. To date, no evidence for the direct binding of DMBQ to CARD1 (and its homologs) is available and neither does TFBQ. In this study, TFBQ also inhibited CK-induced prehaustorium formation. It is unlikely that CKs induce DMBQ production or activate DMBQ signaling, because DMBQ marker genes, including ShQR2 and ShEXPB1, were not upregulated by CK treatments. Our results rather support that TFBQ targets the common downstream signaling pathways of quinone- and CK-type HIFs (Fig. 7B). TFBQ possesses electronegative halogen group(s) that tend to gain an electron and, thus, show high reactivity. Because the redox status is important for prehaustorium induction (Smith et al. 1996, Wada et al. 2019), TFBQ may inhibit prehaustorium formation by disturbing the redox status.
LGR-991, a competitive inhibitor of CK receptors with direct receptor-binding activity (Nisler et al. 2010) effectively inhibited CK-induced prehaustorium formation but not DMBQ-induced prehaustoria in S. hermonthica. Moreover, the CK marker genes, ShRR5 and ShCKX2, were upregulated in tZ-treated S. hermonthica but not in DMBQ-treated seedlings. These results indicate that CK-induced prehaustorium formation is likely mediated through CK receptors and DMBQ-induced prehaustorium formation does not require the activation of CKs.
Taken together, DMBQ and CK signaling pathways for prehaustorium formation are converged at a point upstream of the common pathway. We can distinguish these pathways by the marker gene expression; a subset of genes, such as ShQR2 and ShEXPB1, are activated at the quinone-specific pathway upstream of the converged point, whereas PIRIN, YUC3 and chitinases are acting at the quinone–CK convergent route (Fig. 7).
HIFs in host plant root exudates
DMBQ was first isolated from Sorghum root extract (Chang and Lynn 1986) and is considered a major host-derived HIF. However, the amount of DMBQ in Arabidopsis root exudates is too low to account for its HIF activity (Wang et al. 2020). In this study, we showed that prehaustorium induction by rice and Arabidopsis root exudates was reduced by TFBQ and LGR-991 treatments (Fig. 5A). Because LGR-991 inhibited CK-dependent but not DMBQ-dependent prehaustorium induction, the primary HIFs in host root exudates may be CK-type HIFs. When we used exudates from the Arabidopsis log mutants, prehaustorium induction activity was slightly reduced (Fig. 5B). Moreover, the exudate of the ipt1357 quadruple mutant, another CK-deficient mutant, showed significantly reduced HIF activity compared to wild type, suggesting that CKs contained in Arabidopsis root exudates act as HIFs. On the other hand, these mutant root exudates still retain substantial HIF activity, because the reduction can be observed only in the dilution series of root exudate, indicating that CKs are not the sole component responsible for HIF activity. Consistently, DMBQ marker genes, which are not activated by CKs, are also highly induced by host exudates, indicating that the host root exudates contain quinone-type HIFs and/or other types such as phenolics. Because S. hermonthica treated with host root exudates expressed CK biosynthesis and responsive genes (Fig. 6B, C), endogenous CK production in parasitic plants upon host root exudate treatment may have a function in further promoting prehaustorium formation. In line with this, a recent study indicated that CKs accumulate in rice-extract-treated prehaustoria (Xiao et al. 2022). Arabidopsis root exudates have not been reported to contain CKs, but several reports have shown that CKs are present in rice exudates (Murofushi et al. 1983, Soejima et al. 1992, 1995). Plant root exudates are known to contain various phenolic acids, including ferulic acid and sinapic acid that are potential precursors for quinones (Narasimhan et al. 2003). Therefore, the host root exudates are likely to contain a mixture of quinone- and CK-type HIFs as well as quinone precursors.
In conclusion, we have shown that nanomolar ranges of CKs can induce prehaustoria in Striga but not in P. japonicum, which may influence the host sensitivity and selectivity of the weedy parasitic plants. The presence of multiple HIF pathways may imply the complexity and robustness of prehaustorium induction signaling. This can be taken into account to develop Striga control methods. Determining the exact HIFs involved in host exudates and each signaling component in parasitic plants remains as future research objectives. The combination of genetic and biochemical analysis would dissect the host–parasite signaling interaction.
Materials and Methods
Plant materials
Striga hermonthica seeds were sterilized with 20% (v/v) commercial bleach solution (Kao) five times and then washed with sterile water 10 times. The sterilized seeds were transferred to 9-cm Petri dishes filled with moistened Whatman Glass Microfiber Filters GF/A (GE Healthcare, Little Chalfont, UK) and incubated at 25°C for 4–7 d in dark conditions. To germinate S. hermonthica, seeds were treated with 10 nM strigol (Hirayama and Mori 1999) at 25°C for 24 h in dark conditions.
Phtheirospermum japonicum wild-type seeds (ecotype: Okayama) were sterilized with 10% commercial bleach solution five times and washed with sterile water five times. Sterilized seeds were sown on one-half Murashige and Skoog (MS) (Murashige and Skoog Plant Salt Mixture, Wako Pure Chemical Co., Osaka, Japan) media (pH 5.8) with 0.8% (w/v) agar and 1% (w/v) sucrose and kept at 4°C in the dark for 3 d. The plates were moved to a controlled temperature chamber set at 25°C with a photoperiod of 16 h light/8 h dark and a light intensity of 90 µmol m−2 s−1.
Arabidopsis thaliana ecotype Columbia (Col-0) was used as the wild type. The log12357, log123457, log1234578 and ipt1357 mutants were previously reported (Miyawaki et al. 2006, Kuroha et al. 2009, Tokunaga et al. 2012). Their seeds were sterilized with 5% (v/v) commercial bleach solution and washed with sterile water five times. Sterilized seeds were sown on one-half MS media with 0.8% (w/v) agar and 1% (w/v) sucrose, kept at 4°C in the dark for 3 d and grown at 22°C with a photoperiod of 16 h light/8 h dark and a light intensity of 69 µmol m−2 s−1.
Rice (Oryza sativa cv. Koshihikari) seeds were washed with 70% (v/v) ethanol for 5 min and then sterilized with a 20% (v/v) commercial bleach solution for 30 min with stirring. After sterilization, seeds were rinsed at least five times with sterilized water. For exudate sampling, rice seeds were transferred to wet filter papers (Advantec Co., Tokyo, Japan) in 9-cm Petri dishes and incubated at 25°C for 7–10 d under 16 h light/8 h dark, 90 µmol m−2 s−1, conditions.
Chemicals
DMBQ (Sigma-Aldrich, St. Louis, MO, USA), KIN (Sigma-Aldrich), 2iP, TFBQ (Sigma-Aldrich), PI-55 (Spíchal et al. 2009) and LGR-991 (Nisler et al. 2010) were dissolved in dimethyl sulfoxide (Nacalai Tesque, Kyoto, Japan) as 10 mM stocks. BA (Wako Pure Chemical Co.), tZ (Wako Pure Chemical Co.) and TDZ (Wako Pure Chemical Co.) were dissolved in sterile water as 1 mg/ml stocks. These stocks were diluted with sterile water in each experiment.
Collection of plant root exudates
Five A. thaliana seedlings grown in one-half MS agar plates for 10–14 d were transferred to six-well plates (Iwaki, Shizuoka, Japan) containing 5 ml sterile water/well and incubated at 25°C for 1, 3 or 7 d in the dark. Single 7–10-day-old rice seedlings were transferred into each of the wells and incubated at 25°C for 7 d in the dark. The water in the well was collected and used as the source of plant exudates.
Plant growth experiments and prehaustorium induction assay
Germinated S. hermonthica seedlings were transferred to 96-well plates (Iwaki) containing 100 µl water per well with or without chemicals and incubated at 25°C in the dark. Approximately 20–40 seedlings were placed in each well. After 24 h, the number of prehaustoria was counted using a stereo microscope. Prehaustorium formation was calculated as a percentage of the number of S. hermonthica seedlings forming prehaustoria divided by the number of total S. hermonthica seedlings in each well.
Phtheirospermum japonicum seedlings, grown in one-half MS agar plates with 1% (w/v) sucrose for 7 d, were transferred to 1% (w/v) agar plates containing DMBQ or CKs. Plates were incubated at 25°C with a photoperiod of 16 h light/8 h dark, 90 µmol m−2 s−1. Lateral root number, root length and prehaustorium number were measured after 7 d for the data presented in Supplementary Fig. S1A–C. For Fig. S4B–D, and seedlings of P. japonicum, grown in one-half MS agar plates with 1% (w/v) sucrose for 10 d, were transferred to 1% (w/v) agar plates containing the indicated chemicals and incubated for 7 d with a photoperiod of 16 h/8 h dark, 90 µmol m−2 s−1, before measuring lateral root number, root length and hypocotyl length.
RT-qPCR
RNA was extracted from S. hermonthica treated with water or HIFs for 1, 6 or 24 h using an RNeasy Plant Mini Kit (Qiagen, Hilden, Germany) according to the manufacturer’s instructions. Genomic DNA was removed from samples by RNase-free DNase I (Qiagen) treatment. The total RNA concentration was measured using a NanoDrop ND-1000 (NanoDrop Technologies, Wilmigton, DE, USA). The extracted RNAs (500 ng) were reverse transcribed to complementary DNA (cDNA) using the ReverTra Ace qPCR RT Master Mix (Toyobo, Osaka, Japan) according to the manufacturer’s instructions. cDNA was diluted 10 times, and 2 µl was used as the template in 20 µl of THUNDERBIRD SYBR qPCR Mix (Toyobo) with 0.3 mM of each primer. The quantification of RT-qPCR products was carried out on a CFX-Connect (Bio-Rad, Hercules, CA, USA) according to the manufacturer’s instructions using the standard curve method. Actin was used as an internal control. Target genes in S. hermonthica were searched by blastp using the corresponding A. thaliana genes and the assembled S. hermonthica transcriptome (Yoshida et al. 2019) as the queries. The primer sets used in this study are listed in Supplementary Table S1.
Supplementary Data
Supplementary data are available at PCP online.
Data Availability
The data underlying this article are available in the article and in the Supplementary material.
Funding
Grants-in-Aid for Scientific Research from Ministry of Education, Culture, Sports, Science and Technology (JP19K16169 to S.C., JP19K22432, JP21H02506, JP20H05909 to S.Y.); PRESTO from Japan Science and Technology Agency (JPMJPR194D to S.Y.); Asahi Glass Foundation; NAIST University Fellowships for the Creation of Innovation in Science and Technology to N.A.
Acknowledgements
The authors thank the late Prof. A. G. Babiker (Environment and Natural Resources and Desertification Research Institute, Sudan) and the late Emer. Prof. Kenji Mori (The University of Tokyo) for kindly providing Striga hermonthica seeds and strigol, respectively. The inhibitors PI-55 and LGR-991 were provided by Dr. Lukáš Spíchal (Palacký University, Czech Republic). The log12357, log123457 and log1234578 mutants were gifts from Drs. Takatoshi Kiba and Hitoshi Sakakibara (Nagoya University), and the ipt1357 mutant was provided by Prof. Tatsuo Kakimoto (Osaka University).
Author Contributions
N.A. and S.Y. conceived this study. N.A. and S.C. performed the experiments. N.A., S.C. and S.Y. wrote the manuscript.
Disclosures
The authors have no conflicts of interest to declare.