-
PDF
- Split View
-
Views
-
Cite
Cite
Aoi Inaji, Atsushi Okazawa, Taiki Taguchi, Masatoshi Nakamoto, Nao Katsuyama, Ryoka Yoshikawa, Toshiyuki Ohnishi, Frank Waller, Daisaku Ohta, Rhizotaxis Modulation in Arabidopsis Is Induced by Diffusible Compounds Produced during the Cocultivation of Arabidopsis and the Endophytic Fungus Serendipita indica, Plant and Cell Physiology, Volume 61, Issue 4, April 2020, Pages 838–850, https://doi.org/10.1093/pcp/pcaa008
- Share Icon Share
Abstract
Rhizotaxis is established under changing environmental conditions via periodic priming of lateral root (LR) initiation at the root tips and adaptive LR formation along the primary root (PR). In contrast to the adaptable LR formation in response to nutrient availability, there is little information on root development during interactions with beneficial microbes. The Arabidopsis root system is characteristically modified upon colonization by the root endophytic fungus Serendipita indica, accompanied by a marked stimulation of LR formation and the inhibition of PR growth. This root system modification has been attributed to endophyte-derived indole-3-acetic acid (IAA). However, it has yet to be clearly explained how fungal IAA affects the intrinsic LR formation process. In this study, we show that diffusible compounds (chemical signals) other than IAA are present in the coculture medium of Arabidopsis and S. indica and induce auxin-responsive DR5::GUS expression in specific sections within the pericycle layer. The DR5::GUS expression was independent of polar auxin transport and the major IAA biosynthetic pathways, implicating unidentified mechanisms responsible for the auxin response and LR formation. Detailed metabolite analysis revealed the presence of multiple compounds that induce local auxin responses and LR formation. We found that benzoic acid (BA) cooperatively acted with exogenous IAA to generate a local auxin response in the pericycle layer, suggesting that BA is one of the chemical signals involved in adaptable LR formation. Identification and characterization of the chemical signals will contribute to a greater understanding of the molecular mechanisms underlying adaptable root development and to unconventional technologies for sustainable agriculture.
Introduction
The rhizosphere is the interface where plant roots interact with both beneficial and pathogenic microorganisms. Beneficial microorganisms include rhizobia and mycorrhizae, and the interactions between symbiotic microbes and host plant roots promote plant growth by inducing the adaptive development of vigorous root systems and by enhancing the capacity of the host plant to acquire nutrients and water from the soil (Mommer et al. 2016, Verbon and Liberman 2016). Root growth promotion is imperative for plants in terms of acquiring water and nutrients, while symbionts also benefit from these interactions by receiving photosynthates from host plants (Verma et al. 1998). There are several symbiotic interactions between plants and soil microorganisms. Obligate biotrophic arbuscular mycorrhizal (AM) fungi establish relationships with >80% of all land plants (Berruti et al. 2016). Ectomycorrhizal fungi colonize host plants through saprotrophic, biotrophic and even parasitic interactions, depending on the environmental conditions (Brundrett 2004, Zuccaro et al. 2014). Moreover, other symbiotic interactions may be nonmycorrhizal but nonetheless promote growth and enhance environmental stress tolerance in host plants (Brundrett 2004). For example, the Brassicaceae is composed of AM hosts, nonhosts and presumed nonhost species (Cosme et al. 2018); nonhost species can associate with a diverse array of nonmycorrhizal root endophytic fungi (Hiruma et al. 2018). It has been suggested that host nutrient status and regulation of secondary metabolic pathways, particularly those of tryptophan-derived secondary metabolites in Brassicaceae species, are critically involved in the interaction processes between plant hosts and pathogenic and beneficial microbes (Hiruma et al. 2016, Hiruma 2019).
Serendipita indica is a root-colonizing endophytic fungus belonging to the order Sebacinales (Verma et al. 1998, Franken 2012, Weiß et al. 2016). It establishes mutualistic interactions with various vascular plants and confers beneficial effects to these plants. A number of important crop species are hosts for S. indica (Waller et al. 2005, Schäfer et al. 2009, Qiang et al. 2012). Root colonization by S. indica has been extensively studied in the model crucifer Arabidopsis thaliana.Jacobs et al. (2011) described the unique S. indica colonization process by S. indica, which involves an initial interaction stage at the root surface, followed by biotrophic colonization; cell death-associated colonization; and static, mutualistic fungal reproduction. In the colonization process, S. indica overcomes the plant root immune response by recruiting jasmonic acid signaling, which, in turn, affects the host defense systems, including chemical defense responses involving glucosinolates (Jacobs et al. 2011). However, it has been reported that Arabidopsis produces indolic secondary metabolites that help maintain mutualistic interaction with S. indica (Nongbri et al. 2012, Lahrmann et al. 2015). Vahabi et al. (2015) reported that S. indica-derived diffusible compounds activate stress/defense responses in plants that were later downregulated at the static interaction stage. Johnson et al. (2018) reported that fungal cell wall-derived cellotriose (CT) induces a defense-like response in Arabidopsis, including reactive oxygen species (ROS) production, changes in membrane potential and the expression of genes involved in growth promotion and root development.
Root architecture in Arabidopsis is noticeably modified, with remarkable lateral root (LR) development, during its interaction with S. indica. The adaptable development of root systems is essential for land plants to acclimate to adverse environmental conditions. The characteristic development of root architecture in response to nutrient availability has been extensively studied at the molecular level (Bouain et al. 2019). However, there is still a lack of information regarding adaptive LR formation induced by endophyte infestation. Sirrenberg et al. (2007) reported that the culture medium of S. indica contains auxin at a level sufficient for LR propagation and primary root (PR) growth inhibition. Meents et al. (2019) showed that the application of S. indica spores induces a rapid but nonspecific auxin response along with PR tissues during the early recognition phase. This auxin response may be involved in the initiation of LR primordia (LRP). It is obvious that endogenous indole-3-acetic acid (IAA) concentrations are modified during symbiotic interactions that induce rhizotaxis (Sukumar et al. 2013). However, it is still unclear whether exogenous auxin is actually involved in the complex system of LR formation/branching. In particular, the mechanism governing auxin responses at the site of LR formation along PRs remains unknown. Thus, exogenously supplied fungal metabolites may be involved in the regulation of auxin responses to initiate LR formation.
In this study, we aimed to clarify the mechanisms responsible for the auxin supply that triggers adaptive LR formation in Arabidopsis as a result of S. indica infestation. The taproot systems of dicotyledonous plants, such as Arabidopsis, consist of LRs and a PR. The molecular mechanisms regulating LR formation within the context of root developmental processes have been explained in detail (Verbelen et al. 2006, Du and Scheres 2018). LR formation proceeds through the meristematic zone at the root tip, the transition zone (TZ), the elongation zone (EZ) and the zone of differentiation (DZ). LR is periodically initiated from xylem pole pericycle (XPP) cells within the TZ/EZ of the PR and specifies LR founder cells (LRFCs) to be converted to LRP (Olatunji et al. 2017, Du and Scheres 2018, Stoeckle et al. 2018). LR initiation sites are recognized by periodic DR5 activation around the TZ, known as the oscillation zone (OZ), which is formed by repeated auxin supplies from the root tip (De Rybel et al. 2012, Du and Scheres 2018). LR formation is also induced in response to dynamic environmental conditions (Zhao 2018).
By monitoring the early responses of auxin accumulation, as indicated by DR5::GUS expression, we found that adaptive LR formation in Arabidopsis is induced by diffusible compounds present in the liquid coculture medium (CCM) of Arabidopsis and S. indica. These diffusible compounds (chemical signals) activate LR formation by inducing the auxin response, as indicated by DR5::GUS expression in specific sets of pericycle layer cells along the PR, resulting in a 2.5-fold increase in LR formation within 24 h, regardless of the positions of the preexisting LRs. Biochemical and molecular genetic studies indicated that neither IAA biosynthesis via the IPyA pathway (Kasahara 2016) nor indole-3-acetaldoxime (IAOx) production participates in the auxin response involved in adaptive LR formation. Metabolite profiling analyses excluded the involvement of endophyte-derived IAA, indolic intermediates of IAA biosynthesis and oligosaccharide elicitors (Johnson et al. 2018) in LR induction. Our results showed the presence of chemical signals that increased the supply of auxin to specific cells in the pericycle layer and induced adaptive LR formation in Arabidopsis. As one of the key compounds, benzoic acid (BA) was identified as a potential chemical signal that stimulates the generation of an auxin maximum at the sites of LR initiation. Here, we discuss auxin biosynthesis and transport and the activities of its related metabolites in terms of their putative contributions to the induction of LR formation in response to S. indica infestation. We also address the molecular mechanisms mediated by the chemical signals underlying adaptive LR formation that establish the plastic development of plant root systems. Molecular identification of the chemical signals that modulate rhizotaxis provides novel data to facilitate the development of alternative strategies for sustainable agricultural production.
Results
Induction of Trp-dependent metabolism and LR formation
In Arabidopsis, Trp-dependent metabolism (Supplementary Fig. S1) plays critical roles in the production of plant defense compounds, such as camalexin, indole glucosinolates and indole cyanohydrins, as well as the phytohormone IAA (Grubb and Abel 2006, Glawischnig 2007, Rajniak et al. 2015). Serendipita indica infestation in Arabidopsis strongly induced GUS expression (Supplementary Fig. S2A) under the control of the ANAC042 promoter (proANAC042::GUS). ANAC042 is a transcription factor that regulates camalexin biosynthesis (Saga et al. 2012). Thus, the chemical defense response in Arabidopsis was, in fact, induced by the infestation of S. indica. The expression of proANAC042::GUS was found to be independent of physical interactions between Arabidopsis and S. indica (Supplementary Fig. S2B). Therefore, we investigated whether physical contact with the endophyte was essential for the induction of plant response. The proANAC042::GUS seedlings at 7 d after germination (DAG) and the S. indica fungal suspension were enclosed in separate dialysis membrane tubes containing PNMP liquid medium. These cultures were incubated in PNMP liquid medium for 4 d (Fig. 1A). The external PNMP medium was then recovered as a CCM. The CCM contained 3 nM camalexin, which was a significantly higher concentration than that in the control PNMP medium (0.03 nM), indicating a substantial induction of camalexin biosynthesis in agreement with the observed induction of proANAC042::GUS. After filter sterilization, CCM was used in the second round of liquid medium culture of proANAC042::GUS seedlings. Within 2 d of cultivation, prominent proANAC042::GUS expression was induced at the root tips of PRs and LRs and this was accompanied by a dramatic increase in LR formation (Fig. 1B). These results indicated that diffusible compounds in the CCM induced both Trp-dependent metabolism (Supplementary Fig. S1) and LR formation in Arabidopsis.
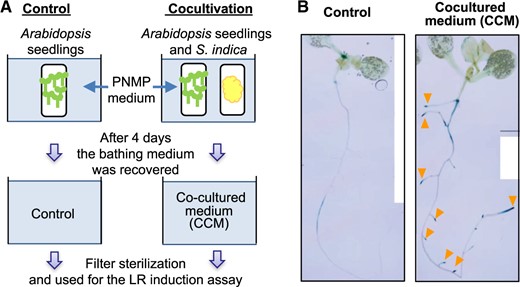
Preparation of the CCM and LR induction by the CCM. (A) For cocultivation in liquid medium, an S. indica suspension and 20 Arabidopsis seedlings (7-DAG) were placed in separate dialysis membrane tubes (Spectrapore; MWCO: 3,500) and cocultured in PNMP liquid media on a rotary shaker (Excella E5) at 80 rpm in a growth chamber (MLR-352-PJ). After cocultivation, the external culture media were recovered as a CCM and as a control medium and sterilized by membrane filtration (EZ-Fit™ Filtration Unit, 0.22 μm) for subsequent experiments. (B) Within 48 h in the CCM, LR formation was markedly induced in the proANAC042::GUS seedlings. The expression of proANAC042::GUS was strongly induced in the root tips of both PRs and LRs (orange triangles).
LR formation assay
The expression of DR5::GUS and DR5rev::GFP reporter constructs (Fig. 2) was induced within 12 h of CCM treatment at the sites of LR formation. Thus, it is possible that CCM treatment may induce LR formation via stimulation of the auxin response. The IAA concentration in the CCM was ∼4 nM (Supplementary Fig. S3), which was much lower than the concentration required for the induction of the auxin response and the additional LR formation (Supplementary Figs. S4, S5). The presence of IAA even at a concentration of 1 nM inhibited PR growth (Supplementary Fig. S6), and PR growth in the CCM (containing ∼4 nM IAA) was also inhibited (Supplementary Fig. S6). In contrast, a high auxin level (1.36 µM after 4 weeks of liquid culture) was reported for S. indica culture medium in a previous study (Sirrenberg et al. 2007). The latter concentration was sufficient to stimulate LR propagation, associated with a very strong inhibition of PR growth (Sirrenberg et al. 2007). Root growth stimulation increased with cultivation time in the CCM (Supplementary Fig. S7). These results indicated that the involvement of S. indica-derived IAA did not explain the root architecture modification caused by the CCM treatment. These findings suggested the presence of unidentified diffusible compounds other than IAA in the CCM that stimulated the auxin response in specific cells in the pericycle layer, leading to additional LR formation. The CCM treatment also stimulated LR formation of Brassica rapa var. laciniifolia (Supplementary Fig. S8). The culture medium of S. indica prepared in the absence of Arabidopsis also showed LR induction activity (Supplementary Fig. S9). Thus, the important chemical signal(s) is thought to be derived from the endophyte. However, it is possible that cocultivation with Arabidopsis may stimulate the production of chemical signals by S. indica. It is also possible that Arabidopsis metabolic activities involved in LR formation may be induced during the cocultivation of S. indica. To pursue these possibilities, we performed additional experiments using coculture media.
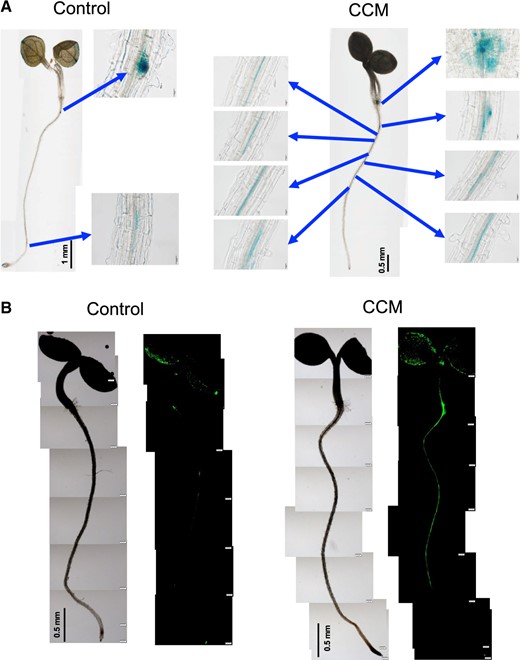
Auxin response visualized using DR5 reporter expression in Arabidopsis. (A) The auxin response sites became noticeable within 12 h in the CCM. Expression of DR5::GUS by the CCM treatment was strongly induced along LRs (blue arrows). (B) The CCM treatment strongly induced the expression of DR5rev::GFP within 6 h along the PR, while the specific sites of LR formation were still indistinguishable.
We prepared an assay system to study stimulated LR formation in greater detail. Figure 3 shows the CCM-dependent induction of LRs in 5-DAG DR5::GUS seedlings. We classified LR formation (Fig. 3A) into three categories based on detailed descriptions of LR development (Malamy and Benfey 1997, Goh et al. 2012): these classifications were ARS (initial auxin response sites marked by DR5::GUS expression before recognizable LRFC formation), LRP (LRFC and LRP) and LR (fully developed and emerged LR). Compared with LR formation stimulated by control PNMP medium, the CCM treatment stimulated LR formation (ARS, LRP and LR) by 2.5-fold in 24 h (Fig. 3B, C). Hereafter, this CCM-dependent LR formation is referred to as ‘additional LR formation’. Additional LRs sometimes formed at positions adjacent to each other (Supplementary Fig. S10). Thus, CCM-dependent LR formation may bypass the control of LR inhibition (Du and Scheres 2018, Toyokura et al. 2019).

CCM-dependent LR formation. (A) LR formation in 5-DAG seedlings of the Arabidopsis DR5::GUS line was analyzed by classifying LR development into one of the three phases: ‘initial auxin response sites’, visualized by DR5::GUS expression; ‘LRP’, later stages of LRP formation; or ‘LR’, clearly visible LRs. Scale bars = 50 μm. (B) Five-DAG seedlings of the DR5::GUS line were incubated either in the control medium or the CCM. The numbers of LR formation sites dramatically increased in the CCM within 24 h. DR5::GUS expression showed the induction of the auxin response at the LR initiation sites. LR formation was observed along the PR from the elongation zone to the maturation zone. LR formation is indicated by red triangles. Scale bars are shown for individual images. (C) LR formation was recorded using 10 seedlings per treatment. The bars indicate the means ± SEs; n = 10 seedlings. The different letters in the stacked bar charts indicate significant differences among the means (P < 0.05 according to Tukey’s test) from the individually scored numbers with respect to ARS, LRP and LR. Significant differences among the total numbers of LRs formed (P < 0.05 according to Tukey’s test) are presented at the bottom.
Involvement of endogenous IAA biosynthesis
We investigated the involvement of IAA biosynthesis induction (Supplementary Fig. S1) in the supply of IAA to specific sections in the pericycle layer (Figs. 2, 3). The major route of IAA biosynthesis (Kasahara 2016) is the IPyA pathway, which comprises two enzymatic steps catalyzed by tryptophan aminotransferase of arabidopsis1/tryptophan aminotransferase related (TAA1/TARs) and flavin-containing monooxygenases (YUCs). The involvement of the IPyA pathway was evaluated using 15 μM l-kynurenine (l-kyn) and 10 μM 4-phenoxyphenyl boronic acid (PPBo), which inhibit TAA1/TARs (He et al. 2011) and YUCs (Kakei et al. 2015), respectively (Fig. 4). Additional LR formation in the control medium was almost completely abolished by l-kyn, and no prominent DR5::GUS induction was detected (Fig. 4A). However, the CCM treatment stimulated additional LR formation in the presence of l-kyn (Fig. 4A). In the presence of PPBo, no additional LR formation was apparent in the control group, but it was significantly stimulated by CCM treatment (Fig. 4B). BBo, another YUC inhibitor (Kakei et al. 2015), provided the same results as did PPBo (data not shown). Another possible route of IAA biosynthesis (Supplementary Fig. S1) involves the production of IAOx by CYP79B2 and CYP79B3. IAOx is the key intermediate involved in the biosynthesis of defense compounds and IAA (Supplementary Fig. S1). CCM treatment strongly upregulated proCYP79B2::GUS (Supplementary Fig. S11), in agreement with the observed increase in camalexin levels in the CCM. In the cyp79b2 cyp79b3 double mutant, however, additional LR formation was stimulated by the CCM treatment (Fig. 4C). In summary, neither the IPyA route nor the IAOx pathway was involved in the local auxin response accompanying additional LR formation. These results suggested that unknown biosynthetic pathways of IAA or an unknown auxin-like compound may contribute to the stimulation of the auxin response and the activation of LR formation. Another possibility is that CCM treatment may facilitate auxin transport to increase local auxin concentrations at the sites of LR formation.
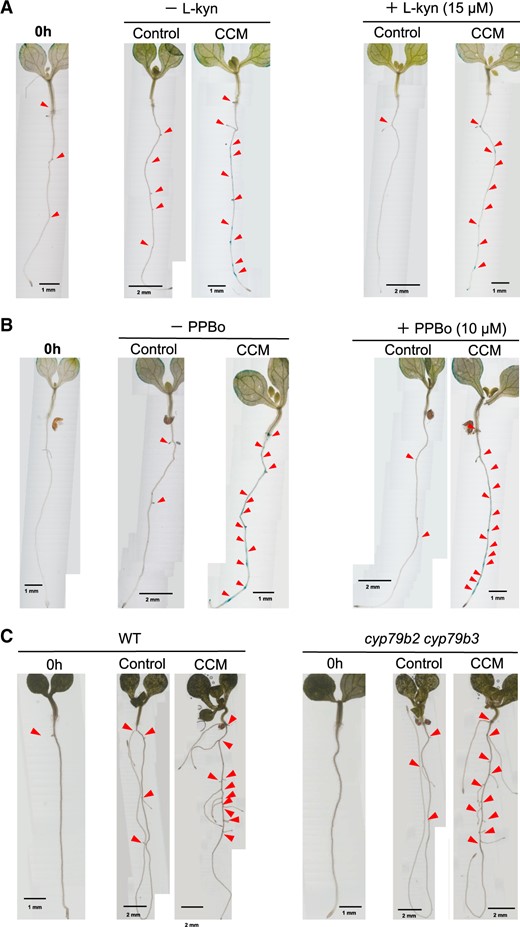
Effects of inhibitors of IAA biosynthesis on CCM-dependent LR formation. (A) LR formation was analyzed using 5-DAG seedlings of the Arabidopsis DR5::GUS line. The seedlings were incubated in the CCM for 24 h in the presence or absence of 15 μM l-kyn, an inhibitor of TAA1/TARs (Supplementary Fig. S1). LR formation is indicated by red triangles. Scale bars are shown for individual images. (B) LR formation was analyzed using 5-DAG seedlings of the Arabidopsis DR5::GUS line. The seedlings were incubated in the CCM for 24 h in the presence or absence of 4-PPBo, an inhibitor of TAA1/TARs (Supplementary Fig. S1). LR formation is indicated by red triangles. Scale bars are shown for individual images. (C) LR formation was analyzed using 5-DAG seedlings of cyp79b2 cyp79b3, an Arabidopsis double mutant defective in the pathway involving IAOx and indole-3-acetonitrile (IAN) intermediates. LR formation in the mutant line was induced in the CCM for 96 h in the same way as it was for the wild type seedlings. LR formation is indicated by the red triangles. Scale bars are shown for individual images.
The auxin supply facilitates LR formation
To clarify the auxin supply source, CCM treatment was performed with root segments without shoots and root tips. Shoot-derived auxin is required for LR formation in Arabidopsis (Bhalerao et al. 2002), and the root tip plays a key role in IAA supply and triggers oscillating LR initiation (Casimiro et al. 2001, Bhalerao et al. 2002, Xuan et al. 2015). After 24 h, root segments treated with CCM showed an initial auxin response and LR initiation (Fig. 5A). Thus, neither shoot-derived IAA nor root tip-derived IAA participated in additional LR formation. This auxin response in root segments was also observed in the presence of L-kyn and PPBo (data not shown), indicating that the IPyA pathway is not involved in the auxin response. When additional LR formation was studied in the presence of 10 μM N-1-naphthylphthalamic acid (NPA), which is an auxin transport inhibitor, no additional LRFCs or LRPs formed. Moreover, no clear DR5::GUS signal was observed in the pericycle layer; rather, the DR5::GUS signal was broadly observed throughout the root cortex and endodermis (Fig. 5B). In the absence of NPA, ARSs and LR initiation were restricted to certain sections in the pericycle layer.
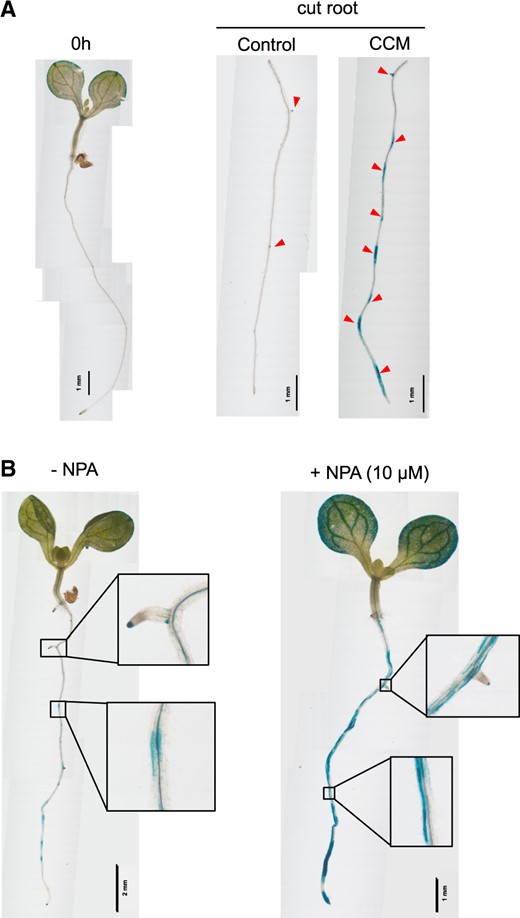
Involvement of auxin transport in CCM-dependent LR formation. (A) LR formation was analyzed using root segments without root tips or shoots. LRs were excised from 5-DAG seedlings of the Arabidopsis DR5::GUS line. The root segments were incubated in either the control medium or the CCM. LR formation and DR5::GUS expression were analyzed 24 h after the transfer of root segments to the test media. The LR formation sites are indicated by red triangles. Scale bars are shown for individual images. (B) Effects of the polar auxin transport inhibitor NPA on LR formation. Five-DAG seedlings of the Arabidopsis DR5::GUS line were incubated in the CCM in the presence or absence of 10 μM NPA. With NPA, no significant LR formation was observed regardless of strong DR5::GUS induction. With NPA, there was no significant DR5::GUS expression in the pericycle layer cells. Rather, it was restricted to the endodermal and cortical cells, as shown in the magnified images. Scale bars are shown for individual images.
Metabolite analysis
CCM may contain metabolites that influence metabolic activities to induce the auxin response or unidentified compounds with auxin-like activity. These compounds may stimulate the auxin response and additional LR formation. Johnson et al. (2018) reported that CT derived from S. indica induces defense responses, such as the production of ROS. CT was detected at ∼50 and ∼20 nM in the CCM and control medium, respectively. Nevertheless, it did not induce additional LR formation at 10 nM, 100 nM or 1 μM (Supplementary Fig. S12). It is well known that exogenous auxin alters root development. For example, 10 μM 1-naphthaleneacetic acid, a synthetic auxin, strongly induces LR formation along the PR (Casimiro et al. 2001, Himanen et al. 2002). However, the IAA level in the CCM was <4 nM (Supplementary Fig. S3) and did not induce an auxin response or LR initiation (Supplementary Figs. S4, S5). Indole-3-butyric acid (IBA) and IPyA were not detected in the CCM, while indole-3-lactic acid (ILA) was present at ∼0.2 nM. Camalexin concentrations in the control medium and CCM were ∼0.03 and ∼1.0 nM, respectively. Neither LR formation nor the auxin response was induced by ILA or camalexin (data not shown). Phenylacetic acid (PAA), another auxin (Kasahara 2016), was not detected in the CCM. However, BA, an allelochemical compound (Zhang et al. 2018), was detected in the CCM (∼1 μM). BA was also detected in the S. indica liquid culture medium (∼1 μM) prepared in the absence of Arabidopsis. Next, the CCM was subjected to ethyl acetate fractionation to identify diffusible compounds. LR initiation activity was recovered in the nonpolar phase (Supplementary Fig. S13), which was then fractionated by reverse-phase HPLC (Fig. 6). The fraction containing the LR formation activity (F3 in Fig. 6) was separated into two peaks (F3a and F3b); the latter was found to be the active fraction for LR formation (Supplementary Fig. S14). Our preliminary liquid chromatography–mass spectrometry/mass spectrometry (LC–MS/MS) analysis revealed that F3b contained IAA (<3 nM) and several unidentified metabolite peaks. The application of 5 nM IAA alone did not initiate LR formation (Fig. 7), indicating that a compound other than IAA in F3 may be responsible for the LR formation activity. To study the concerted effects of different compounds, we coapplied IAA and metabolites detected in the CCM, such as ILA, camalexin and BA. Interestingly, the coapplication of 5 μM BA and 5 nM IAA was sufficient to stimulate LR formation (Fig. 7), although the induction levels did not rise to those induced by CCM application. Since BA itself did not induce LR initiation activity, it may facilitate a transport mechanism to elevate auxin concentrations at specific sites in the pericycle layer, thus enabling additional LR formation. Therefore, BA is thought to be one of the chemical signals involved in LR formation.
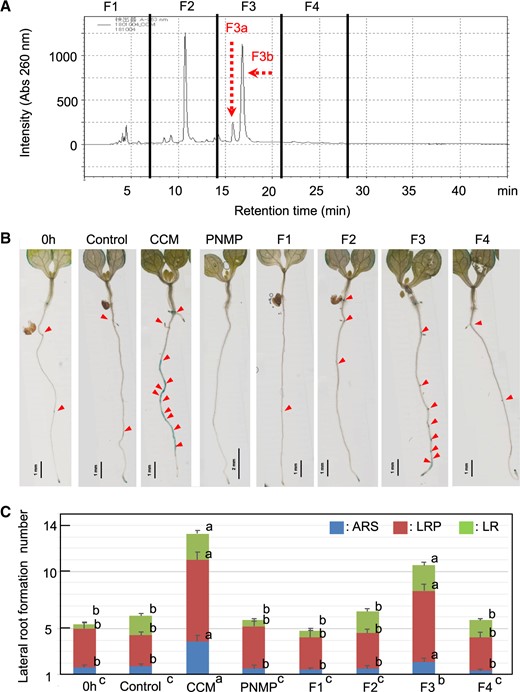
CCM fractionation. (A) HPLC fractionation of the nonpolar phase. The eluate was recovered as four fractions (F1, F2, F3 and F4). Two major peaks observed in F3 were separately analyzed as F3a and F3b for the LR formation assay (Supplementary Fig. S14). (B) The HPLC fractions were evaporated to dryness, dissolved in ethanol and diluted with the PNMP medium used to grow the 5-DAG seedlings of the Arabidopsis DR5::GUS line for 24 h. LR formation activity was recovered in the F3 fraction. (C) Numbers of LR formation sites at 24 h after cultivation. LR formation was classified as shown in Fig. 3. The bars indicate the means ± SEs; n = 10 seedlings. The different letters in the stacked bar charts indicate significant differences among the means (P < 0.05 according to Tukey’s test) from the individually scored numbers with respect to the ARS, LRP and LR. Significant differences among the total numbers of LRs formed (P < 0.05 according to Tukey’s test) are indicated in the labels of the different experiments at the bottom.
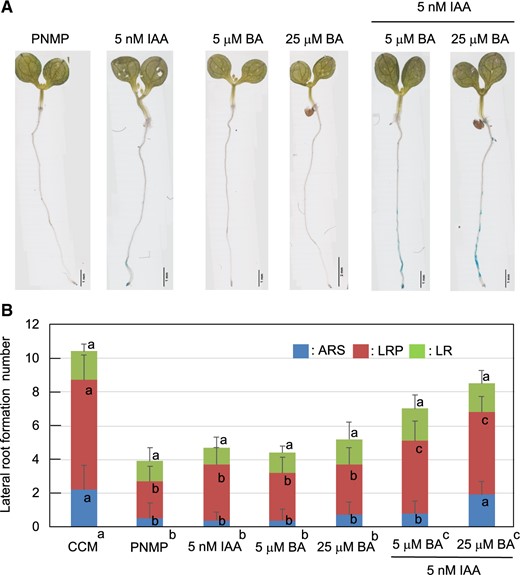
Induction of LR formation by simultaneous applications of IAA and BA. (A) LR formation was analyzed using 5-DAG DR5::GUS seedlings. The seedlings were incubated for 24 h in PNMP liquid media consisting of either 5 nM IAA, 5 µM BA, 25 µM BA, 5 nM IAA with 5 µM BA or 5 nM IAA with 25 µM BA. The auxin response and LR formation were strongly induced by the simultaneous application of IAA and BA. Individual applications of either 5 nM IAA, 5 µM BA or 25 µM BA did not induce LR formation. (B) Numbers of LR formation sites at 24 h after cultivation. LR formation was classified as shown in Fig. 3. The bars indicate the means ± SEs; n = 10 seedlings. The different letters in the stacked bar charts indicate significant differences among the means (P < 0.05 according to Tukey’s test) from the individually scored numbers with respect to the ARS, LRP and LR. Significant differences among the total numbers of LRs formed (P < 0.05 according to Tukey’s test) are indicated in the labels of the different experiments at the bottom. The total LR formation number induced by the CCM was significantly larger than that recorded in response to the simultaneous treatment of BA and IAA.
Discussion
The additional LR formation induced by coculture media (Fig. 2) exemplifies the adaptive LR development induced during interactions with endophytes. Here, we showed the presence of diffusible compounds (chemical signals) responsible for the induction of local auxin responses in specific sets of pericycle layer cells to enable additional LR formation. These chemical signals may influence the developmental program responsible for LR formation. In general, periodic LR initiation and prepatterning (Verbelen et al. 2006, Du and Scheres 2018) occur in XPP cells of the root tips. LR initiation sites are recognized by periodic DR5 activation around the TZ in the OZ. The OZ is formed by repeated auxin supplies from the root tip, where IBA is converted to IAA in concert with recurrent programed cell death (De Rybel et al. 2012, Du and Scheres 2018). The OZ visualized by DR5 expression stabilizes in the early DZ to form ‘prebranch sites’ that are converted to LRFCs. These sites, in turn, are activated to initiate LRs in the DZ. LR initiation from the prebranch sites depends on polar auxin transport between the cotyledon and root tip (Laskowski et al. 2008, Lucas et al. 2008, Ivanchenko et al. 2010). In fact, exogenously applied auxin does not induce new prebranch sites in the OZ (Moreno-Risueno et al. 2010). However, with prolonged exposure to 10 μM auxin, LR development is initiated along the PR (Himanen et al. 2002). Thus, XPP cells maintain their competency for additional LR initiation. Dubrovsky et al. (2008) reported that additional LRP forms from auxin-producing pericycle sections. Therefore, local increases in auxin concentrations may specify LRFCs independent of the root development stage and LRFCs marked by DR5 expression sites are converted to LRP.
CCM-dependent LR formation
Meents et al. (2019) reported that the application of S. indica spores enhanced the fluorescence signals of DR5::EGFP-DR5v2::tdTomato along the PR in a tissue-nonspecific manner during the early interaction phases. The DR5-dependent expression of the reporter genes was also induced by the application of exogenous IAA at 5 μM. In both cases, fluorescence signals were detected throughout the entire root tissue, without cell- or tissue-specific expression patterns. Thus, exogenous IAA and the presence of S. indica within root tissues can induce auxin-responsive gene expression in a nonspecific manner along the PR. In our experiments, the IAA concentration in the CCM was <4 nM. Neither DR5-responsive reporter gene expression nor LR initiation was induced by the application of 5 nM IAA (Fig. 7). Thus, the CCM-dependent initiation of LR formation cannot be explained in terms of developmental process intervention by IAA derived from S. indica. Meents et al. (2019) suggested that unidentified signals derived from S. indica activate auxin-responsive reporter genes. Therefore, there must be as yet unknown mechanisms that control the accumulation of auxin at the sites of LR formation.
CCM-dependent LR formation may be initiated through the stimulation of local auxin supplies to pericycle cells. In our experiments, auxin transport from the shoots and root tips was not necessary for the CCM-dependent induction of DR5::GUS or for LR formation (Fig. 5A). Thus, additional LR formation (Figs. 2, 3) could not be simply explained in terms of periodic LR initiation and prepatterning and there must be CCM-dependent mechanisms to stimulate the auxin response in the pericycle layer cells for the initiation of additional LR formation. All DR5-expressing sections induced by the CCM treatment could be converted to LRFCs. However, CCM treatment in the presence of NPA broadly induced DR5 expression in the cortex and endodermis, but not specifically in the pericycle layer (Fig. 5B), and additional LR formation was not initiated. It is possible that unidentified local auxin production pathways may be activated by the CCM in the root cortex and/or endodermal cells. Otherwise, an unidentified transport system may be facilitated by diffusible compounds, resulting in the accumulation of auxin at specific cells within the pericycle layer to initiate LR formation. These observations suggest that auxin transport may be facilitated by the CCM along with the pericycle layer that is preserved for the future specification of LRFCs. It remains to be determined which cells or sections were responsible for DR5::GUS expression preceding LR formation.
Source of auxin
Chemical signals stimulated the auxin responses in the pericycle layer cells along the PR (Figs. 2, 3). However, as described above, the CCM contained IAA at a concentration of <4 nM (Supplementary Fig. S3), which is too low to induce LR formation. It is, therefore, possible that these chemical signals may activate endogenous auxin biosynthesis. However, inhibitor studies excluded the involvement of the IPyA and IAOx pathways in IAA production and additional LR formation (Fig. 4). Thus, we explored the possibility that IAA biosynthesis precursors present in the CCM may be involved in the induction of the auxin response at sites of LR formation. The CCM contained ILA (<0.2 nM) and camalexin (<1 nM). Neither ILA nor camalexin induced LR initiation in 5-DAG Arabidopsis seedlings (data not shown). Moreover, we did not detect other metabolites, such as indole-3-acetamide, IAOx, IBA, IPyA, N-hydroxytryptamine, indole-3-acetonitrile (IAN), tryptamine or PAA, in the CCM. Therefore, these metabolites are unlikely to be the chemical signals that induce or act as precursors to the induction of additional LR formation. It is possible that an unidentified auxin biosynthetic pathway may have been activated by the CCM. A possible alternative IAA source may be indole synthase (INS); however, there is no consensus regarding the role of INS in auxin biosynthesis or plant development (Zhao 2018). PAA biosynthesis may be involved in additional LR initiation, but its biosynthetic pathway has not yet been elucidated (Kasahara 2016, Cook et al. 2016). Thus, molecular genetic approaches are not applicable for studying the possible involvement of PAA in CCM-dependent LR formation.
Environmental factors, such as nutrient availability and water supply, affect auxin sensitivity involved in the LR formation processes (Motte et al. 2019). For example, phosphate starvation conditions induce the expression of auxin receptor gene TRANSPORT INHIBITOR RESPONSE1 (TIR1) in pericycle cells responsible for LR formation (Pérez-Torres et al. 2008). Thus, it is possible that the chemical signals may directly enhance local sensitivity to auxin or induce the expression of auxin-related genes, such as TIR1, and the transcription factors belonging to the AUXIN RESPONSE FACTOR family. It is also possible that the chemical signals(s) may facilitate auxin transport and contribute to the generation of a local auxin maximum, leading to the initiation of additional LR formation.
Chemical signals
Exogenously supplied IAA did not specifically induce DR5-dependent reporter expression at the sites of LR formation (Meents et al. 2019). In addition, LR formation induced by the CCM treatment was independent of the major IAA biosynthetic pathways (Fig. 4). These observations support the idea that CCM-dependent LR formation may be induced by unknown chemical signals. Johnson et al. (2018) reported that the application of 0.1 μM CT, an elicitor derived from S. indica, evokes a transient increase in cellular [Ca2+] in Arabidopsis and upregulates genes involved in plant defense, growth promotion and root development. Among these CT-induced genes is a respiratory burst oxidase homolog (RBOHD). RBOHD is responsive to IAA and is expressed during the late stages of LR formation, producing ROS to facilitate LR outgrowth. However, in the CCM, the CT concentration was <50 nM and CT application did not induce additional LR formation (Supplementary Fig. S12). Therefore, the chemical signal in the CCM is not S. indica-derived CT.
Metabolite profiling analysis revealed BA in both the CCM and an S. indica liquid culture medium. Phenolic allelochemicals, cis-cinnamic acids and BA alter auxin homeostasis and root development (Wasano et al. 2013, Steenackers et al. 2017). Zhang et al. (2018) reported that exogenous BA inhibits PR elongation by modulating auxin accumulation via the stimulation of IAA biosynthesis and transport. However, the involvement of BA in adaptive LR formation has not yet been investigated. In this study, we found that the coapplication of 5 μM BA with 5 nM IAA stimulated LR formation (Fig. 7). However, LR initiation was not induced when either 5 μM BA or 5 nM IAA was supplied independently. A BA-facilitated IAA transport system may contribute to generating auxin concentration gradients within specific sets of XPP cells awaiting conversion to LRs (Fig. 7). Thus, BA is purported to be one of the chemical signals involved in additional LR formation. However, it is important to note that the BA concentration detected in the CCM (∼1 μM) was not sufficiently high to exert a concerted effect with IAA to induce LR formation. Nonetheless, we cannot rule out the possibility that unknown metabolites related to BA may be the true compound in the CCM that exerted LR induction activity.
Specific cells in the pericycle layer maintain the capability of accumulating auxin in response to chemical signals to initiate LR development. In this study, the xylem and phloem poles were not clearly distinguished as the sites of the initial DR5::GUS expression (Fig. 5). Clarification of the cells responsible for the initial auxin response is essential for understanding the mechanisms involved in additional LR formation. Identification of other chemical signals from S. indica is crucial for elucidating the entire scenario underlying adaptive rhizotaxis during interactions with beneficial soil microbes. Arabidopsis mutants defective in CCM-dependent LR formation will provide essential information for this purpose. Furthermore, molecular identification of the chemical signals may provide an alternative approach to improving root architecture for soil ecosystem health and sustainable crop productivity.
Materials and Methods
Plant materials
All experiments were performed on A. thaliana ecotype Columbia (Col-0). The Arabidopsis lines used in this study included proANAC042::GUS (Saga et al., 2012), DR5rev::GFP (Friml et al. 2003), DR5::GUS (Ulmasov et al. 1997), proCYP79B2::GUS (Ljung et al. 2005) and the cyp79B2 cyp79B3 double mutant (Sugawara et al. 2009). The plants were grown in a growth chamber (MLR-352-PJ; Panasonic Health Care, Tokyo, Japan) maintained at 23°C under a 16-h light/8-h dark photoperiod (140–160 μmol photons m−2 s−1). Under sterile conditions, seeds were germinated on agar (0.9% w/v) plates containing germination medium composed of 1/2-strength Murashige and Skoog salts and 1% (w/v) sucrose.
Serendipita indica inoculation
Serendipita indica (Mycobank No. 812127; Weiß et al. 2016) was maintained at 28°C on 1.5% (w/v) agar plates containing complete media (Hill and Kafer 2001). Serendipita indica was cultured for 7 d in the modified PNM liquid media (pH 5.7) consisting of 5 mM KNO3, 2 mM MgSO4, 2 mM Ca(NO)3, 70 μM H3BO3, 14 μM MnCl2, 0.5 μM CuSO4, 1 μM ZnSO4, 0.2 μM Na2MoO4, 10 μM FeSO4 and 10 nM CoCl2. For plant cocultivation, the modified PNM liquid medium (PNMP medium) was supplemented with 1 mM KH2PO4. For inoculation studies, S. indica was collected on filter paper and fungal pellets were resuspended and diluted in liquid PNMP media for inoculation. For direct inoculation studies on PNMP agar, S. indica was applied via filter paper on 10-DAG Arabidopsis seedlings containing a proANAC042::GUS expression cassette (Saga et al. 2012). For indirect inoculation studies, 10-DAG Arabidopsis seedlings were covered with nylon membranes (0.45 μm; GE Health Care Japan, Tokyo, Japan) and overlaid with 1-cm2 filter paper transfused with 20 μl of S. indica suspension (Supplementary Fig. S2). For cocultivation in liquid media (Fig. 1), a fungal suspension (1 g FW) and 20 Arabidopsis seedlings (7-DAG) were placed in separate dialysis membrane tubes (Spectrapore; MWCO: 3,500; Funakoshi Co., Ltd., Tokyo, Japan) and cocultured in 400 ml of liquid PNMP media on a rotary shaker (Excella E5; Eppendorf, Tokyo, Japan) at 80 rpm in an MLR-352-PJ growth chamber. After cocultivation, the external culture media were recovered as a CCM and a control medium and were sterilized by membrane filtration (EZ-Fit™ Filtration Unit, 0.22 μm; Merck Millipore, Tokyo, Japan) for subsequent experiments.
LR initiation assay
Five-DAG Arabidopsis seedlings were used to evaluate LR formation in 12-well cell culture plates. Four seedlings were placed in each well containing 3 ml of either the CCM or control liquid medium. The plates were then incubated in an MLR-352-PJ growth chamber. Assays were performed in the presence or absence of the auxin flux inhibitor NPA. The involvement of the endogenous IAA biosynthetic pathway was studied using the following inhibitors of enzymes in the IPyA pathway: PPBo, 4BBo and l-kyn (He et al. 2011, Kakei et al. 2015). Root growth was also studied in the presence or absence of exogenously applied CT (Tokyo Chemical Industries, Co., Ltd., Tokyo, Japan). GFP was visualized with a DP72 image analyzer (IX81 and SZX61; Olympus, Tokyo, Japan), and histochemical analysis of DR5::GUS expression was performed according to a previously described method (Saga et al. 2012).
Fractionation of the CCM
The CCM (100 ml) was subjected to ethyl acetate extraction. The nonpolar phase was evaporated to dryness and subsequently dissolved in 4 ml of ethanol. This solution was then passed through a polytetrafluoroethylene (PTFE) filter (0.45 μm; Advantec, Toyo Roshi Kaisya, Ltd., Tokyo, Japan) and fractionated via an LC-10AT HPLC system (Shimadzu Corporation, Kyoto, Japan) fitted with an octadecyl silica (ODS) column (Kinetex C18; particle diameter 5 μm; 50 mm × 21.2 mm; Phenomenex, Torrance, CA, USA). Elution was performed with solvent A (0.1% formic acid) and solvent B (0.1% formic acid in acetonitrile) using the following gradient elution method (flow rate: 3 ml min−1): 0–2 min, 10% B; 2–32 min, 70% B; 32–35 min, 95% B; 35–37 min, 95% B; 37–40 min, 10% B; and 40–42 min, 10% B. Fractions were collected every 7 min and evaporated to dryness. The samples were dissolved in 50 μl of ethanol for the LR formation assay. F3 from the nonpolar phase (Fig. 6) was evaluated by chromatography in a Nexera X2 UHPLC system equipped with an SPD-M30A diode array detector (Shimadzu Corporation). Chromatographic separation was performed using a Kinetex C18 column (2.6 µm, 150 mm × 2.1 mm, Phenomenex) by linear gradient elution (0.3 ml min−1) with solvent A (0.1% formic acid) and solvent B (0.1% formic acid in acetonitrile) as follows: 0–2 min, 15% B; 2–32 min, 45% B; 32–35 min, 45% B; 35–37 min, 95% B; 37–40 min, 95% B; and 40–42 min, 15% B. The eluate was evaporated to dryness and then dissolved in 50 μl of ethanol. For the LR formation assay, the concentrated materials were diluted by 200-fold in a PNMP medium.
Metabolite analyses
Plant roots (300 mg) were frozen, pulverized in liquid N2 and suspended in 80% (v/v) methanol. The homogenates were then centrifuged at 12,000 × g for 15 min at 4°C. The supernatant was extracted with 67% methanol:chloroform, and the aqueous phase was recovered and filtered through disposable PTFE membrane filter units (DISMIC-13JP 0.20 µm, Advantec), evaporated under N2 and stored at −80°C until use. The metabolites were analyzed using a Shimadzu LC–MS 8040 system with a Nexera X2 UHPLC instrument (Shimadzu Corporation) and a reverse-phase column (Kinetex C18; 2.6 µm, 150 mm × 2.1 mm; Phenomenex), with a column temperature of 40°C. Elution was performed with solvent A (0.1% formic acid) and solvent B (0.1% formic acid in acetonitrile) by the gradient elution method (flow rate: 0.3 ml min−1) as follows: 0–3 min, 15% B; 9 min, 65% B; 10 min, 5% B; 12 min, 95% B; 13 min, 15% B; and 15 min, 15% B. The metabolites were identified by the multiple reaction monitoring (MRM) method for camalexin (positive mode, MRM at m/z = 176.05 > 130.15), IAA (positive mode, MRM at m/z = 176.05 > 130.15), IBA (positive mode, MRM at m/z = 204.10 > 186.10), ILA (negative mode, MRM at m/z = 204.05 > 158.95) and IPyA (negative mode, MRM at m/z = 202.05 > 158.25). Selected ion monitoring (SIM) was performed for PAA (negative mode, SIM at m/z = 135.05) and BA (negative mode, SIM at m/z = 121.35). Deuterated IAA (indole-3-acetic-2,2-d2 acid; Sigma-Aldrich Corp., Tokyo, Japan) was added as an internal standard (positive mode, MRM at m/z = 178.05 > 132.15) for precise IAA determination in the culture medium. The CCM was extracted with ethyl acetate and evaporated to dryness. The dried materials were subsequently dissolved in ethanol and subjected to LC–MS analysis. Indole-3-acetamide, N-hydroxyl tryptamine, IAOx, indole-3-acetonitrile, dihydrocamalexic acid, indole-3-acetaldehyde, indole cyanohydrin, indole-3-cyanohydrin and indole-3-carbonyl nitrile were monitored by the SIM method using monoisotopic mass values. For the analysis of oligosaccharides, including CT, the CCM was lyophilized and dissolved in 50% acetonitrile. The sample was then subjected to an LC–MS instrument (LC-MS 8040 with Nexera X2; Shimadzu Corporation) equipped with an Acquity UPLC BEH amide column (1.7 µm, 2.1 mm × 150 mm; Waters Corp., Milford, MA, USA). Elution was performed in a linear gradient at a flow rate of 0.3 ml min−1 using acetone:acetonitrile (1:1) in 0.2% NH4OH as solvent A and 0.2% NH4OH as solvent B (0–2 min, 15% B; 2–22 min, 35% B; and 22–24 min, 10% B).
Chemical compounds
The 5-[4-chlorophenyl]-2,4-dihydro-[1,2,4]-triazole-3-thione (camalexin), IAA, IBA, ILA, indole-3-pyruvic acid, 4-biphenyl boronic acid, 3-chloro-4-hydroxyphenylacetic acid, 1-naphthoxyacetic acid, l-kynurenine, NPA, 4-phenoxyphenyl boronic acid and 3-chloro-4-hydroxyphenylacetic acid compounds used in the experiments were obtained from Sigma-Aldrich Corp. PAA was obtained from Wako Pure Chemical Industries, Ltd. (Osaka, Japan). The oligosaccharide standard compounds were obtained from Tokyo Chemical Industry Co., Ltd.
Supplementary Data
Supplementary data are available at PCP online.
Acknowledgments
We thank Hiroyuki Kasahara and John Celenza for sharing published materials and Takumi Ogawa for helping with S. indica cultivation.
Funding
Grants-in-Aid for Scientific Research of MEXT Japan (16K13090).
Disclosures
The authors have no conflicts of interest to declare.
References
Author notes
Aoi Inaji and Atsushi Okazawa contributed equally to this work.