-
PDF
- Split View
-
Views
-
Cite
Cite
Mitsuhiko Muroya, Haruka Oshima, Shoko Kobayashi, Aya Miura, Yohei Miyamura, Hajime Shiota, Kiyoshi Onai, Masahiro Ishiura, Katsushi Manabe, Shinsuke Kutsuna, Circadian Clock in Arabidopsis thaliana Determines Flower Opening Time Early in the Morning and Dominantly Closes Early in the Afternoon, Plant and Cell Physiology, Volume 62, Issue 5, May 2021, Pages 883–893, https://doi.org/10.1093/pcp/pcab048
- Share Icon Share
Abstract
Many plant species exhibit diurnal flower opening and closing, which is an adaptation influenced by the lifestyle of pollinators and herbivores. However, it remains unclear how these temporal floral movements are modulated. To clarify the role of the circadian clock in flower movement, we examined temporal floral movements in Arabidopsis thaliana. Wild-type (accessions; Col-0, Ler-0 and Ws-4) flowers opened between 0.7 and 1.4 h in a 16-h light period and closed between 7.5 and 8.3 h in a diurnal light period. In the arrhythmic mutants pcl1-1 and prr975, the former flowers closed slowly and imperfectly and the latter ones never closed. Under continuous light conditions, new flowers emerged and opened within a 23–26 h window in the wild-type, but the flowers in pcl1-1 and prr975 developed straight petals, whose curvatures were extremely small. Anti-phasic circadian gene expression of CIRCADIAN CLOCK ASSOCIATED 1 (CCA1), LATE ELONGATED HYPOCOTYLE (LHY) and TIMING OF CAB EXPRESSION 1 (TOC1) occurred in wild-type flowers, but non-rhythmic expression was observed in pcl1-1 and prr975 mutants. Focusing on excised petals, bioluminescence monitoring revealed rhythmic promoter activities of genes expressed (CCA1, LHY and PHYTOCLOCK 1/LUX ARRHYTHMO, PCL1/LUX) in the morning and evening. These results suggest that the clock induces flower opening redundantly with unknown light-sensing pathways. By contrast, flower closing is completely dependent on clock control. These findings will lead to further exploration of the molecular mechanisms and evolutionary diversity of timing in flower opening and closing.
Introduction
Many diurnal biological activities, such as wake–sleep behavior in animals, are sustained with a periodicity of 24 h under the artificial continuous light and constant temperature conditions. Temporal changes in organism/cell behavior that occur at intervals under constant conditions are known as outputs of circadian clock control (Bünning 1973). In plants, the circadian clock influences many physiological and developmental processes, such as photosynthesis, movement and seasonal flower formation (Dodd et al. 2005, Kinmonth-Schultz et al. 2013).
Plants form flowers to attract pollinators to facilitate the generation of progeny. In flowers, pollinators transfer pollen to stigmas, thereby inducing plant fertilization. Since pollinators are only active during certain times of the day–night cycle, floral movement (i.e. flower opening and closing) must synchronize with the diurnal activity of pollinators (Grant 1992, van Doorn and van Meeteren 2003, Hasegawa et al. 2006, Kessler et al. 2008, Kessler et al. 2010). The opening and closing of many plant flowers appear to be gated by the circadian clock (Sweeny 1963, Engelmann et al. 1974, Kaihara and Takimoto 1979, Bai and Kawabata 2015).
Molecular genetics studies in eukaryotes have identified many genes that are regulated by the clock. Furthermore, transcription and translation of the core clock genes themselves are regulated in a circadian manner (Yakir et al. 2007). Thus, the eukaryotic clock is based upon a circadian feedback loop dependent on the transcription and translation of clock components.
Intensive studies of the model species Arabidopsis thaliana (Arabidopsis) established that circadian feedback loops are controlled by different transcription factors expressed in the morning or evening (Nohales and Kay 2016). CIRCADIAN CLOCK ASSOCIATED 1 (CCA1) and LATE ELONGATED HYPOCOTYL (LHY) are expressed in the morning. These transcription factors bind to the promoter of the evening-expressed gene TIMING OF CAB EXPRESSION 1 (TOC1) to decrease transcription during the day (Alabadi et al. 2001). Near dusk, TOC1 transcription is induced by another transcription factor, REVEILLE8 (Hsu et al. 2013). TOC1 binds to CCA1 and LHY to repress transcription (Gendron et al. 2012, Huang et al. 2012). Thus, these transcription factors form the circadian feedback loops. These loops are connected with other loops in which transcription factors and PSEUDO RESPONSE REGULATOR proteins (PRR9, PRR7 and PRR5) increase throughout the day to repress the expression of CCA1 and LHY (Nakamichi et al. 2010). Subsequently, the expression of PRR9 and PRR7 decreases in response to the evening-expressed transcription factor PHYTOCLOCK1 (PCL1, also designated as LUX, Hazen et al. 2005, Onai and Ishiura 2005, Huang and Nusinow 2016). In the morning, the expression of PCL1/LUX is repressed by CCA1 and LHY (Hazen et al. 2005, Helfer et al. 2011, Huang and Nusinow 2016, Kamioka et al. 2016). In addition, PCL1/LUX forms a complex, called the evening complex (EC), with proteins, such as EARLY FLOWERINGs, ELF3 and ELF4, that suppress the expression of target genes, such as PRR7, PRR9 and PHYTOCHROME INTERACTING FACTOR 4, PIF4 (Helfer 2011, Dixon 2011, Nusinow 2011). EC also binds to the genes involved in photosynthesis and growth to regulate transcription (Ezer 2017). The genes of photosynthesis and growth are also directly controlled by these PRR proteins (Nakamichi et al. 2012, Liu et al. 2013, Liu et al. 2016). These circadian feedback loops might function throughout the entire plant such that each organ or tissue could respond to daily environmental changes (Endo et al. 2014, Takahashi et al. 2015). However, our understanding of circadian feedback loops in flower organs is limited to nocturnal volatile emissions from Petunia flowers (Fenske et al. 2015).
Arabidopsis flowers repress the formation of insect attractive traits, namely volatiles, color and size (Chen et al. 2003, Hoffmann et al. 2003, Krizek and Anderson 2013, Boachon et al. 2015). Arabidopsis probably tends to limit insect attraction to the flower as a reproductive adaptation strategy. However, the flowers open and close during the day (Müller 1961). Currently, the underlying control mechanism of movement and significance of insect limitation remains unknown. We hypothesized that circadian feedback loops may underpin the temporal opening and closing of Arabidopsis flowers.
In this study, we demonstrated that circadian feedback loops are essential to daily floral behavior through the analyses of circadian arrhythmic mutants pcl1-1 and prr975. Both mutants open their flowers daily after the dark-to-light transition at a timing similar to the wild-types. The mutant flowers show a deficiency in closing. Thus, pcl1-1 showed a marked delay in flower closing. prr975 exhibited a severe closing defect. Under the continuous light conditions, the wild-types developed flowers that opened and closed at expected circadian timings. By contrast, pcl1-1 developed flowers with a closing shape like cleistogamy. In addition, prr975 showed a severe opening defect. Consistent with the abnormal phenotypes, mutant flowers lost the rhythmic oscillation of core clock genes CCA1, LHY and TOC1 as the circadian feedback loops. This study enabled our knowledge of diurnal flower opening and closing to proceed to molecular analysis between the circadian clock and plant leaf and flower movement.
Results
Flower opening and closing in Arabidopsis accessions
Arabidopsis flowers exhibited opening and closing under the diurnal light conditions at Müller’s B4 and B5 flower developmental stages (Müller 1961, Smyth et al. 1990; Fig. 1A, B, Supplementary Fig. S1). To obtain the diurnal gating index for flower opening and closing, we determined the profile of petal curvatures, which was schematized and is presented in Fig. 1C. To quantify opening and closing times, Topen (time of flower opening) and Tclose (time of flower closing) were defined as the times of flower opening and closing, respectively (Fig. 1C). Thus, the half-maximal value for curvature reflects the flower opening and closing times.
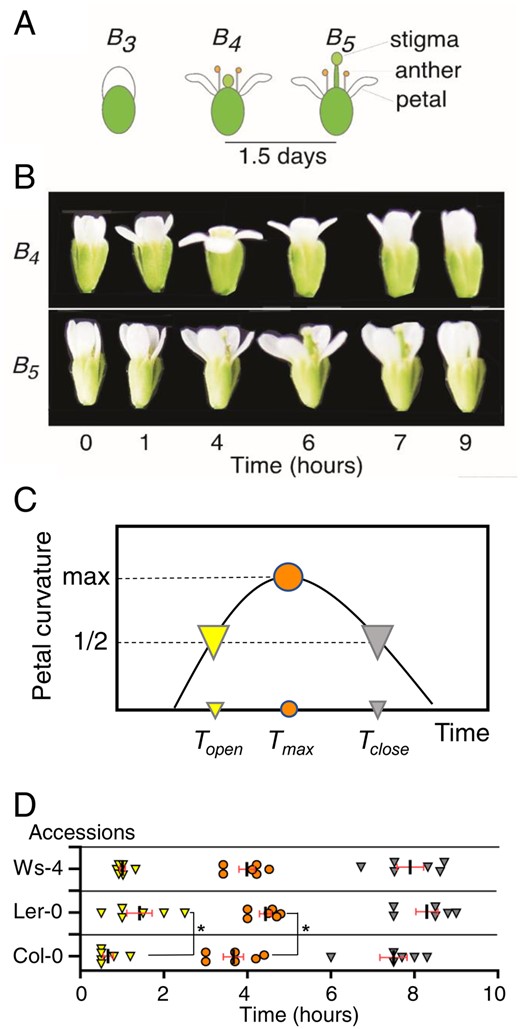
Flower opening and closing in Arabidopsis. (A) Müller’s developmental stages of Arabidopsis flower opening and closing. At stage B3, the petals elongate enough to cover the pistil and stamens. B4 flowers have a shorter pistil than stamens. In stage B5, the pistil extends longer than the stamens. In our experimental conditions, the duration from opening to wilting for the petal was shorter than 1.5 d (Supplementary Fig. S1). (B) The photographs show dynamic movements of stages B4 and B5. The petals curve during opening and closing in the day. The time in the light is shown below the photograph. Flowers of the Col-0 accession are represented. (C) Schematized graph defining the time points in the dynamic petal curvature change. The vertical and horizontal axes represent the curvature of facing petals and times in the light, respectively. Topen and Tclose are defined as indices of opening and closing, which correspond to half the value of the maximal petal curvature. The former and latter are depicted as yellow and gray arrowheads, respectively. In addition, circles filled in orange show the maximal curvature and original time, Tmax. (D) Distributions of Topen, Tclose and Tmax in Arabidopsis accessions. The data were plotted along the hours in the light. In advance of the time-course experiment, 8 h of darkness reset the time of flower opening. The symbols represent the times defined as (A). Each average time is shown as a short bar. An asterisk shows statistical significance (P < 0.05, one-way ANOVA and Tukey’s tests). Data were obtained from three tests. In each test, two plants were examined. In each plant, a bud changed to a flower every day.
To probe the genetic variation in these indices, we examined Arabidopsis accessions, Col-0, Ler-0 and Ws-4. Daily light cycles (16-h light and 8-h dark) were administered to reset the circadian clock in the plants. Then, time-lapse images were captured from budding to floral movement in light conditions as shown in Fig. 1B. We further analyzed the petal curvature. From the obtained profiles, each value of Topen and Tclose was extracted, which corresponded to half-maximal petal curvature (Fig. 1D, Table 1). The data revealed ranges of opening and closing from 0.7–1.4 to 7.5–8.3 h, respectively (Fig. 1D). Statistical analysis indicated a significant difference (P < 0.05) in Topen between Ler-0 and Col-0, suggesting that genetic variation influences opening time. In addition, events of Topen were limited to a short time range (∼1 h), as well as those of Tclose. This precise gating suggested possible control by the circadian clock.
Times in the flowering and maximal curvature of petals of Arabidopsis accessions
Accession . | Topen (h) . | Tmax (h) . | Tclose (h) . | Max. curvature (°) . |
---|---|---|---|---|
Col-0 | 0.7 ± 0.1 | 3.7 ± 0.2 | 7.5 ± 0.3 | 154.2 ± 7.8 |
Ler-0 | 1.4 ± 0.3* | 4.4 ± 0.1* | 8.3 ± 0.3 | 171.7 ± 9.7 |
Ws-4 | 1.0 ± 0.1 | 4.0 ± 0.2 | 7.9 ± 0.3 | 132.0 ± 13.3 |
Accession . | Topen (h) . | Tmax (h) . | Tclose (h) . | Max. curvature (°) . |
---|---|---|---|---|
Col-0 | 0.7 ± 0.1 | 3.7 ± 0.2 | 7.5 ± 0.3 | 154.2 ± 7.8 |
Ler-0 | 1.4 ± 0.3* | 4.4 ± 0.1* | 8.3 ± 0.3 | 171.7 ± 9.7 |
Ws-4 | 1.0 ± 0.1 | 4.0 ± 0.2 | 7.9 ± 0.3 | 132.0 ± 13.3 |
Topen and Tclose represent the times of flower opening and closing, respectively, and corresponding to times of half-maximal value for petal curvature, i.e. max. curvature. Tmax means the time of maximal petal curvature. The timing of h = 0 corresponds to the transition from dark-to-light conditions.
P < 0.05 (mean±SEM; one-way ANOVA and Tukey’s test; n =6) between Col-0 and Ler-0.
Times in the flowering and maximal curvature of petals of Arabidopsis accessions
Accession . | Topen (h) . | Tmax (h) . | Tclose (h) . | Max. curvature (°) . |
---|---|---|---|---|
Col-0 | 0.7 ± 0.1 | 3.7 ± 0.2 | 7.5 ± 0.3 | 154.2 ± 7.8 |
Ler-0 | 1.4 ± 0.3* | 4.4 ± 0.1* | 8.3 ± 0.3 | 171.7 ± 9.7 |
Ws-4 | 1.0 ± 0.1 | 4.0 ± 0.2 | 7.9 ± 0.3 | 132.0 ± 13.3 |
Accession . | Topen (h) . | Tmax (h) . | Tclose (h) . | Max. curvature (°) . |
---|---|---|---|---|
Col-0 | 0.7 ± 0.1 | 3.7 ± 0.2 | 7.5 ± 0.3 | 154.2 ± 7.8 |
Ler-0 | 1.4 ± 0.3* | 4.4 ± 0.1* | 8.3 ± 0.3 | 171.7 ± 9.7 |
Ws-4 | 1.0 ± 0.1 | 4.0 ± 0.2 | 7.9 ± 0.3 | 132.0 ± 13.3 |
Topen and Tclose represent the times of flower opening and closing, respectively, and corresponding to times of half-maximal value for petal curvature, i.e. max. curvature. Tmax means the time of maximal petal curvature. The timing of h = 0 corresponds to the transition from dark-to-light conditions.
P < 0.05 (mean±SEM; one-way ANOVA and Tukey’s test; n =6) between Col-0 and Ler-0.
Flower opening and closing in continuous light conditions with constant temperature
In individual buds, the transition from bud to flower opening (and closing) occurs only once, as a matter of course. The timing indices Topen and Tclose are data based on such transition events. Herein, we applied the indices to probe the influence of the circadian clock on the developmental event of buds in individual plants under the continuous light conditions at a constant temperature. Before monitoring, plants were cultivated under daily light cycles (16 h:8 h) to entrain the clock. From the final dark-to-light transition, the plants were subjected to continuous light conditions, and the buds were monitored for 64 h (Supplementary Fig. S2). As a result, in the wild-type (Col-4), individual buds developed to flowers and opened and closed. Each bud grew enough to expand the petals and open and close in continuous light conditions. The data points shown as Topen were distributed with the circadian period in these conditions (period length estimated by the hours between the 2nd and 3rd hours of Topen: 24.5 ± 1.2 h, n = 6, three tests; Supplementary Fig. S3), suggesting that the circadian clock might influence the timing of the developmental event. We further examined the influence by using cry1 cry2 double mutants, whose seedlings showed a slightly long period phenotype in the circadian rhythm of gene expression or leaf movement (Devlin and Kay 2000, Yanovsky et al. 2001, Gould et al. 2013). In contrast to the long period in the mutant seedlings, the rhythm of the distribution of Topen showed a short period length (22.0 ± 1.6 h), although no significant difference was indicated. However, there was a significant abnormality in the double mutant, with 2.2 h delays (Student’s t-test, P < 0.05, n = 6) compared to Col-4 in response to the dark-to-light transition (Supplementary Fig. S3; Col-4, 0.7 ± 0.2 h; cry1 cry2, 2.8 ± 0.9 h).
Flower opening and closing in circadian arrhythmic mutants
Although mutation in the core clock gene causes an abnormal period length in circadian leaf movement and gene expression (Farré et al. 2005, Nakamichi et al. 2005), the arrhythmic phenotype was the most severe. Circadian clock mutants, pcl1-1 and prr975, showed the arrhythmic phenotype in gene expression and leaf movement as seedlings under the continuous conditions of light (or darkness) with constant temperature (Onai et al. 2004, Nakamichi et al. 2005, Onai and Ishiura 2005). To address the influence of the clock on floral movement, we analyzed Topen and Tclose in pcl1-1 and prr975 mutants. The results obtained in the light period for pcl1-1 showed a delay in opening (0.5 h) that was statistically significant (P < 0.05, Table 2, Fig. 2B; 4 h). In pcl1-1, Tclose was 5 h later than that in the wild type G38. In prr975, the flower exhibited a nonclosing phenotype (Table 2, Fig. 2; 16 h). During the closing phase, petal curvature in the mutants was consistently larger than that of the wild type (Fig. 2). Therefore, the circadian clock had a minor influence on flower opening. Significantly, the clock functioned indispensably to close the flower. Overall, these results suggested that the circadian clock was essential for flower closing, yet dispensable for flower opening.
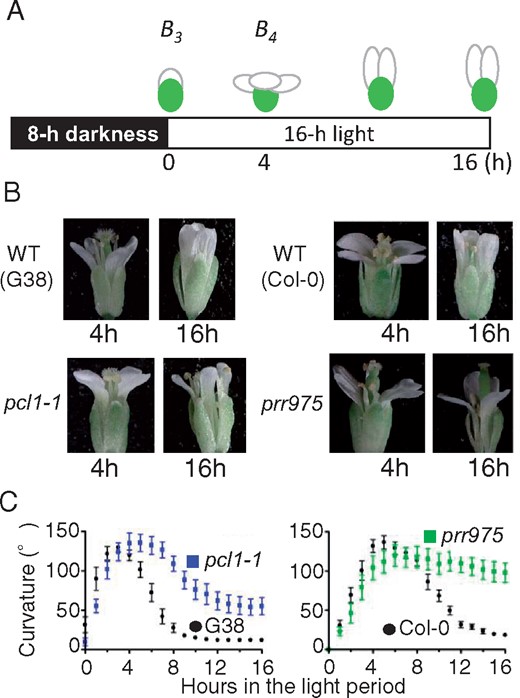
Abnormal flower opening and closing in circadian mutants pcl1-1 and prr975. (A) Scheme of experimental light conditions and flower stages. Numbers below the schematic in the 16-h light condition correspond to the hours that are labeled in the photos. (B) Flower shapes and movements in the wild-type and pcl1-1. Photos on the right represent the shapes in wild-type and prr975 flowers. Both mutants had opened flowers with normal petal curvature (4 h) but did not close for 16 h. The photos represent differences of petal curvature in closing between each wild-type and mutant. The photos were taken by using a stereo microscope. (C) Profiles of petal curvature in the wild-types and mutants. Mean with SE (n = 10).
The opening and closing times and maximal petal curvature of circadian mutants in Col-0 under diurnal light cycles
Plant . | Topen (h) . | Tclose (h) . | Max. curvature (°) . |
---|---|---|---|
G38 | 0.8 ± 0.2 | 6.1 ± 0.3 | 135.0 ± 5.9 |
pcl1-1 | 1.4 ± 0.3* | 11.0 ± 0.7** | 142.1 ± 9.5 |
Col-0 | 2.2 ± 0.3 | 9.9 ± 0.3 | 143.4 ± 7.7 |
prr975 | 3.2 ± 0.5 | N.C. | 128.4 ± 11.0 |
Plant . | Topen (h) . | Tclose (h) . | Max. curvature (°) . |
---|---|---|---|
G38 | 0.8 ± 0.2 | 6.1 ± 0.3 | 135.0 ± 5.9 |
pcl1-1 | 1.4 ± 0.3* | 11.0 ± 0.7** | 142.1 ± 9.5 |
Col-0 | 2.2 ± 0.3 | 9.9 ± 0.3 | 143.4 ± 7.7 |
prr975 | 3.2 ± 0.5 | N.C. | 128.4 ± 11.0 |
Mean±SEM; Student’s t-test (n =10). Asterisks, and
mean P < 0.05 and 0.01, respectively, against each wild-type. N.C., “not closed”. Topen, Tclose and max. curvature are represented as above. The timing of h = 0 corresponds to the transition from dark-to-light conditions.
The opening and closing times and maximal petal curvature of circadian mutants in Col-0 under diurnal light cycles
Plant . | Topen (h) . | Tclose (h) . | Max. curvature (°) . |
---|---|---|---|
G38 | 0.8 ± 0.2 | 6.1 ± 0.3 | 135.0 ± 5.9 |
pcl1-1 | 1.4 ± 0.3* | 11.0 ± 0.7** | 142.1 ± 9.5 |
Col-0 | 2.2 ± 0.3 | 9.9 ± 0.3 | 143.4 ± 7.7 |
prr975 | 3.2 ± 0.5 | N.C. | 128.4 ± 11.0 |
Plant . | Topen (h) . | Tclose (h) . | Max. curvature (°) . |
---|---|---|---|
G38 | 0.8 ± 0.2 | 6.1 ± 0.3 | 135.0 ± 5.9 |
pcl1-1 | 1.4 ± 0.3* | 11.0 ± 0.7** | 142.1 ± 9.5 |
Col-0 | 2.2 ± 0.3 | 9.9 ± 0.3 | 143.4 ± 7.7 |
prr975 | 3.2 ± 0.5 | N.C. | 128.4 ± 11.0 |
Mean±SEM; Student’s t-test (n =10). Asterisks, and
mean P < 0.05 and 0.01, respectively, against each wild-type. N.C., “not closed”. Topen, Tclose and max. curvature are represented as above. The timing of h = 0 corresponds to the transition from dark-to-light conditions.
Flower opening and closing in continuous light conditions
To further explore the function of the clock in flower opening, the mutants’ buds at the stage B3 during 0–16 h in continuous light conditions were further followed by the capturing (Fig. 3A, upper bud/flower). Although the subjective light period lacked an environmental transition from dark to light, wild types G38 and Col-0 opened as expected, following a pattern of circadian timing based on a 24-hour clock as shown in Supplementary Fig. S2. In contrast to the wild type, mutant flowers opened slightly (24 h; Fig. 3B, C, Table 3). Compared to the maximal curvature in the wild types, the levels in the mutants were 8.3% and 30% in pcl1-1 and prr975, respectively (Fig. 3E). These severe opening defects suggested that the clock functions not only to close the flower but also to open it. Because of the severe abnormality in mutants’ opening, it is difficult to estimate the abnormal levels of in circadian dysfunction by using the index of Topen, such as the above tests done in diurnal conditions or wild type in continuous light conditions (Figs. 1, 2 or Supplementary Figs. S1–S3). Accordingly, we defined another index, Tinit (times of initiation in flower opening), for further analysis of the weak opening. This time corresponds to 1 h before the time when the petal curvature profile reached >0° (Fig. 3A, B). Interestingly, prr975, whose closing dysfunction was more severe than pcl1-1, initiated the weak opening at the same circadian times in the wild type. But pcl1-1 initiated the opening at hours in wider duration with a delay of approximately 5 h than the wild type (Fig. 3F).
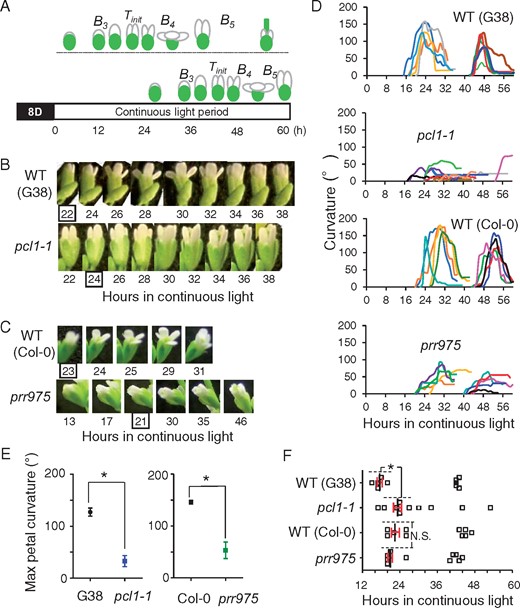
Circadian flower opening properties in continuous light conditions. (A) Experimentally extended light period and flower shapes. Upper: opening and closing occurred in 16–36 h. Subsequently, the pistil enlarged further, but petals lost the dynamic movement activity. Lower: until 36 h, bud kept the shape at stage B3 and the opening and closing occurred in 42–54 h. Photos were captured starting at 0 h (Supplementary Fig. S2). However, the data of buds whose opening occurred in response to the darkness/light transition (i.e. data of 0–12 h) were excluded to distinguish the circadian regulations. Tinit was defined as the index of initiation in flower opening under the continuous light. This means 1 h before time when the petal curvature profile reached >0°. (B and C) Photos of bud/flower in the mutants of pcl1-1 and prr975 in continuous light conditions. Wild-type buds opened and closed the flower (upper photos from 22 to 38 h or 23 to 31 h in (B) and (C), respectively). The mutants pcl1-1 and prr975 exhibited weak openings. Enclosed numbers represent Tinit. (D) Profiles of flower opening in individual bud/flower in continuous light period. Each profile in the curvature is depicted in different color to distinguish each one as individual bud data. Total 10 profiles from bud/flowers are presented in a graph as a data obtained from five plants of each mutant (or wild-type). (E) Maximal petal curvatures in wild-types and the mutants. Data are mean with SD (Student’s t-test; n = 10). (F) Times of initiation in flower opening under continuous light conditions. Data of Tinit were extracted from the profiles in (D). Thus, 10 buds in each plant line were applied. Dashed lines show the statistically analyzed data points (Student’s t-tests, n = 5). Asterisk indicates significant difference, P < 0.05.
Flower opening times and maximal petal curvature in continuous light conditions
Plant line . | Topen (h) . | Max. curvature (°) . | . |
---|---|---|---|
G38 | 23.0 ± 0.5 | 133.6 ± 9.8 | (n = 5) |
pcl1-1 | N.O. | 26.8 ± 9.0** | (n = 8) |
Col-0 | 25.9 ± 1.2 | 166.6 ± 7.0 | (n = 5) |
prr975 | N.O. | 55.7 ± 13.1** | (n = 6) |
Plant line . | Topen (h) . | Max. curvature (°) . | . |
---|---|---|---|
G38 | 23.0 ± 0.5 | 133.6 ± 9.8 | (n = 5) |
pcl1-1 | N.O. | 26.8 ± 9.0** | (n = 8) |
Col-0 | 25.9 ± 1.2 | 166.6 ± 7.0 | (n = 5) |
prr975 | N.O. | 55.7 ± 13.1** | (n = 6) |
Topen and max. curvature in continuous light conditions are represented as above (mean ± SEM; Student’s t-test against corresponding wild-type). N.O. means “not opened”. The timing of h = 0 corresponds to the transition from dark-to-light conditions.
P < 0.01.
Flower opening times and maximal petal curvature in continuous light conditions
Plant line . | Topen (h) . | Max. curvature (°) . | . |
---|---|---|---|
G38 | 23.0 ± 0.5 | 133.6 ± 9.8 | (n = 5) |
pcl1-1 | N.O. | 26.8 ± 9.0** | (n = 8) |
Col-0 | 25.9 ± 1.2 | 166.6 ± 7.0 | (n = 5) |
prr975 | N.O. | 55.7 ± 13.1** | (n = 6) |
Plant line . | Topen (h) . | Max. curvature (°) . | . |
---|---|---|---|
G38 | 23.0 ± 0.5 | 133.6 ± 9.8 | (n = 5) |
pcl1-1 | N.O. | 26.8 ± 9.0** | (n = 8) |
Col-0 | 25.9 ± 1.2 | 166.6 ± 7.0 | (n = 5) |
prr975 | N.O. | 55.7 ± 13.1** | (n = 6) |
Topen and max. curvature in continuous light conditions are represented as above (mean ± SEM; Student’s t-test against corresponding wild-type). N.O. means “not opened”. The timing of h = 0 corresponds to the transition from dark-to-light conditions.
P < 0.01.
Gene expression profiles of the core clock genes in flowers
To confirm the relationship between circadian clock and flower opening and closing, we examined when core circadian genes were expressed in flowers. We collected the buds and flowers under light conditions and analyzed messenger RNA abundance by quantitative reverse transcription PCR (RT-qPCR) methods with specific primers for CCA1, LHY and TOC1. As a result, CCA1 exhibited rhythmicity with expression peaking in the subjective morning (Fig. 4, upper). Homolog LHY showed a similar pattern (Fig. 4, middle). Conversely, TOC1 exhibited subjective evening expression (Fig. 4, lower). Mutants pcl1-1 and prr975 lost the rhythms of expression (Fig. 4, left and right, respectively), indicating dysfunction of the circadian clock in these flowers. Thus, these results were consistent with the above floral phenotypes of the wild-type and mutant lines.
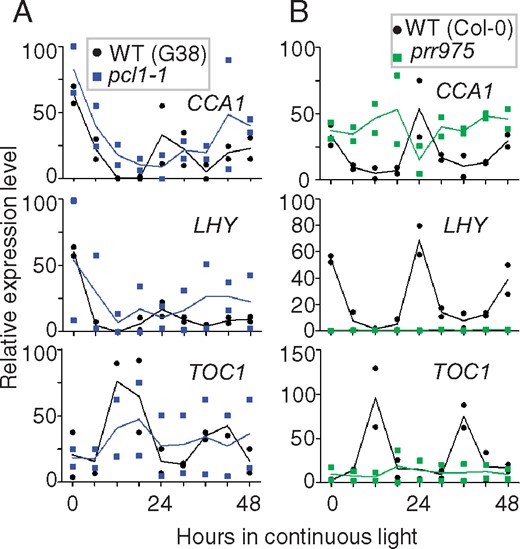
Relative expression profiles in circadian clock genes in flowers. The buds and flowers in continuous light were used for RT-qPCR analysis using specific primer pairs for CCA1 (upper), LHY (middle) and TOC1 (lower). (A) Expression of CCA1, LHY and TOC1 mRNA in the wild-types and pcl1-1. The filled circles and squares colored in black and cyan represent data of the wild-type and pcl1-1, respectively. (B) Expression in the wild-type and prr975. Squares filled in green are prr975. Two biological replicates were examined. Means and individual values are indicated by solid lines and symbols, respectively.
Bioluminescence monitoring of core circadian gene expression in petals
We further tested the robustness and periodicity of circadian gene expression by a bioluminescence monitoring method. Recombinant plants carrying the luciferase gene fused to the promoter of CCA1, LHY or PCL1 were cultivated. To monitor the bioluminescence of petals, we excised them from the bud at Müller’s B3 flower stage, meaning the bud never opened. The petals were inserted onto the surface of agar medium containing luciferin as a substrate. The bioluminescence recording was carried out under continuous light conditions (Fig. 5A). As a result, the petals showed bioluminescence rhythms with a 24-h period under the continuous light (Fig. 5B, Table 4). The circadian phase of maximal CCA1 and LHY expression was at the subjective morning. In addition, PCL1 reporter petals showed a phase delay until the evening (Fig. 5B, Table 4). The index values of robustness FFT-NLLS RAE (Fast Fourier Transformation Non-Linear Least Squares Relative Amplitude Error) were 0.2 (Table 4). Thus, the petals of CCA1, LHY and PCL1 showed robust circadian rhythms of morning and evening expression, respectively, similar to that reported previously for seedling bioluminescence rhythms (Hazen et al. 2005). These data indicate the existence of a robust circadian clock in petal cells.

Circadian rhythms in excised petals. (A) Schematized chart of bioluminescence monitoring. Plants carrying luciferase reporter genes (LUC) were entrained to light and dark cycles (16 h:8 h). Two excised petals were obtained from buds of stage B3. The petals stood on the slant medium in a plastic tube by inserting each base, and the tube was laid on its side in a bioluminescence measurement apparatus. After the end of an 8-h dark period, the monitoring apparatus recorded circadian changes in bioluminescence under continuous light conditions. (B) Bioluminescence rhythms corresponding to the core clock genes. The detached petals from CCA1p:LUC and PCL1p:LUC reporter plants exhibited the robust circadian rhythms in bioluminescence, which are depicted by solid and dashed lines, respectively (upper). Lower panel presents the rhythms of LHYp:LUC reporter petals with that of the PCL1p:LUC reporter as shown above. These raw data points from each representative sample were traced by lines as circadian rhythms in bioluminescence. The measuring interval was 40 min. Two petals in a tube were applied to obtain each rhythm. The bioluminescence level represents the promoter activity for genes CCA1, LHY and PCL1 from two petals on a slant (A.U., arbitrary unit). Two transgenic plant lines were established from genetically independent T1 clones for each of the clock genes. T4 generation plants were used here. Data from line #3 of the CCA1 reporter are presented. The bioluminescence levels in seedlings and petals were higher (2-fold) than line #14. However, the circadian period, phase and relative amplitude of error (RAE) were the same as line #3.
Promoter . | Phase (CT) . | Period (h) . | RAE . | . |
---|---|---|---|---|
CCA1 | 5.1 ± 1.8 | 25.8 ± 0.6 | 0.2 ± 0.0 | (n = 6) |
LHY | 4.6 ± 1.8 | 25.0 ± 2.2 | 0.4 ± 0.2 | (n = 3) |
PCL1 | 15.5 ± 0.6 | 26.8 ±0.5 | 0.2 ± 0.1 | (n = 5) |
Promoter . | Phase (CT) . | Period (h) . | RAE . | . |
---|---|---|---|---|
CCA1 | 5.1 ± 1.8 | 25.8 ± 0.6 | 0.2 ± 0.0 | (n = 6) |
LHY | 4.6 ± 1.8 | 25.0 ± 2.2 | 0.4 ± 0.2 | (n = 3) |
PCL1 | 15.5 ± 0.6 | 26.8 ±0.5 | 0.2 ± 0.1 | (n = 5) |
Phase and period, peak time and period of the rhythms. CT, circadian time. RAE (relative amplitude of error) means relative value of rhythmic strength in circadian condition (mean ± SEM; Student’s t-test between all combinations of the three reporters detected statistical significances of P < 0.05).
Promoter . | Phase (CT) . | Period (h) . | RAE . | . |
---|---|---|---|---|
CCA1 | 5.1 ± 1.8 | 25.8 ± 0.6 | 0.2 ± 0.0 | (n = 6) |
LHY | 4.6 ± 1.8 | 25.0 ± 2.2 | 0.4 ± 0.2 | (n = 3) |
PCL1 | 15.5 ± 0.6 | 26.8 ±0.5 | 0.2 ± 0.1 | (n = 5) |
Promoter . | Phase (CT) . | Period (h) . | RAE . | . |
---|---|---|---|---|
CCA1 | 5.1 ± 1.8 | 25.8 ± 0.6 | 0.2 ± 0.0 | (n = 6) |
LHY | 4.6 ± 1.8 | 25.0 ± 2.2 | 0.4 ± 0.2 | (n = 3) |
PCL1 | 15.5 ± 0.6 | 26.8 ±0.5 | 0.2 ± 0.1 | (n = 5) |
Phase and period, peak time and period of the rhythms. CT, circadian time. RAE (relative amplitude of error) means relative value of rhythmic strength in circadian condition (mean ± SEM; Student’s t-test between all combinations of the three reporters detected statistical significances of P < 0.05).
Discussion
In this study, we developed a method to determine the times of flower opening and closing. By this method, it was demonstrated that Arabidopsis flowers open early in the morning (Topen = 0–2 h) and close in the afternoon (Tclose = 6–9 h), as shown in Fig. 1D. We further revealed that circadian transcription–translation feedback loops influence flower opening and closing. These results improve our understanding of the molecular mechanisms controlling diurnal flower opening and closing.
We compared three Arabidopsis accessions and found a significant difference in Topen between Col-0 and Ler-0 (Fig. 1D, Table 1), meaning that Ler-0 exhibited Topen in a more dispersed and delayed range compared to Col-0. The day length in habitat was different between Ler-0 and Col-0 (i.e. 17 and 15 h, respectively; Michael et al. 2003). This might be considered a factor causing the significant difference of Topen between the accessions. However, another accession Ws-4, whose habitat had a 17-h day length, exhibited no statistical significance. Therefore, day length might be a minor factor in this experiment. Notably, Ler-0 exhibited abnormal cell–cell regulation due to mutations in the leucine-rich repeat receptor-like Ser/Thr kinase gene ERECTA. This abnormality altered the spatiotemporal expression pattern of the gene ASYMMETRIC LEAVES1 (AS1), associating with adaxial–abaxial characteristics of the leaf and cell division (van Zanten et al. 2009). Hence, significant differences in Topen and Tclose suggest the mutation in ERECTA as a major factor in this experiment. The study using leaf movement rhythm shows the variation in circadian period and the phase among accessions (Swarup et al. 1999, Michael et al. 2003). In Supplementary Fig. S3, we showed circadian gating of Topen. Together with the leaf movement study, we expect the discovery of common factors and organ-specific factors in the circadian clock of cotyledons and flowers.
Further study with a greater number of accessions from each ecotype could give insight into the significance of diurnal flower opening and closing in respect to the adaptation to natural habitats. Several plant species respond to dark-to-light transitions by promptly opening their flowers (15 min in Eustoma, Bai and Kawabata 2015; 60 min in Portulaca; Ichimura and Suto 1998). This rapid opening response might be caused by light signal transduction (Bai and Kawabata 2015). Accordingly, the opening delay of Ler-0 flowers might be due to genetic differences in light signaling rather than differences in circadian signaling. The nearly wild-type flower opening of circadian mutants supports this idea (Fig. 2). For instance, many activities in Arabidopsis respond to environmental light ranging from ultraviolet to far red. Corresponding photoreceptor proteins absorb the light (de Wit et al. 2016). To get insight on the light response, we further examined flower opening and closing in the blue light receptor mutant cry1 cry2 (Mockler et al. 1999) under the continuous light and constant temperature conditions. The mutant exhibited a 2-h delay in Topen in response to the dark-to-light transition (Supplementary Fig. S3), supporting the role of light signaling in opening and closing. Although no significant difference in the circadian period length was indicated between Col-4 and cry1 cry2, it seems to be due to a wider range in the distribution of the data in the double mutant. Together with the significant delay in Topen in response to the dark-to-light transition, we suggest that cryptochromes may transmit environmental light signals to the circadian clock in the flower or the clock independent flower opening presented in the arrhythmic mutants (Fig. 2).
Petals develop from developmental disks similar to leaves such that both show the same structural properties, i.e. adaxial/abaxial asymmetry (Friedman et al. 2004). This similarity might give us further insight into molecular mechanisms of flower opening and closing since the cotyledon also shows circadian directional changes adaxially/abaxially from the stem (Onai et al. 2004, Dornbusch et al. 2014). In these reports, leaf elevation angle profiles show several similarities to that of our floral curvature: (i) switching from abaxial to adaxial early in the morning; (ii) phase of the switching (2–3 h in the light); and (iii) movement ends within 16 h of light. In addition, a mutant of light signaling for the morphology and clock, pif4-pif5, exhibited reduced amplitude of the elevation angle. A circadian arrhythmic mutant, elf3-1, exhibited an advanced phase of switching. Accordingly, we expected to get insights into the mechanism of flower opening and closing by analyzing floral movement in mutants pif4-pif5 and elf3-1.
The dysfunction of flower opening and closing was determined in circadian clock mutants pcl1-1 and prr975. Even though pcl1-1 is a single gene deficiency, it was confirmed that the mutation results in dysfunctions not only of the leaves but also of the flowers (Figs. 2, 3). Hence, the PCL1/LUX gene was suggested to play an essential role ubiquitously in the circadian clock. In previous reports using an allele of pcl1-1, lux-2, the same type of arrhythmic gene expression is demonstrated by using bioluminescence reporter technique. Interestingly, the report further indicated that the arrhythmic phenotype was cured under low the temperature continuous light conditions (Jones et al. 2019). So, we are interested in whether such a condition-dependent effect occurs in the movements of the arrhythmic mutant. As a homologous protein of PCL1/LUX, NOX (Latin word for “night”), whose function is unknown, has been reported, by which in vitro binding was demonstrated between the purified protein and target DNA (Helfer et al. 2011). We are interested in further experiments of flower opening and closing using mutants under the low temperature conditions. In addition to the remark dysfunction of opening and closing, it is noteworthy that prr975 buds started flower opening as same circadian timing as analyzed by using the index Tinit (Fig. 3F). This suggests that prr975 buds have the clock with same circadian period to the wild-type despite the low-amplitude abnormality. Thus, without the PRR transcription factors, the flower might harbor a low-amplitude-type circadian clock.
For flowers and excised petals, CCA1 and LHY were expressed in the morning. By contrast, TOC1 and PCL1 were expressed in the evening (Figs. 4, 5). These profiles were consistent with that of the seedling, shoot apex and roots (Endo et al. 2014, Takahashi et al. 2015). Circadian arrhythmic profiles were revealed in the flowers of pcl1-1 and prr975, as reported above regarding seedlings and plant organs (Fig. 4). In contrast to several reports using Arabidopsis seedlings of prr975 mutants, in which the LHY expression increases, our result using buds of the mutants showed a severe reduction in LHY expression (Farré et al. 2005, Nakamichi et al. 2005, Nakamichi et al. 2010; Fig. 4). This suggests that the regulation of LHY expression in flowers is different from that of seedlings. It is probably related to the maintenance of the identity of the flower organs. LHY is involved in temperature compensation in the high-temperature range of the circadian cycle (Gould et al. 2006), further suggesting the importance of LHY expression in flowers.
Despite the different effects of the LHY expression level on prr975 flowers, the total results support the functional importance of core clock genes in regulating flower opening and closing. The data also suggest that feedback loops of day- and evening-expressed core clock genes, PRRs and PCL1/LUX, respectively, influence the regulations of CCA1/LHY, as in other organs (Nohales and Kay 2016).
Arabidopsis petals wilt within 2 d of flowering (Müller 1961). The duration from petal elongation to wilting is primarily determined by the ethylene signaling pathway (Rogers 2013, Chen et al. 2015). Excised petals exhibited circadian rhythms in bioluminescence longer than double the duration (Fig. 5). Even though the rhythmic amplitude gradually declined, this might reflect the cellular level of adenosine triphosphate, which is the substrate of firefly luciferase expressed in plants. The decline also might reflect the state of clock amplitude along with the progress of the senescence program, including cell death. At present, we cannot attribute the significance to the circadian rhythm observed for excised petals. However, we presumed that the clock in the petal may influence senescence since the circadian transcription factor PRR9 activated senescence in the leaves (Kim et al. 2018).
As described above, flower and leaf movements display three key properties: direction, reversibility and phases. By contrast, petals show unique adaptations in gene expression such that flower movement may be related to the regulatory mechanism controlling flower size (Varaud et al. 2011). Therefore, further study of differential growth is essential between the adaxial and abaxial cell layers in petals to understand mechanical aspect of circadian or light-sensing flower movement.
Core clock genes functioned to limit the opening time in flowers under experimental conditions with or without a dark-to-light transition (Figs. 2, 3). This may contribute to limit the visiting of insects. A related pollinator limitation strategy is the formation of closed flowers, i.e. cleistogamy (Culley and Klooster 2007; Fig. 6A). Even though cleistogamy leads to the disadvantage of inbred weakness, it confers a reduction in the biotic and abiotic stresses encountered during seed production (van Doorn and Kamdee 2014). Another limitation caused by minimal insect interaction is that some plant species close their flowers after outcrossing fertilization (Fig. 6B). In contrast to the above two types of closing, Arabidopsis flowers closing by clock signaling is genetically programmed (Fig. 6C). We propose that this is also a type of insect-limiting strategy. In this hypothesis, circadian gating shortens the amount of time the flower remains open, possibly so plants can reduce the amount of damage caused by insects and potentially harmful bacteria because the flowers emit molecules (e.g. sesquiterpene) targeting microorganisms (Huang et al. 2012).
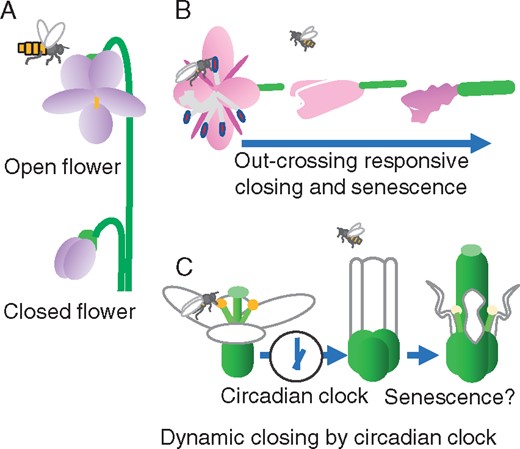
Representative flower closing mechanisms in angiosperms. (A) Open and closed Viola flowers. The open flower is visited by pollinators. While the closed flower never opens, seeds are produced by self-pollination in the secure space (Mayers and Lord 1983). (B) Chamerion angustifolium flower closing is triggered by outcrossing pollination, as compared to self-pollination (Clark and Husband 2007). (C) Arabidopsis flower closing consists of two steps. In the daily light period, the circadian clock in petals measures the time for flower closing and, subsequently, senescence starts. Arrows show the transition until senescence.
Even though the primary function of petals in Arabidopsis might be an attractive visual signal for pollinators, flower closing may suppress abiotic stress (e.g. heat stress) to pistils after fertilization (Zinn et al. 2010, Endo et al. 2013). According to the day and night behaviors in generalist and specialist pollinators, large and conspicuous flowers in Eustoma and wild Nicotiana species respond to diurnal light/dark transitions (and vice versa) and close (Bai and Kawabata 2015, Yon et al. 2016). However, in the context of defensive petal closing in Arabidopsis, closing in advance of the day-to-night transition would be beneficial to reproductive fitness. Regarding Arabidopsis closing in the light period, daylily species, Iris domestica and Hemerocallis fulva, also open their flowers early in the morning and close before dusk (Nitta et al. 2010, Liu et al. 2018). Even though these flowers are large and ornamental, the circadian clock might dominantly regulate flower closing, as in Arabidopsis.
Although the circadian feedback loops in other plant flowers are associated with volatile metabolite release, our study is the first report of clock function in regulating flower opening and closing. The knowledge obtained in Arabidopsis, including the molecular mechanisms of flower opening and closing, may be comparable among unrelated diurnal flowering species (Yon et al. 2016). It appears that the circadian clock preferentially gates flower closing. This preferential gating might be a unique reproductive trait in Arabidopsis. Further studies in Arabidopsis could contribute to the engineering of opening and closing in horticultural and agriculturally valuable plants.
Materials and Methods
Plant materials and culture conditions
We used the Col-0 accession of A.thaliana (Arabidopsis) and its derivative line G38 as wild-types. Plant G38 and the circadian arrhythmic mutant pcl1-1 have been described previously (Onai et al. 2004, Onai and Ishiura 2005). The other arrhythmic mutant prr975 has triple mutations in PRR5, PRR7 and PRR9 loci (Nakamichi et al. 2005). Mutant seedlings of pcl1-1 and prr975 exhibit arrhythmia under both constant light and constant darkness (Onai and Ishiura 2005, Nakamichi et al. 2009). Ler-0 and Ws-4 plants were used as additional accessions of Arabidopsis. Blue light receptor mutant cry1 cry2 and the background accession Col-4 were used. The cry mutant lacks cryptochrome1 and cryptochrom2 in the genome as the alleles of hy4-B104 and cry2-1, respectively, and was obtained from the Chentao Lin laboratory (Mockler et al. 1999). Surface-sterilized seeds were germinated on Murashige and Skoog (MS) agar medium (Murashige and Skoog 1962) supplemented with 2% sucrose. From seedlings to the reproductive stage of growth, plants were cultivated in soil under the long-day conditions (16 h:8 h). Fluorescent tubes were used as a light source with a photon flux density of 50 µmol/m2/s. The temperature was 25°C unless specified. For the experiments, plants were cultivated to form the secondary shoot at the cauline-leaf branch in each plant. Buds of the primary and secondary shoot inflorescences were examined as shown in Supplementary Fig. S2 as the flower opening and closing trait of a single plant. The prr975 mutant usually makes abnormally small buds at the primary shoot inflorescence that do not develop further. Hence, the 2nd and 3rd shoots were examined. Plants were grown under 16 h:8 h in the constant light conditions until just before RNA analysis of flowers. The wild-type was cultivated for 9–11 weeks. pcl1-1 and prr975 mutants were cultivated for 12–14 and 20–22 weeks, respectively.
Flower movement
Image capture of the flower and analysis of petal curvature were performed as follows (other detailed explanations are shown in Supplementary Figs. S1, S2). Flowerpots, each containing a plant and soil, were placed on a stand with a plastic plate and a peduncle-fixing-pad (25 cm height). There are bloomed flowers near buds. Such flowers sometimes move in front of the buds due to the elongation and swirling movements of the flower stalk. Then, to capture bud image in the system, we cut these wilting flowers before the 8-h dark period or the time for readjustment of the stalk during the capturing. In the mutant analysis under the circadian condition, we also cut or carefully identified such flowers. The flowers were traced in the following image analysis procedure. Each plant was supported on the pad at its stem, branch and flower by using pins, enabling photographs to be taken. Mature buds were analyzed for flower movements during opening and closing. We readjusted the flowers on the pad twice a day. An automatic turntable (60 cm diameter) equipped with 20 stands along the rim was constructed. Twenty plants could be placed on the turntable and examined under uniform light conditions. The turntable rotated and then, at each stand, rested so that photographs could be taken with a digital camera (Nikon, Tokyo, Japan, model COOLPIX P7800). Twenty iron tags were attached to the turntable to mark the position of the stands. Accordingly, a magnetic motion sensor with a modified electronic remote release (Nikon, model MD-DC2) sent an electric signal to the camera. The combination of these devices enabled us to produce image files for flowers from 20 plants in an assay. The details are as follows. The turntable moves every 3 min and stops to present the next sample to the camera. Therefore, one cycle of taking 20 samples spends 1 h. Due to this 3-min interval process, the shooting time of the 20th sample delays by 1 h from the 1st. However, in the case in which we tested wild-type and mutants at the same time, we used only 10 stands, i.e. 1–10, such that 0–30 min of data was treated as the ones of the same time. In addition, the wild-types or the mutants were set on odd-numbered or even-numbered stands, respectively, or vice versa, to balance the variation in values due to the difference in shooting time. In addition, the system can shorten the interval between turntable movements by 1 min, allowing each sample to be photographed at short intervals of 20 min. The image analysis processor ImageJ (National Institute of Health, Bethesda, USA) was used to measure the angle of petal curvature between two facing petals. Image file sorting, angle measurement and graph processing assisted us in extracting the diurnal or circadian rhythm from the images of flowers that were obtained. Operation of curvature measurements were manually carried out by using ImageJ in each time-lapse image. That processing software was ordered by Shinsuke Kutsuna and programed in a company (Applitek Co., Ltd, Yokohama, Japan). It can be used on Windows Xp platform with Office applications, Access and Excel software (Microsoft, USA).
Circadian accumulation profiles of the mRNA in core circadian clock genes
Total RNA was extracted from whole flower organs, i.e. buds and flowers at developmental stages 13–15 (Smyth et al. 1990). Flowers were frozen in liquid nitrogen and kept at −80°C. Then, total RNA was extracted with a kit (RNeasy Plant Mini Kit, Qiagen, Dusseldorf, Germany). RLT buffer in the kit was used with 2-mercaptoethanol and polyethylene glycol at a final concentration of 0.01% and 2%, respectively. Total RNA was reverse transcribed with the ExScript RT reagent kit (Takara Bio Inc, Shiga, Japan) according to the instructions by adding 0.1-fold (vol./vol.) of a synthetic RNA solution for the quantitative reaction (RTmate, Nippon Gene Co., Ltd, Japan). Reverse transcription was carried out with 0.1 μg of total RNA and a random hexamer. The obtained cDNA was quantified by real-time qPCR. SYBR FAST qPCR Master Mix (Kapa Biosystems, Wilmington, USA) and MiniOpticon (Bio-Rad Laboratories Inc, Hercules, USA) were used as a fluorescent DNA amplification reporter reagent and real-time fluorescence detecting thermal cycler, respectively. Specific primers for TOC1, LHY and CCA1 genes were used as described in a previous report (Gould et al. 2006). UBQ10 primers were used for normalization among samples as described (Lau et al. 2011). To amplify the product, the following PCR conditions were used: for TOC1 and CCA1, 95°C (3 min), 41 cycles of 95°C (30 s), 54°C (30 s) and 72°C (30 s); for LHY, 95°C (3 min), 43 cycles of 95°C (10 s), 54°C (10 s) and 72°C (30 s); and for UBQ10, 95°C (3 min), 47 cycles of 95°C (10 s), 60°C (10 s) and 72°C (30 s). To check the uniformity of products in each reaction, melting curve analysis in which single peaks responded to heating were obtained under the serial temperature conditions of 95°C (10 s), 67°C (5 s) and 85°C.
Bioluminescence monitoring of the core clock genes
To establish bioluminescence reporter plants for the core clock genes, we transformed the Ler-0 Arabidopsis accession. The CCA1p::LUC+::Tnos reporter DNA cassette consisted of an upstream region of CCA1 (CCA1 promoter; nucleotides 919–2,227 under accession no. U79156), a short linker site of pairing cytosines, followed by the translation initiation codon of a modified firefly luciferase gene (LUC+; Promega, USA) and the transcriptional terminator sequence of Agrobacterium nopaline synthase gene (Tnos). This reporter cassette was inserted into the SmaI site of binary pBIB-Hyg (Becker 1990) in the same direction as an HPT (hygromycin B phosphotransferase) gene cassette, giving pBIB/CCA1::LUC+. We transferred the T-DNA regions of pBIB/CCA1::LUC+ into the genome of wild-type plant Ler-0 using the Agrobacterium tumefaciens-mediated floral dip method (Clough and Bent 1998). We selected plants containing T-DNA in a single locus as a homozygote using the standard technique (Weigel and Glazebrook 2002) and used homozygous T3 plants as CCA1-bioluminescence reporter plants. Two transformant lines were established as genetically independent clones from T1 generation plants. The plant line examined in this study, namely CCA1p:LUC#3, had twice the bioluminescence in seedlings and petals as that of line #14. Two or three plants of each line were used to obtain the average (Table 3). The period and robustness of the circadian bioluminescence rhythms were in the same range. PCL1 and LHY reporter plants were also established. PCL1p:LUC#3 (and #12) and LHYp:LUC#3 (and #6) showed the same levels of bioluminescence and circadian properties in the period and robustness within the derivatives from different T1 plants harboring identical promoter constructs. These plants were subjected to diurnal light cycles of 16L:8D. Two petals were excised from a bud of the developmental stage B3. The petals were inserted vertically at the bases onto MS agar medium containing 0.17 mM d-luciferin potassium salt (FUJIFILM Wako Pure Chemical Co., Osaka, Japan) and 2% sucrose (w/v). After an 8-h dark period, bioluminescence recording started in continuous light conditions. The measures were taken once every 40 min. As described above, we also carried out the establishment of PCL1 (and LHY) reporter plants in the Ler-0 accession by using the DNA construct pBIB/PCL1::LUC+ (Onai and Ishiura 2005) and pBIB/LHY::LUC+. The latter DNA construct harboring the LHYp::LUC+::Tnos reporter DNA cassette consisted of an upstream region of LHY (LHY promoter; nucleotides 37,063–38,913 under sequence ID no. CP002684.1). The nucleotide 37,062 corresponded to the first nucleotide of LHY translational initiation codon. The DNA constructs were used in the transformation in Ler-0 as described above.
Statistical and rhythm analyses
Statistical analysis was implemented in PRISM (GraphPad, http://www.graphpad.com, San Diego, USA, last accessed on April 9, 2021). Bioluminescence profiles were analyzed using tools in BIODARE 2 (https://biodare2.ed.ac.uk; Edingburgh, UK, last accessed on April 9, 2021, Moore et al. 2014). Linear detrended data were applied. The period length was estimated using Fast Fourier Transform Non-Linear Least Squares on the detrended data.
Supplementary Data
Supplementary data are available at PCP online.
Funding
The Japan Society for the Promotion of Science (Grant Number 26650128 to S.K.).
Acknowledgments
We thank Nakamichi Norihito and Takeshi Mizuno for providing prr975 seeds. cry1 cry2 double mutant seeds were provided by Tomonao Matsushita with kindness from Chentao Lin. We are grateful to Wolfgang Engelmann, Dustin Ernst, Tetsu Kinoshita and Michiyuki Yamada for the correction of the manuscript, Kumi Yoshida and Tokitaka Oyama for fruitful discussions and Yusuke Kutsuna for the design of the turntable apparatus.
Disclosures
The authors have no potential conflict of interest with respect to the research, authorship, and/or publication of this article.