-
PDF
- Split View
-
Views
-
Cite
Cite
Xinyi Yu, Yingjun Hou, Weiping Chen, Sanhong Wang, Peihong Wang, Shenchun Qu, Malus hupehensis miR168 Targets to ARGONAUTE1 and Contributes to the Resistance against Botryosphaeria dothidea Infection by Altering Defense Responses, Plant and Cell Physiology, Volume 58, Issue 9, September 2017, Pages 1541–1557, https://doi.org/10.1093/pcp/pcx080
- Share Icon Share
Abstract
MicroRNA (miRNA)-mediated post-transcriptional regulation plays a fundamental role in various plant physiological processes, including responses to pathogens. MicroRNA168 has been implicated as an essential factor of miRNA pathways by targeting ARGONAUTE1 (AGO1), the core component of the RNA-induced silencing complex (RISC). A fluctuation in AGO1 expression influences various plant–pathogen interactions, and the homeostasis of AGO1 and miR168 accumulation is maintained by a complicated feedback regulatory loop. In this study, the connection between miR168 and the resistance of Malus hupehensis to Botryosphaeria dothidea is revealed. The induction of both the mature miR168 and its precursor in plants subjected to B. dothidea infection indicate the transcriptional activation of MIR168a. MIR168a promoter analysis demonstrates that the promoter can be activated by B. dothidea and salicylic acid (SA). However, the direct target of miR168, M. hupehensis ARGONAUTE1 (MhAGO1), is shown to be induced under the infection. Expression and transcription activity analysis demonstrate the transcriptional activation and the post-transcriptional suppression of MhAGO1 in response to B. dothidea infection. By inhibiting reactive oxygen species (ROS) production and enhancing SA-mediated defense responses, miR168a delays the symptom development of leaves inoculated with B. dothidea and impedes the pathogen growth, while MhAGO1 is found to have the opposite effects. Collectively, these findings suggest that the expression of miR168 and MhAGO1 in M. hupehensis in response to B. dothidea infection is regulated by a complicated mechanism. Targeting to MhAGO1, a negative regulator, miR168 plays a positive role in the resistance by alterations in diverse defense responses.
Introduction
Current research has demonstrated that microRNA (miRNA)-mediated post-transcriptional regulation plays a central role in the modulation of plant physiological processes, including growth and development, hormone signaling transduction and stress responses (Chen 2009, Voinnet et al. 2009, Rubio-Somoza et al. 2011, Khraiwesh et al. 2012, Sunkar et al. 2012). A large body of evidence has revealed the significance of miRNAs in the response of plants to abiotic stresses, such as salt, drought, heat, cold, nutrient deficiency and metal toxicity (Liu et al. 2008, Jia et al. 2009, Kawashima et al. 2009, Zhang et al. 2013). Recent efforts have focused on investigating the function of miRNAs in plant response to biotic stresses. A number of miRNAs have been identified and functionally analyzed for their role in plant responses to a variety of different pathogens, including viruses (Kasschau et al. 2003, Bazzini et al. 2007, Csorba et al. 2007, Varallyay et al. 2010), bacteria (Navarro et al. 2006, Jagadeeswaran et al. 2009, Li et al. 2010, W. Zhang et al. 2011, X. Zhang et al. 2011) and fungi (Lu et al. 2007, Xin et al. 2010, Chen et al. 2012, Zhao et al. 2012, Li et al. 2014, Shen et al. 2014). The pathogen-induced miRNAs have been implicated as important regulators in multilevel defense responses, including pathogen-associated molecular pattern (PAMP)-triggered immunity (PTI) (Padmanabhan et al. 2009, Li et al. 2010, X. Zhang et al. 2011), effector-triggered-immunity (ETI) (Navarro et al. 2008, Zhai et al. 2011, Shivaprasad et al. 2012) and basal resistance (Navarro et al. 2008). These findings highlight the fundamental role of miRNAs in the interaction of plants with pathogens.
Apple (Malus domestica) is a major fruit crop worldwide. The initial investigation of apple miRNAs was conducted by Gleave et al. (2008). More than 200 conserved or apple-specific miRNAs have now been recognized and experimentally confirmed (Varkonyi-Gasic et al. 2010, Yu et al. 2011, Xia et al. 2012, Ma et al. 2014). The expression of several Malus miRNAs has been reported to be developmentally related (Xing et al. 2014, Xing et al. 2016) and their role in development has been confirmed by ectopic expression in Arabidopsis (Sun et al. 2012, Zhao et al. 2015). Apple miRNAs that respond to Botryosphaeria dothidea (Yu et al. 2014) and fire blight disease (Kaja et al. 2015) have been identified using high-throughput sequencing technology. Ma et al. (2014) identified a novel apple miRNA that targets an NBS gene, and a transient transformation system was utilized to verify its role in Alternaria leaf spot disease. Considering the number of studies that have documented the involvement of miRNA pathways in plant defense responses in other species, however, our current knowledge of Malus defense-related miRNAs is far from complete.
miRNA168 is one of the most commonly detected miRNAs in plant stress responses (W. Li et al. 2012), and has been shown to be induced during the course of infection by diverse pathogens. Mature miR168 is highly conserved in plant species and has been implicated as a critical regulator of miRNA pathways by regulating its exclusive target ARGONAUTE1 (Vaucheret 2006). ARGONAUTE1 (AGO1), as the executor component of the RNA-induced silencing complex (RISC), is considered to be a key component in miRNA pathways (Vaucheret et al. 2004, Voinnet 2009). By binding to the AGO1 protein, most mature miRNAs guide the RISC to cleave directly (Baumberger and Baulcombe 2005, Jones-Rhoades et al. 2006) or translationally inhibit their targets (Lanet et al. 2009).
Evidence for the involvement of AGO1 in diverse plant–pathogen interactions has been documented in numerous studies. AGO1 is considered a key regulator in plant antiviral response. AGO1 protein recruits viral-derived small interfering RNAs (vsiRNAs), resulting in the ability of plants to inactivate viral RNAs (Zhang et al. 2006, Burgyan and Havelda 2011). Furthermore, an Arabidopsis hypomorphic mutant ago1 is more susceptible to viral infection (Morel et al. 2002, Qu et al. 2008). Li et al. (2010) demonstrated that AGO1 is necessary for a series of bacteria-related PTI responses. Arabidopsis ago1 plants also exhibit enhanced resistance to Verticillium dahliae (Ellendorff et al. 2009, Shen et al. 2014). Moreover, Weiberg et al. (2013) demonstrated that Botrytis cinerea is capable of hijacking the plant RNA interference (RNAi) pathway and inhibiting defense responses by producing AGO1-binding small RNAs. Based upon these findings, the modulation of miR168 and AGO1 expression appears to have a significant impact on plant resistance to pathogen infection.
The regulation of AGO1 mRNA expression by miR168 (Vaucheret et al. 2004) and AGO1-derived siRNAs (Mallory and Vaucheret 2009) is well established. Two additional components are also involved in the regulation of AGO1, namely AGO1-mediated post-transcriptional stabilization of miR168 and the co-regulated expression of AGO1 and MIR168 genes (Vaucheret et al. 2006). Thus, the homeostasis of AGO1 and miR168 is maintained by a dedicated feedback regulatory loop.
Several recent investigations provide evidence that the expression of AGO1 and miR168 under stress conditions is controlled by multiple regulatory mechanisms. ABA-related transcriptional activation of MIR168a has been shown to be common to a variety of different plant abiotic stress responses, and the impact on AGO1 accumulation has been demonstrated to be partly eliminated by the transcriptional activation of the AGO1 gene (W. Li et al. 2012).
In regards to biotic stresses, most investigations have focused on plant–virus interactions. Various viruses induce miR168 accumulation in their hosts by encoding RNA-silencing suppressors in order to repress AGO1 expression, resulting in the inhibition of host miRNA-mediated antiviral responses (Burgyán 2008, Várallyay et al. 2010, Pumplin and Voinnet, 2013). Recent research has indicated that fungal elicitors can also activate MIR168 expression and a concomitant repression of AGO1 (Baldrich et al. 2014). Despite this report, the regulatory mechanism associated with miR168 and its target AGO1 in response to fungal pathogens is not well understood.
Malus hupehensis (Pamp.) Rehd. is an important Malus germplasm endemic to China, and is widely used as apple rootstock in China. Botryosphaeria dothidea is one of the most economically important fungal pathogens of Malus (Smith et al. 1997). In this study, the role of miR168 and its target MhAGO1 in M. hupehensis responses to B. dothidea infection was investigated. The results indicate that both MIR168a and MhAGO1 are transcriptionally activated by B. dothidea, while MhAGO1 is partly repressed through miR168-mediated post-transcriptional regulation. Several pathogen-responsive cis-elements are present in the MIR168a promoter and respond to B. dothidea inoculation and salicylic acid (SA) treatment. Plants that transiently overexpress miR168a or silence AGO1 are less sensitive to B. dothidea infection, as evidenced by a delay in symptom development and an inhibition in pathogen growth, while results of the miR168 interfering plants turns out the opposite. The observed enhancement in disease resistance may be the result of alterations in reactive oxygen species (ROS) production, basal resistance and SA-mediated defense.
Results
Accumulation of miR168 transcripts is induced by B. dothidea infection
miRNA168 has been implicated as an important regulator in various plant–virus interactions (Csorba et al. 2007, Várallyay et al. 2010, Várallyay et al. 2014). Recent studies have also reported the induced expression of mature miR168 during the response to several fungal pathogens (Baldrich et al. 2014, Shen et al. 2014). Our previous high-throughout sequencing analysis indicated that expression of M. hupehensis miR168 was induced in response to B. dothidea infection (Yu et al. 2014). In the present study, the expression pattern of miR168 after inoculation of plants with B. dothidea was analyzed in order to substantiate further the responsiveness of miR168 to infection. Rooted 6-week-old M. hupehensis tissue culture seedlings were inoculated with a suspension of B. dothidea conidia. Seedlings inoculated with water were used as a control. Stem–loop real-time quantitative PCR (RT-qPCR) was used to detect the abundance of mature miR168 at 0, 6, 12, 24, 48, 72 and 96 hours post-inoculation (hpi). The results indicated that a distinct up-regulation of miR168 expression occurred at 6 hpi. Relative to the control, mature miR168 in infected plants continued to accumulate over a period of 24 hpi, and maintained the elevated levels (about 5-fold higher than in control plants) until the last detection at 96 hpi (Fig. 1A). The evidence of pathogen infection was shown by the calculated B. dothidea colonization coefficient, which indicates the relative biomass of the pathogen and host (Fig. 1C). The RT-qPCR data confirmed the results obtained by high-throughout sequencing, and provided further evidence that miR168 is induced by B. dothidea infection.

Expression analysis of miR168 and pre-miR168a during the response to B. dothidea infection. (A) Stem–loop RT-qPCR analysis of mature miR168. Amplification was normalized to 5.8 s rRNA transcripts. (B) RT-qPCR analysis of pre-miR168a. The expression of EF-1α was used as the internal control for normalization. The data presented represent the relative expression level compared with the corresponding mock-treated controls at each time point. RT-qPCR analysis included three technical replicates of each biological replicate. Significant differences between each of the time points and 0 hpi are indicated by asterisks (*P ≤ 0.05; **P ≤ 0.01). (C) Quantification of B. dothidea growth on infected M. hupehensis leaves. The colonization coefficient was calculated by the relative biomass of the pathogen and host, with a correction (see the Materials and Methods). The data represent the mean ± SD of three biological replicates (n = 3).
According to information in the miRbase 21 database, mdm-miR168a and mdm-miR168b, two members of the M. domestica miR168 family, share the same mature miRNA sequence. Gene-specific reverse primers were used to isolate the precursor of miR168a and b from total RNA. A 124 bp precursor of miR168a was obtained, and the hairpin structure is illustrated in Supplementary Fig. S1. Nevertheless, we have failed to obtain the pre-miR168b from M. hupehensis. Considering that Arabidopsis MIR168b expression is restricted to the shoot apical meristem while MIR168a is widely co-expressed with AGO1 (Vaucheret 2009), we suspect that members of the Malus miR168 family follow a similar pattern. Further investigations in the present study focused on miR168a. A 583 bp fragment containing the precursor of miR168a was isolated from M. hupehensis genomic DNA and sequenced. The transcript levels of pre-miR168a were monitored by RT-qPCR in order to investigate how this specific family member responds to B. dothidea infection. The results indicated that the accumulation of pre-miR168a rapidly reaches a peak at 24 hpi, and then gradually diminishes (Fig. 1B). Based upon these data, it is reasonable to infer that the transcription of miR168a was induced by B. dothidea infection.
Transcriptional activation of eukaryotic genes by RNA polymerase II requires cis-acting regulatory elements to interact with trans-acting factors (Kadonag 2004). Just as in protein-coding genes, cis-acting elements of MIR genes are located in their upstream regulatory regions (Xie et al. 2005). Therefore, the promoter region of MIR168a was scanned for the presence of potential cis-acting elements in order to obtain further insight into the transcriptional regulation of MIR168a in response to B. dothidea infection. A 3 kb fragment upstream of the precursor structure of miR168a was downloaded from the National Center for Biotechnology Information (NCBI) database. Given that the M. hupehensis genome may be slightly different from the sequenced Golden Delicious whole genome, a 2 kb fragment upstream of the transcription start site (TSS) was isolated from M. hupehensis genomic DNA using a set of nested primers. The obtained sequence was scanned to identify the presence of known stress- and hormone-related cis-acting elements.
The results indicated that cis-elements which were correlated with response to pathogens, elicitors and SA are in abundance in the MIR168a promoter (Fig. 2A; Supplementary Table S1). Several typical W-boxes, including W-BOX NPR1 (TTGAC) and WRKY 710 S (TGAC), were identified. W-boxes are specifically recognized by SA-responsive WRKY transcription factors and are found in the promoters of genes involved in the SA signaling pathway, such as pathogenesis-related (PR) genes (Eulgem and Somssich 2007). ASF MOTIF CAMV (TGACG) and GT1 CONSENSUS (GRWAAW) are two additional SA-related cis-elements present in the promoter of MIR168a. ASF MOTIF CAMV is the binding site of ASF-1 and TGA family transcription factors, both of which are involved in the transcriptional activation of several genes by SA, including NtPR1a (Grüner et al. 2003). GT1 CONSENSUS (GRWAAW), which binds GT-1-like factors to the tobacco PR-1 a promoter, influences the level of SA-inducible gene expression (Buchel et al. 1996). ELI-box3 (AAACCAATT), a conserved fungal-responsive element (Pastuglia et al. 1997), was also found in the MIR168a promoter. Additional identified pathogen-responsive elements included a CACGTG MOTIF, GT1GMSCAM4 (GAAAAA), a BOXLCOREDCPAL (ACCWWCC) and a BIHD10S (BIHD10S). A number of other cis-elements associated with the defense-related hormones, ethylene and methyl jasmonic acid, were also identified in the MIR168a promoter. Several regulatory elements related to plant growth, as well as abiotic stresses, are also present in the MIR168a promoter.
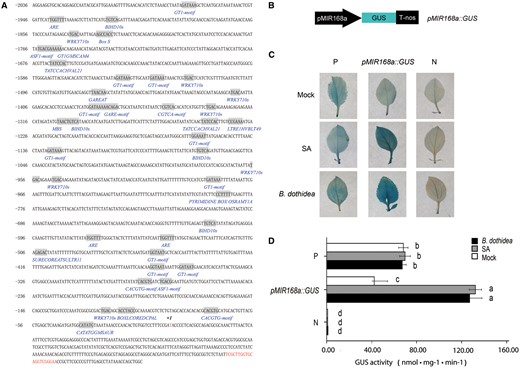
Structural and functional analyses of the MIR168a promoter. (A) Nucleotide sequence of the MIR168a promoter (sense strand). The TSS (+1) is indicated as +1. The sequences of identified cis-elements are shaded and the motif names are provided under the elements. The mature miRNA is shown in red (see Supplementary Table S1 for details). (B) Schematic diagram of the pMIR168a::GUS construct. (C and D) Histochemical staining pattern (C) and GUS activity (D) of M. hupehensis leaves in response to different treatments. The fully expanded leaves from 6-week-old tissue culture plants of M. hupehensis were infiltrated with Agrobacterium carrying different constructs. GUS staining was performed 3 d after B. dothidea inoculation, SA treatment or mock treatment with water. The presented images of leaves in (C) are representative of the results obtained from three independent experiments. Data shown in (D) represent the mean ± SD of three independent experiments (n = 3). Different letters denote significant differences between the constructs and the treatents (P ≤ 0.05). P, positive control (pBI121); N, negative control (pBI101).
A further analysis of the MIR168a promoter was conducted in order to confirm the transcriptional induction of miR168a in response to B. dothidea infection. In order to examine gene expression patterns, the MIR168a promoter region fragment was fused to the β-glucuronidase (GUS) gene, replacing the 35SCaMV (Caulifower mosaic virus) promoter of the binary vector pBI121. The obtained construct, pMIR168a::GUS (Fig. 2B), was used to transform M. hupehensis leaves transiently. A 35SCaMV::GUS (pBI121) construct served as the positive control, with a construct (pBI101) devoid of a promoter serving as the negative control. Transiently transformed leaves of M. hupehensis were inoculated with B. dothidea, with leaves infiltrated with a suspension buffer serving as a control. Considering the presence of the SA-related cis-elements in the MIR168a promoter, an additional treatment with 0.1 mM SA was also included. The resulting histochemical staining pattern revealed the effect of the various treatments on the level of GUS expression in transiently transformed leaves (Fig. 2C, D). As expected, the GUS gene driven by the 35SCaMV promoter was constitutively expressed in the transformed leaves. A low level of GUS expression driven by the MIR168a promoter was observed in untreated transformed leaves. GUS expression, however, increased markedly when leaves were either inoculated with B. dothidea or after receiving an SA treatment. In fact, the level of expression was even much greater than in the positive control. These results confirmed that the MIR168a promoter was activated in the pMIR168a::GUS construct when leaves were exposed to SA or B. dothidea, even though a relatively low level of GUS expression was also detected in the negative control (Fig. 2C, D). The data from the precursor and mature miR168 expression analysis, along with the promoter activity assay, demonstrated that the induced expression of mature miR168 transcripts in response to B. dothidea is at least partially due to the activation of the MIR168a promoter.
The induced expression of miR168 was also observed in Arabidopsis and Brassica napus that were inoculated with fungal pathogens (Baldrich et al. 2014, Shen et al. 2014). To understand whether the transcriptional activation of MIR168 is universal or specific in M. hupehensis, we scanned promoter regions of MIR168 in 20 dicot and monocot species. Five pathogen-responsive cis-elements (ASF1 MOTIF CAMV, CACGTG MOTIF, W-BOX, GT1GMSCAM4 and BIHD1OS), which were found in the MIR168 promoter, were identified in the promoter region of these MIR168 homologs; among which, W-BOX and GT1GMSCAM4 displayed a high occurrence (Supplementary Table S2). This observation suggests that, in diverse species of land plant, the pathogen-regulated MIR168 expression may be conserved, and share a similar regulatory mechanism.
MhAGO1 is the target of miR168
miRNAs participate in various biological pathways via silencing of gene expression in a targeted manner (Jones-Rhoades et al. 2006). Therefore, the identification of the miR168 target is essential to understanding its role in the response to B. dothidea. Thus, apple coding DNA sequence (CDs) was downloaded from the Genome Database for Rosaceae (GDR) (www.rosaceae.org) and examined using psRNATarget (Dai and Zhao. 2011) to identify the potential targets of miR168. The analysis indicated that MDP0000161046 is a candidate target of miR168. A 4,126 bp cDNA fragment containing a 3,375 bp open reading frame (ORF) was amplified from M. hupehensis using a pair of specific primers, cloned and subsequently sequenced. The complete ORF revealed a deduced protein sequence containing 1,124 amino acids. The Piwi and PAZ domains showed that this is a potential Argonaute gene (Supplementary Fig. S2). Homology analysis of the Arabidopsis ARGONAUTE protein family and the identified amino acid sequence indicated that the potential M. hupehensis Argonuaute protein was highly homologous to Arabidopsis AGO1 (Supplementary Fig. S3A). Therefore, the potential target was designated as MhAGO1. This designation is consistent with the common belief that the AGO1 gene is the conserved target of miR168 in plants (Vaucheret 2006). In order to verify the accuracy of the computational prediction, a 5′ RNA ligase-mediated rapid amplification of cDNA ends (RLM-RACE) analysis was conducted. The results confirmed that MhAGO1 is indeed the target of miR168. Identification of the accurate cleavage site indicates that the cleavage occurs between the 10th and 11th nucleotide of the target site (Fig. 3A). Additional AGO1 proteins from other plant species were downloaded from the NCBI database followed by a BLASTP analysis. Multiple sequence alignment and a phylogenetic analysis indicated a high degree of evolutionary conservation of the AGO1 gene among species that are members of the Rosaceae family (Supplementary Fig. S3B).
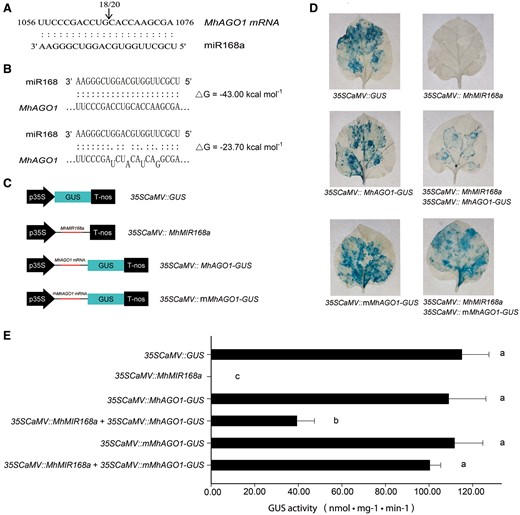
Identification and confirmation of MhAGO1 as a target of miR168. (A) Identification of the predicted miR168 target site as determined with 5′ RLM-RACE. The position of 5′ RLM-RACE products is indicated with an arrow. The numbers above the sequence indicate the detected cleavage site in independent clones. (B) Nucleotide sequences of two versions of AGO1 with different sensitivity to miR168: MhAGO1 (sensitive) and mMhAGO1 (insensitive). (C) Schematic diagrams of constructs used in co-transformation. (D and E) Histochemical assay (D) and GUS activity (E) of tobacco leaves infiltrated with different constructs. The different constructs were agroinfiltrated into fully expanded leaves of 6-week-old tobacco plants. GUS staining was performed 3 d after the injection. The presented images of leaves in (D) are representative of the results obtained from three independent experiments. Data presented in (E) represent the mean ± SD of three independent experiments (n = 3). Different letters denote significant differences between the constructs (P ≤ 0.05).
Taking advantage of the co-transformation technique in tobacco, the interaction between miR168 and MhAGO1 was further examined in vivo. The DNA fragment containing the miR168a precursor was cloned into pBI121 to make a 35SCaMV::MIR168a construct. The wild-type and mutated MhAGO1 fragment was fused with GUS to obtain 35SCaMV::MhAGO1-GUS and 35SCaMV::mMhAGO1-GUS constructs, respectively. The mutated MhAGO1 has synonymous mutations in the target site that disrupt the sensitivity to miR168, while the amino acid sequence is unchanged. A 35SCaMV::GUS (pBI121) construct was used as a positive control (Fig. 3B, C). GUS expression was equally high in leaves infiltrated with 35SCaMV::GUS, 35SCaMV::MhAGO1-GUS and 35SCaMV::mMhAGO1-GUS. In contrast, GUS expression could not be detected in 35SCaMV::MIR168a-transformed leaves. GUS expression was significantly lower in leaves that were simultaneously infiltrated with both 35SCaMV::MhAGO1-GUS and 35SCaMV::MIR168a, yet co-expression of MIR168a and mMhAGO1-GUS did not detectably affect the expression of GUS (Fig. 3D). These observations indicated that GUS expression in 35SCaMV::MhAGO1-GUS leaves was inhibited by exogenous MIR168a via complementary base pairing. GUS expression analyses were in good agreement with the histochemical observations (Fig. 3E).
Collectively, the results obtained from the computational prediction and the 5′ RLM-RACE, in combination with results of the tobacco co-transformation, indicate that MhAGO1 is the direct target of miR168, and is silenced by mRNA cleavage.
Transcriptional activation and miR168-mediated post-transcriptional regulation of MhAGO1 in response to B. dothidea infection
To explore further the effect of induced accumulation of miR168 on its MhAGO1 target during B. dothidea infection, Northern blot analysis was conducted to measure the expression of the mature miR168 and the MhAGO1 mRNA in M. hupehensis seedlings inoculated with B. dothidea for 0, 6, 12, 24, 48, 72 and 96 h. A probe located upstream of the target site was designed to detect both uncleaved mRNA and 5′ cleavage products (Fig. 4A). Unexpectedly, the results indicated that the abundance of uncleaved MhAGO1 mRNA increased in response to B. dothidea infection, sharing a similar tendency with the mature miR168. The accumulation of potential 5′ cleavage products also increased during the infection (Fig. 4A). For evidence of pathogen infection, please see the calculated B. dothidea colonization coefficients (relative biomass of the pathogen and host quantified by RT-qPCR at different times post-inoculation presented in Fig. 1C.
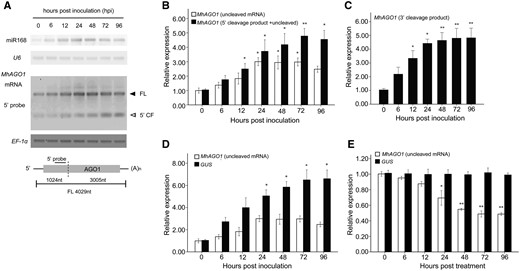
Expression analysis of MhAGO1 in response to B. dothidea infection. (A) Northern blot analysis of mature miR168 and MhAGO1 mRNA. LMW RNA was probed with DIG-labeled oligonucleotides complementary to miR168 and U6, while the total RNA was hybridized to the probe as indicated in the schematic of MhAGO1. FL, full length; 5′ CF, 5′ cleavage fragment. The experiment was repeated three times, and similar results were observed. (B) RT-qPCR analysis of uncleaved MhAGO1 mRNA and 5′ cleavage products. The white column indicates the accumulation of MhAGO1 uncleaved mRNA, which was amplified using a primer pair flanking the cleavage site. The black column represents the data obtained using a primer pair located upstream of the target site, which provided both uncleaved mRNA and 5′ cleavage products. (C) RT-qPCR analysis of the accumulation of 3′ cleavage products of MhAGO1. The total mRNA exacted from inoculated or mock-treated M. hupehensis leaves was ligated with the adaptor used in the 5′ RLM-RACE and subsequently reverse transcribed. A sense primer located in the adaptor region and a gene-specific antisense primer were used to amplify the 3′ cleavage products. (D and E) RT-qPCR analysis of GUS and AGO1 mRNA abundance in leaves transiently transformed with a pMhAGO1::GUS construct and treated with B. dothidea (D) and SA (E). EF-1α transcripts were used to normalize all expression values. The data represent the relative expression level compared with the corresponding mock-treated controls at each time point. Data represent the mean ± SD of three biological replicates (n = 3). RT-qPCR analysis included three technical replicates of each biological replicate. Significant differences between time points are indicated with asterisks in (B) and (C). Asterisks in (D) and (E) indicate significant differences between GUS and MhAGO1 (*P ≤ 0.05; **P ≤ 0.01).
RT-qPCR analysis was also conducted to quantify the MhAGO1 expression. Two pairs of gene-specific primers were initially designed. One primer pair, spanning the target site of miR168, was used to detect the uncleaved MhAGO1 mRNA. The other primer pair, located upstream of the target site, was used to detect both uncleaved mRNA and 5′ cleavage products (Fig. 4B). At 24 hpi, the abundance of uncleaved MhAGO1 mRNA was approximately 3-fold higher than in the mock-treated samples. The rate of increase and overall level of accumulation of the potential 5′ cleavage products was slightly higher than it was for the uncleaved mRNA (Fig. 4B).
Subsequently, the abundance of 3′ cleavage products was examined by RT-qPCR using the adaptor that had been used in the 5′ RLM-RACE. The sense primer was contained within the adaptor, and a gene-specific antisense primer was located downstream of the cleavage site. The RT-qPCR data revealed a rapid increase in the accumulation of 3′ cleavage products from 6 to 24 hpi, after which the levels remained at a high level (Fig. 4C). The observed differential abundance of the two cleavage products may be the result of the higher instability of the 5′ cleavage product (Chen 2008).
The accumulation of endogenous MhAGO1 and exogenous GUS transcripts driven by the AGO1 promoter responding to B. dothidea infection was monitored in order to elucidate further how the expression of MhAGO1 is regulated. A 2 kb DNA fragment upstream of the MhAGO1 TSS was cloned and inserted into pBI121, replacing the 35SCaMV promoter. The resulting pMhAGO1::GUS construct was then transiently expressed in M. hupehensis leaves using Agrobacterium-mediated transformation. In the absence of miR168-mediated cleavage, the increase in the abundance of GUS mRNA in transformed leaves was much higher than MhAGO1 transcripts after B. dothidea inoculation (Fig. 4D). These results suggest that MhAGO1 is transcriptionally activated in response to B. dothidea infection, and the induction is partly inhibited by miR168 at the post-transcriptional level.
To investigate whether MhAGO1 is regulated by a similar mechanism as for miR168 during the infection, we screened its promoter and further examined the endogenous MhAGO1- and pMhAGO1-driven GUS transcripts by RT-qPCR under SA treatment. Despite the identification of five tentative cis-elements that are related to the pathogen and SA (Supplementary Table S3), GUS expression was not observed to be up-regulated by SA treatment (Fig. 4E). Meanwhile, the endogenous MhAGO1 was probably decreased by the post-transcriptional regulation of induced miR168 (Fig. 4E).
MIR168a positively regulates the resistance of M. hupehensis to B. dothidea
Given the induced miR168 accumulation during the infection, we speculated that miR168 and AGO1 may play a part in the resistance of M. hupehensis to B. dothidea. To clarify this point, the resistance of transiently transformed M. hupehensis leaves was assessed. Leaves transiently overexpressing miR168 were obtained by agroinfiltration with the 35SCaMV::MIR168a construct. A construct containing a short tandem target mimic (35SCaMV::STTM168) was also used to generate leaves with reduced endogenous miR168 activity (STTM168). Leaves infiltrated with 35SCaMV::GUS (Vector) and non-infiltrated leaves (wild type) were used as controls. Infiltrated leaves were maintained at a high relative humidity in dark conditions for a period of 2 d.
To examine the transformation efficiency, Northern blot analysis was conducted to determine the expression levels of miR168 and MhAGO1. The expression of mature miR168 and its precursor from leaves infiltrated with 35SCaMV::MIR168a was observably higher than in the two controls (Fig. 5A), while mature miR168 was almost undetectable in the STTM168 leaves (Fig. 5B). The abundance of uncleaved MhAGO1 mRNA showed modest regulation in the corresponding leaves (Fig. 5A, B), indicating that the transient transformation can effectively regulate MhAGO1 expression. Thus, the transformation efficiency was enough to proceed with further analysis.
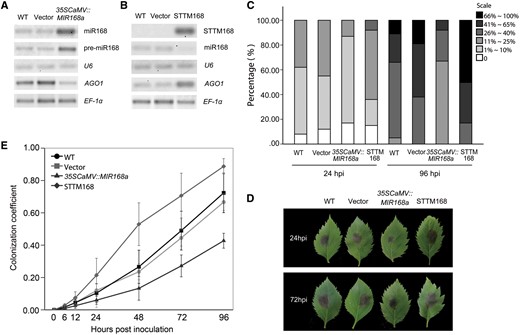
The B. dothidea resistance of MIR168a-overexpressing or STTM168 M. hupehensis leaves. (A) Northern blot analysis of miR168, pre-miR168a and full-length MhAGO1 mRNA in 35SCaMV::MIR168a M. hupehensis leaves. (B) Northern blot analysis to detect STTM168, miR168 and full-length MhAGO1 mRNA in STTM168 leaves. U6 and EF-1α served as internal controls. Ten random leaves were pooled in each experiment and used as a biological replicate. (C) Percentage of infected leaves at 24 and 96 hpi in different disease level categories. Non-infiltrated (WT) leaves and leaves infiltrated with empty vector (Vector), 35SCaMV::MIR168a and 35SCaMV::STTM168 (STTM168) were placed in the dark for 2 d and inoculated with a suspension of B. dothidea conidia (6 × 106 conidia ml–1). Disease progress was divided into six categories based on the area of the necrotic lesion relative to total leaf area. Level 1, <1%; level 2, 1–10%; level 3, 11–25%; level 4, 26–40%; level 5, 41–65%; and level 6, >66%. The data represent the average of three independent experiments using a total of 320 infected leaves. (D) Representative symptoms in M. hupehensis leaves infected with B. dothidea. Pictures were photographed at 24 and 72 hpi after the 2 d dark incubation. (E) Quantification of B. dothidea growth on infected M. hupehensis leaves non-infiltrated or infiltrated with different constructs. The colonization coefficient was calculated with a correction (see the Materials and Methods). The data represent the mean ± SD of three biological replicates (n = 3).
The transiently transformed leaves and two controls were then challenged with B. dothidea, and disease severity was evaluated at 24 and 96 hpi. The disease progress in the infected leaves was divided into six levels based on the size of the necrotic lesion. The occurrence of leaves with a low level of disease progress was much higher at both 24 and 96 hpi in 35SCaMV::MIR168a leaves as compared with the two controls (Fig. 5C), while the STTM168 leaves exhibited the most severe disease. At 24 hpi, the percentage of leaves at level 2 represented the majority of infected 35SCaMV::MIR168a leaves, while the percentage of leaves at level 3 was greater in the two controls than it was for the 35SCaMV::MIR168a leaves. Notably, whereas a percentage of leaves in the two controls were classified in level 6 at 96 hpi, the highest level of disease progress in 35SCaMV::MIR168a leaves was at level 5 (Fig. 5C). Meanwhile, level 6 occupied the biggest proportion of the STTM168 leaves at that time point (Fig. 5C). Unexpectedly, the disease severity in leaves infiltrated with the 35SCaMV::GUS construct was slightly greater than in the non-infiltrated leaves. Typical symptoms of infiltrated and non-infiltrated leaves are shown in Fig. 5D. Thus, in regards to the observed symptoms, miR168 plays a crucial role in the susceptivity to B. dothidea.
Next, the growth of B. dothidea on infected M. hupehensis leaves was determined by calculating a colonization coefficient based on the relative biomass of the pathogen and host combined with RT-qPCR analysis. An analysis of the dissociation curve for primer specificity indicated the specific amplification of the target DNAs in both the plant and the pathogen (Supplementary Fig. S4A). Standard curves for calculating the biomass of the host and the pathogen are presented in Supplementary Fig. S4B and C, respectively. No significant difference in the detected biomass in leaf or pathogen was observed due to the different infiltrated constructs and hours of post-leaf detachment (Supplementary Fig. S4D). The calculated colonization coefficient in the challenged leaves revealed no statistical difference until 12 hpi. The colonization coefficient in miR168a-overexpressing leaves and the two controls increased differentially and the divergence between them became statistically significant at 24 hpi and the subsequent time points (Fig. 5E). The colonization coefficient clearly demonstrated that the B. dothidea growth rate was much higher in the two controls than it was in the miR168a-overexpressing leaves. As expected, the STTM168 leaves had a significantly high colonization coefficient compared with the empty vector and wild-type controls (Fig. 5E) after B. dothidea inoculation.
Collectively, the observation of disease symptoms and the calculation of the pathogen colonization coefficient implied miR168 as a positive regulator in the resistance of M. hupehensis to B. dothidea
Negative regulation of miR168, an oxidative burst and cell death in response to B. dothidea infection
PTI is the first line of plant defense against pathogens and is triggered by PAMPs (Dangl and Jones 2001). miRNA-mediated post-transcriptional regulation is thought to play an essential role in several classical PTI responses, including an oxidate burst of ROS. To determine whether or not the enhanced B. dothidea resistance observed in the present study in leaves transiently overexpressing miR168a is associated with an oxidative burst of ROS, changes in H2O2 generation were used as an indicator to compare the ROS burst in 35SCaMV::MIR168a, STTM168 and the two controls. Significant increases in the accumulation of H2O2 were detected in the two controls, while this increase was diminished in 35SCaMV::MIR168a leaves. In contrast, a dramatic accumulation of H2O2 was observed in STTM168 leaves during the infection (Fig. 6A).
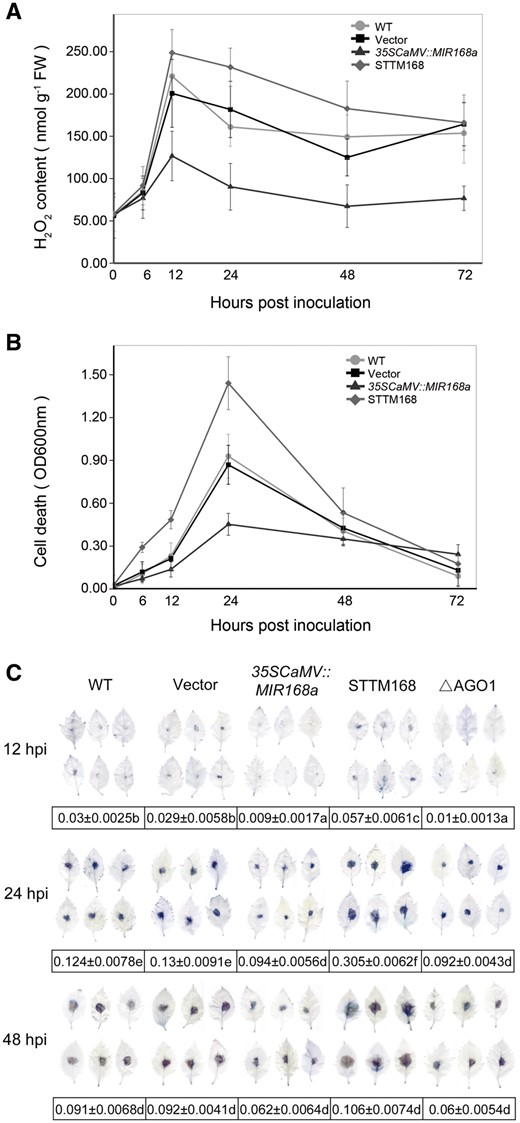
ROS production and cell death of 35SCaMV::MIR168a or STTM168 leaves in response to B. dothidea infection. (A) Changes in the level of H2O2 generation in leaves infiltrated with 35SCaMV::MIR168a or STTM168 and non-infiltrated (WT) and empty vector control (Vector) at different time points (hpi) after B. dothidea inoculation. (B and C) Level of cell death, as determined by Evans blue staining, in leaves infiltrated with the indicated constructs and in non-infiltrated leaves after B. dothidea inoculation. The amount of dye present in dead cells was determined by extracting the dye and measuring absorbance at a wavelength of 600 nm (B). Stained and decolorized leaves were selected at random and scanned (C). Data presented in (C) are the pixel number ratios of stained areas and the total leaf areas measured by the Adobe Photoshop histogram tool. Different letters denote significant differences between the constructs and the time points (P ≤ 0.05). The data represent the mean ± SD of three biological replicates (n = 3). Fifteen leaves of each biological replicate were examined for each constructs at each time point.
ROS-triggered cell death was also monitored. An Evans blue staining assay was used to indicate cell death. Evans blue staining was barely detectable in all leaves with the mock treatment. In contrast, an increasing level of Evans blue staining was observed in leaves of the two controls from 12 to 24 hpi, indicating an increase in the level of cell death (Fig. 6B). Evans blue staining decreased after 24 hpi and was close to zero at 72 hpi. As the dye is not retained in disrupted cells (Baker and Mock 1994), the reduction in staining was most probably due to the collapse of the dead cells. The cell death rate was lower in 35SCaMV::MIR168a leaves than it was in the two controls and gradually declined after 24 h, indicating that fewer cells were killed, as evidenced by the different pattern of Evans blue staining relative to the two controls. STTM168 leaves had a significantly higher rate of cell death than the two controls, and the retained dye level dropped rapidly after 24 hpi (Fig. 6B). Consistent results were obtained by scanning and image analysis of stained leaves (Fig. 6C).
The combined result of the Evans blue staining assay and the evaluation of H2O2 generation support the premise that miR168 is a negative regulator of the ROS burst and cell death that typically occurs in response to B. dothidea infection.
miR168a activated the basal resistance and SA-mediated defense of M. hupehensis
In order to determine the involvement of SA-mediated basal defense in the altered resistance of 35SCaMV::MIR168a and STTM168 M. hupehensis, the expression of four PR genes belonging to different families of PR proteins, and PAL, the key regulator of the SA biosynthesis pathway, were asluated. A significant increase in the constitutive expression R genes and PAL was observed in leaves transiently overexpressing miR168a, relative to the levels observed in leaves from the two controls, while STTM168 leaves had a significantly reduced transcripts level of these genes (Fig. 7A). The abundance of PR1, an SA defense marker gene (Uknes et al. 1992), was then evaluated in response to an SA treatment and B. dothidea infection. An increase in PR1 abundance was observed in the 35SCaMV::MIR168a and TTM168 leaves, as well as in the two controls, in response to both the SA treatment (Fig. 7B) and B. dothidea infection (Fig. 7C). A more sensitive induction of PR1 was observed in 35SCaMV::MIR168a leaves after SA treatment, relative to that in the two controls (Fig. 7B), though the difference in the increased rate was not statistically significant (Fig. 7B). In contrast, the level of PR1 in leaves transiently overexpressing miR168a in response to B. dothidea infection was dramatically higher as compared with the two controls (Fig. 7C). Meanwhile, STTM168 leaves showed modest PR1 accumulations after both the SA (Fig. 7B) and B. dothidea treatment (Fig. 7C).
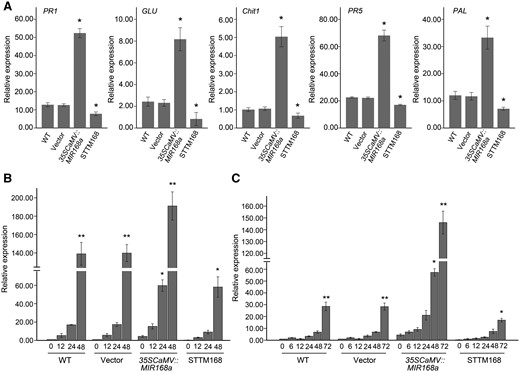
SA-mediated basal defense responses are altered by miR168a. (A) Constitutive expression of PR genes and PAL in 35SCaMV::MIR168a or STTM168 leaves and in leaves of non-infiltrated (WT) and empty vector control (Vector). Data were normalized to EF-1α. (B and C) Accumulation of PR1 at different time points after SA treatment (B) or B. dothidea inoculation (C) in infiltrated and non-infiltrated leaves. The expression level in non-infiltrated leaves in response to mock inoculation was set at a value of 1.0. EF-1α was used as the internal control. Asterisks in (A) indicate significant differences in expression relative to the expression in non-infiltrated leaves. Significant differences between the constitutive expression levels of the PR1 gene and that at different time points in the different treatments are indicated by an asterisk in (B) and (C) (*P ≤ 0.05; **P ≤ 0.01). Data represent the mean ± SD of three biological replicates (n = 3).
MhAGO1 is a negative regulator of the B. dothidea resistance
In our experiments, the induced miR168 accumulation, in parallel with the increasing cleavage of the target, was accompanied by a concomitant transcriptional activation of MhAGO1 in response to the B. dothidea infection. To address the exact role of MhAGO1 in response to B. dothidea, an RNAi construct was used to generate leaves transiently silenced of MhAGO1 (ΔAGO1). Northern blot analysis confirmed that the abundance of MhAGO1 transcripts was notably reduced in the transiently transformed leaves (Fig. 8A). The MhAGO1 silencing was confirmed to be caused by the RNAi pathway, through the accumulation of low molecular weight (LMW) RNA with the RNAi construct sequence (Fig. 8A).
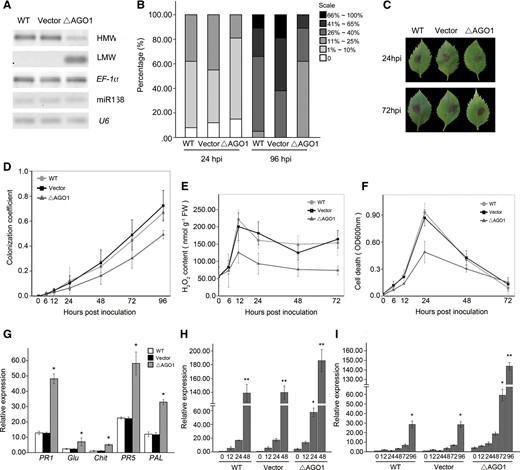
Effects of MhAGO1 silencing on B. dothidea resistance, ROS production, cell death and SA-mediated basal defense responses. (A) Northern blot analysis of MhAGO1 for high molecular weight (HMW) and low molecular weight (LMW) RNAs, and miR168 in MhAGO1 silenced leaves. U6 and EF-1α served as internal controls. Ten random leaves were pooled in each experiment and used as a biological replicate. (B) Percentage of infected leaves at 24 and 96 hpi in different disease level categories. (C) Representative symptoms in MhAGO1 silenced M. hupehensis leaves infected with B. dothidea. (D) Quantification of B. dothidea growth on infected leaves infiltrated with the MhAGO1 RNAi construct. (E) Changes in the level of H2O2 generation in MhAGO1 silenced leaves at different time points (hpi) after B. dothidea inoculation. (F) Level of cell death in MhAGO1 silenced leaves after B. dothidea inoculation. (G) Constitutive expression of PR genes and PAL in MhAGO1 silenced leaves. (H and I) Accumulation of PR1 at different time points after SA treatment (H) or B. dothidea inoculation (I) in MhAGO1 silenced leaves. For (G), (H) and (I), EF-1α was used as the internal control. Asterisks in (G) indicate significant differences in expression relative to the expression in non-infiltrated leaves. Significant differences between the constitutive expression levels of the PR1 gene and that at different time points in the different treatments are indicated by an asterisk in (H) and (I) (*P ≤ 0.05; **P ≤ 0.01). All data represent the mean ± SD of three biological replicates (n = 3). WT, non-infiltrated control; Vector, empty vector control.
Similar to 35SCaMV::MIR168a leaves, when inoculated with B. dothidea, the ΔAGO1 leaves showed delayed symptom development (Fig. 8B, C) and alower calculated colonization coefficient compared with the two controls (Fig. 8D). The accumulation of H2O2 and ROS-triggered cell death were detected for ΔAGO1 leaves during the B. dothidea infection. As expected, the increase of H2O2 abundance in the ΔAGO1 leaves was significantly weaker than in the empty vector and the wild-type control (Fig. 8E). Consistent with the H2O2 accumulation, the cell death rate was relatively lower in ΔAGO1 leaves compared with the two controls (Figs. 8, 6C). The constitutive expression of the four PR genes and PAL gene was also measured in ΔAGO1 leaves. All the five detected genes were significantly up-regulated in ΔAGO1 leaves compared with the two controls (Fig. 8G). We next investigated the PR1 expression after B. dothidea inoculation and SA treatment in ΔAGO1 leaves. The results indicated that ΔAGO1 leaves had higher PR1 accumulations than the two controls during both treatments (Fig. 8H, I).
Discussion
The involvement of miR168-mediated regulation of AGO1 in various plant–virus interactions is well recognized (Havelda et al. 2008, Várallyay et al. 2010, Várallyay and Havelda 2013, Várallyay et al. 2014). Recent reports focusing on fungal-responsive miRNAs have also demonstrated the involvement of miR168 and AGO1 in the response of plants to fungal pathogens (Weiberg et al. 2013, Shen et al. 2014, Baldrich et al. 2014). In the present study, evidence is presented supporting the premise that miR168 interfering with MhAGO1 participates in the defense response of M. hupehensis against B. dothidea.
Monitoring the abundance of miR168 and its precursor pre-miR168a during the response of M. hupehensis leaves to B. dothidea infection revealed an increase in miR168 accumulation accompanied by the up-regulation of pre-miR168a, indicating that mature miR168 is induced as a response to B. dothidea infection. Mature miR168 plays a regulatory role by regulating its exclusive target AGO1 (Vaucheret 2006). In our study, we identified a putative ARGONAUTE gene MhAGO1, with high homology to AtAGO1, as the potential target of M. hupehensis miR168. The cleavage of MhAGO1 by miRNA168 was confirmed by 5′ RLM-RACE and a tobacco co-transformation assay.
AGO1 expression is post-transcriptionally regulated by miR168 and the siRNAs generated from its cleavage products (Mallory and Vaucheret 2009). A complex feedback regulatory loop, involving the co-expression and interaction of MIR168 and AGO1, maintains the homeostasis of both AGO1 and miR168 (Vaucheret et al. 2006, Vaucheret, 2009, Várallyay et al. 2010, W. Li et al. 2012). Our data revealed that miR168 and MhAGO1 accumulation in response to B. dothidea infection was regulated at several levels. As previously mentioned, an up-regulation of pre-miR168a was observed in response to B. dothidea infection, demonstrating that the increase in miR168 accumulation is at least partly due to the transcriptional activation of MIR168a. The observed activation of the MIR168a promoter in response to inoculation, combined with the diverse identified pathogen-responsive cis-acting elements, provides further evidence that MIR168a is involved in mediating the response of M. hupehensis to B. dothidea. Our results are consistent with a recent report indicating that Arabidopsis MIR168a is transcriptionally activated by fungal elicitors (Baldrich et al. 2014). In addition, a couple of cis-acting elements related to pathogens are found within the promoter of MIR168 homologs in several plant species, suggesting a common transcriptional regulation mechanism of MIR168 in responding to pathogen infection. Furthermore, the noted SA-activated Malus MIR168a promoter in this study, along with the ABA-responsive transcription factor-activated Arabidopsis MIR168a promoter reported by W. Li et al. (2012), indicates that plant hormone signaling pathways may also play an important role in the transcriptional regulation of MIR168.
In sharp contrast to the rapid accumulation of the 5′ cleavage product, an increase in the abundance of MhAGO1 mRNA with a simultaneous up-regulation of miR168 expression was observed. Driven by the MhAGO1 promoter, the GUS reporter gene was transcriptionally activated in response to B. dothidea infection. The observed differences in the degree of activation of miR168-free GUS and endogenous MhAGO1 support the premise that MhAGO1 is induced at the transcriptional level and partly repressed at the post-transcriptional level in response to B. dothidea infection. Similar to the MIR168a promoter, the promoter of MhAGO1 was also found to contain several pathogen- and SA-related cis-elements. Nevertheless, the pMhAGO1-driven GUS was not observed to be up-regulated by SA treatment, which indicates that the up-regulation of MhAGO1 by B. dothidea infection may have a different mechanism from MIR168a.
A wealth of evidence has confirmed the critical regulatory role of miRNAs in various physiological processes (Chen 2009, Voinnet et al. 2009, Rubio-Somoza et al. 2011, Khraiwesh et al. 2012, Sunkar et al. 2012). AGO1, as an miRNA effector protein, is one of the critical components of the miRNA pathway (Baumberger and Baulcombe 2005). The fluctuation in AGO1 expression influences diverse plant–pathogen interactions (Morel et al. 2002, Ellendorff et al. 2009, Li et al. 2010, Várallyay et al. 2010). Therefore, the modulation of MhAGO1 expression by multiple mechanisms during the response to B. dothidea infection is not surprising. It is worth noting that fungal pathogens, such as Botrytis cinerea, can hijack the host RNAi machinery by generating small RNAs that bind to AGO1 and selectively silence host defense responses (Weiberg et al. 2013). We speculate that the induced expression of MhAGO1 observed in the present study may represent a virulence mechanism of B. dothidea. Therefore, the regulation of MhAGO1 at both the transcriptional and post-transcriptional levels may reflect a competition between the pathogen and the host.
Transient overexpression of miR168a in leaves results in enhanced resistance, and miR168 interference leads to a higher susceptibility. The finding clearly indicates that miR168a has a positive effect on the defense response of M. hupehensis against B. dothidea. Moreover, the results obtained on the quantification of pathogen growth are consistent with the observed necrotic lesion scale. Thus, the present study demonstrates that the increased miR168 expression inhibited both symptom development in the host tissues and the colonization and, hence, the growth of the pathogen.
Previous studies have demonstrated the involvement of miRNA-mediated post-transcriptional regulation in various PTI responses (Li et al. 2010, X. Zhang et al. 2011). Arabidopsis ago1 mutants exhibit several alterations in responses triggered by PAMPs (Li et al. 2010). We demonstrate that miR168a acts as a negative regulator of H2O2 accumulation and the resulting cell death triggered by the oxidative burst during the response to B. dothidea infection. In accordance with the previous, silencing of MhAGO1 in our study leads to a suppression of ROS production. Although hypersensitive cell death plays a key role in impeding the growth of invading pathogens, it can also facilitate infections by necrotrophic pathogens (Edlich et al. 1989, Govrin and Levine 2000). Since B. dothidea is considered as a necrotrophic pathogen (Phillips et al. 2013), it is reasonable to infer that the induction of miR168a might contribute to the inhibition of B. dothidea growth by repressing ROS production and cell death.
miR168 was also observed to activate basal resistance. In this regard, a series of Resistance (R) genes have been indentified as targets of miRNAs in several plant species (Shivaprasad et al. 2012, Boccara et al. 2014), including M. domestica (Ma et al. 2014). miRNA pathways have been reported to serve as important regulatory mechanisms of R gene expression (Zhai et al. 2011, F. Li et al. 2012). In view of the fact that altered expression of R genes often leads to a constitutive alteration of PR gene expression (Dangl and Jones 2001), the activation of PR genes observed in the present study is likely to be due to the inhibition of R gene-related miRNA pathways. Similar activation of PR genes has been reported in OsDCL1 RNAi lines (Zhang et al. 2015) and an Arabidopsis rdr6 loss-of function mutant (Boccara et al. 2014), providing further evidence for this hypothesis.
miR168a has positive roles in the accumulation of PR1 occuring in leaves in response to infection. It is interesting, however, that a similar difference was not observed in SA-treated leaves. Relating this result to the constitutive activation of PAL, we suggest that the more highly induced SA-mediated defense responses (i.e. the up-regulation of PR genes and PAL) may be the result of enhanced SA biosynthesis. Thus, the improved resistance observed in our study may be partly due to the activation of basal resistance and the SA-mediated defense response. MIR168a is transcriptionally activated by SA, indicating that this essential pathogen-responsive hormone may play multiple roles in miR168-mediated defense responses.
Given the pleiotropic character of AGO1 and its participation in miRNA pathways, miR168-mediated cleavage of MhAGO1 and the resulting alteration of multiple levels of defense responses are expected. The repression of MhAGO1 by overexpressing miR168 and the enhanced resistance were observed in the present study, and silencing of the gene displayed similar results. Yet these findings are not in accordance with the identification of single miRNA pathways that are involved in plant defense (Navarro et al. 2006, Li et al. 2010). The interaction between M. hupehensis and B. dothidea, however, is still not entirely elucidated. Regardless of the mechanism that is involved, we suggest that MhAGO1 negatively regulates B. dothidea resistance globally, while several MhAGO1-binding miRNAs may have positive effects.
In conclusion, the present study clearly demonstrates that the expression of miR168 and MhAGO1 in response to B. dothidea infection is regulated by a mechanism, and that miR168 is pivotal to the resistance of M. hupehensis to B. dothidea. Evidence is provided supporting the transcriptional activation of MIR168a expression in response to B. dothidea infection. The activation of the MIR168a promoter by both B. dothidea inoculation and SA treatment may result from its possessing several pathogen-responsive cis-acting elements. MhAGO1, the directed target of miR168, is transcriptionally activated by the infection, and partly repressed at the post-transcriptional level by the induction of miR168. Being a positive regulator of the B. dothidea resistance, miR168 not only delays disease symptom development but also impedes pathogen growth, and may play its role by altering diverse defense responses. MhAGO1, as a negative regulator, has opposing effects. The elucidation of the precise mechanism involved in the regulation of defense responses against B. dothidea by alterations in miR168/MhAGO1 expression still requires further research. Whether this particular miRNA pathway is limited to the M. hupehensis and B. dothidea interaction or is general to all Malus–pathogen interactions still remains an open question. The findings of the present study provide further evidence for the involvement of miR168 and AGO1 in plant–pathogen interactions, and highlight the significance of miRNA-mediated regulation in the defense response of Malus.
Materials and Methods
Plant growth conditions and pathogen cultures
Tissue-cultured plants of M. hupehensis (Pamp.) Rehd were subcultured in Murashige and Skoog (MS) medium with 6-benzylaminopurine (6-BA) (0.5 mg l–1) and 1-naphthaleneacetic acid (NAA) (0.1 mg l–1), and then rooted in 1/2 MS with NAA (0.1 mg l–1) at 25°C with a 16 h light/8 h dark cycle. Six-week-old rooted seedlings were used in all of the subsequent experiments. Nicotiana benthamiana seeds were sown in a soil medium consisting of a mixture of loam, vermiculite and perlite (1 : 1 : 1) and grown in an environmental chamber at 22°C with a 16 h light/8 h dark cycle. The seedlings were subsequently used for co-transformation experiments 6 weeks after germination of the seeds. Botryosphaeria dothidea strain LW48 was cultured on potato dextrose agar (PDA) in the dark at 25°C and irradiated with UV light to induce sporulation. Conidia were rinsed from the surface of the culture with water and the resulting mixture was passed through cheese cloth in order to remove mycelial debris. The concentration of the condial suspension was adjusted with sterile water to 6 × 10–6 condia ml–1.
Pathogen inoculation and SA treatment
Whole plants or isolated leaves were sprayed with the conidial suspension or with SA (0.1 mM; Sigma) for use in the gene expression analyses and the promoter functional assays. Plants that were treated with distilled water served as a mock treatment. Samples were collected at different time points and were subsequently frozen in liquid nitrogen and stored at –70°C. For the GUS assay, they were immediately utilized after collection. In order to evaluate the resistance of transiently transformed leaves, 10 µl of the B. dothidea conidial suspension was pipetted on to the lamina of each leaf, avoiding the main veins. All plant materials were maintained in a 100% relative humidity environment after inoculation.
Extraction of genomic DNA and RNA, and first-strand cDNA synthesis
Genomic DNA was extracted using the method reported by Gusberti et al. (2012). Total RNA was extracted using the Plant RNeasy Extraction Plus kit (Fuji) and LMW RNA was isolated using the polyethylene glycol (PEG) method (Wang et al. 2010). First-strand cDNA was synthesized using the PrimeScript RT reagent Kit with gDNA Eraser (TAKARA). A stem–loop reverse transcription primer was used for mature miR168 and gene-specific primers were utilized for pre-miR168a and 5.8 s rRNA (Supplementary Table S4).
Analysis of gene expression
Stem–loop RT-qPCR was used to determine the expression levels of mature miR168 with 5.8 s rRNA as an internal control. The transcript levels of pre-miR168a, the target gene and other genes were quantified using RT-qPCR and normalized using Elongation factor 1-α (EF-1α) as a housekeeping gene. The RT-qPCR was carried out on an ABI 7300 Real-Time PCR System (Applied Biosystems), using the SYBR Premix Ex Taq reaction system (TAKARA). Each analysis consisted of three biological replicates and three technical replicates of each biorep. For Northern blot, 50 µg of total or LMW RNA was loaded in each lane. Blots were hybridized using digoxigenin (DIG)-labeled nucleic acid probes. Signals were detected according to the manufacturer’s instructions for the DIG Luminescent Detection Kit (Roche), and U6 or EF-1α was used as the loading control. The gene-specific primers and probes used in the RT-qPCR and northern blot assay are listed in Supplementary Table S4.
Gene cloning and the 5′ RLM-RACE assay
A DNA fragment containing the miR168a precursor and its flanking regions was cloned from M. hupehensis genomic DNA. Pre-miR168 was amplified using gene-specific primers. The coding region of the candidate target gene was isolated from M. hupehensis cDNA using primers designed based on the sequence downloaded from the GDR. A 2 kb region upstream from the TSS of MIR168a and MhAGO1 was isolated from M. hupehensis genomic DNA by nested PCR. The obtained DNA fragments were designated as the promoter regions of MIR168a and MhAGO1. The cleavage site in the miR168 gene was verified by 5′ RLM-RACE using a FirstChoice® RLM-RACE Kit (Ambion) according to the manufacturer’s instructions. The 5′ RACE cDNA was synthesized from mRNA ligated with a 5′ adaptor using random decamers. Nested PCR was performed to obtain the 3′ cleavage products of the target gene using a set of adaptors and gene-specific primers. The 5′ RACE cDNA was also used to detect the accumulation of 3′ cleavage products by RT-qPCR. Adaptors and adaptor primers were provided with the kit. Gene-specific primers are listed in Supplementary Table S4. All PCR products were cloned into the pMD19-T Easy vector (TAKARA) and sequenced by Invitrogen Biotechnology Co. Ltd.
Plasmid construction
All of the primers used to make the constructs are listed in Supplementary Table S4.
35SCaMV::MIR168a construct. A DNA fragment containing the precursor of miR168a was amplified. The GUS coding region of binary vector pBI121 was excised and replaced with the miR168a precursor.
35SCaMV::MhAGO1-GUS and 35SCaMV::mMhAGO1-GUS constructs. The full-length MhAGO1 or mMhAGO1 fragment (without the stop codon) was inserted into the pBI121 vector to fuse with the GUS gene to generate the complete construct. Point mutations were introduced to obtain the mutated MhAGO1 using the GeneArt Site-Directed Mutagenesis System kit (Invitrogen) following the manufacturer’s instructions.
pMIR168a::GUS and pMhAGO1::GUS constructs. The 2 kb promoter regions of MIR168a and MhAGO1 were cloned into pBI121 to replace the 35SCaMV promoter and were used to drive the GUS gene.
35SCaMV::STTM168 construct. Using a method modified from Yan et al. (2012), a nucleotide fragment containing two copies of miR168 partially complementary sequences linked by a 48 bp short spacer was artificially synthesized.
RNAi construct. A fragment harboring sense and antisense sequences of a 25 bp segment in the MhAGO1 gene separated by a 20 bp intron of the GUS gene was prepared by PCR (Ifuku et al. 2005).
Agrobacterium-mediated transient transformation
A single colony of Agrobacterium tumefaciens EHA105 (containing the recombinant plasmid) was cultured in YEB liquid medium supplied with rifampin (50 µg ml–1) and kanamycin (50 µg ml–1). After culturing, the bacteria were pelleted and resuspended in suspension buffer (10 mM MES, 10 mM MgCl2, pH 5.6). The bacterial suspension was then adjusted to OD600 = 0.7, and 100 µmol l–1 acetosyringone was added before the suspension was used for infiltration.
Tobacco co-transformation. The leaves of 6-week-old tobacco plants were infiltrated with the bacterial suspension by injection. Tiny holes were made in the tobacco leaves using syringe needles. The bacterial suspension was then injected into those tiny holes with a needleless syringe. Four holes were made on each leaf, and 5 ml of the bacterial suspension were administered into each hole. The infiltrated seedlings were then moved back to the environmental chamber and kept in the dark for 3 d.
Apple transient transformation. The third and fourth fully expanded leaves of 6-week-old rooted tissue cultured plants of M. hupehensis were detached, immersed in a 50 ml bacterial suspension and vacuum infiltrated. The infiltrated leaves were placed adaxial surface up in covered containers with the petiole inserted into an agar block. The containers were then sealed with parafilm and kept for 2 d at 25°C using a 16 h light/8 h dark cycle.
GUS assay
Histochemical GUS staining of infiltrated leaves was performed as described by Jefferson et al. (1987). Leaves were then immersed in ethanol to remove Chl. Quantification of GUS activity was determined using the fluorometric 4-methylumbelliferyl-β-d-glucuronide (MUG) method (Jefferson et al. 1987). One unit of GUS activity was defined as 1 nM of 4-methylumbelliferon (4-MU) generated per minute per milligram of soluble protein. Ten leaves were infiltrated for each construct in each independent experiment, and then combined to detect GUS activity.
Evaluation of disease severity and colonization coefficient calculation in infected leaves
The disease progress was categorized based on the area of the necrotic lesion relative to the total leaf area as follows: level 1, < 1%; level 2, 1–10%; level 3, 11–25%; level 4, 26–40%; level 5, 41–65%; and level 6, > 66%. At least 50 leaves infiltrated with each construct were examined in three independent experiments.
Quantification of H2O2 generation and cell death
The modified colorimetric method described by Zhou et al. (2006) was used to quantify H2O2 levels in infected leaves. The results were expressed as nmol g−1 FW. Cell death within infected leaves was quantified by measuring the uptake of Evans blue as described by Baker and Mock (1994). Ten leaves for each construct were harvested and infiltrated with Evans blue dye at each time point post-inoculation or post-mock inoculation, and examined. For the image analysis, decolorized leaves were scanned. The pixel numbers of stained areas and the total leaf areas were measured via the Adobe Photoshop histogram tool. The cell death level was presented by the value of the ratio of pixel numbers.
Bioinformatics analysis
The precursor sequence of miR168a was obtained from the database, miRbase 21 (http://www.mirbase.org/) (Kozomara and Griffiths-Jones 2013) and mapped to the M. domestica genome. Potential targets of miR168a were predicted using psRNATarget (http://plantgrn.noble.org/psRNATarget/) (Dai and Zhao 2011), using M. domestica coding sequences downloaded from the GDR. Conserved motifs of the putative amino acid sequence of the target gene were identified by Pfam (http://pfam.xfam.org/) (Finn et al. 2014). Multiple sequence alignment of Argonuaute proteins was conducted using Clustal Omega (http://www.ebi.ac.uk/Tools/msa/clustalo/). Phylogenetic and homology trees were generated by MEGA 6.0 using the Neighbor–Joining method. The TSS was identified using TSSP/Prediction of PLANT Promoters (http://linux1.softberry.com/). For cis-acting element analysis, an approximately 2 kb fragment upstream of the TSS was scanned for known cis- elements with the PLACE database (http://www.dna.affrc.go.jp/PLACE/) (Hieno et al. 2014).
Statistical analysis
The presented data represent the mean ± SD of three independent experiments.
A t-test (P ≤ 0.01) was used to determine significant differences between samples with IBM SPSS 19.0 (IBM® SPSS® Statistics, IBM Corporation) software.
Supplementary data
Supplementary data are available at PCP online.
Funding
This work was supported by an Agricultural science and technology innovation fund project in Jiangsu Province [project No. CX(15)1022].
Abbreviations
- 6-BA
6-benzylaminopurine
- CDs
coding DNA sequence
- EF-1α
elongation factor 1-α
- ETI
effector-triggered-immunity
- GDR
Genome Database for Rosaceae
- GUS
β-glucuronidase
- hpi
hours post-inoculation
- LMW
low molecular weight
- miRNA
microRNA
- MS
Murashige and Skoog
- MUG
4-methylumbelliferyl-β-d-glucuronide
- NAA
1-naphthaleneacetic acid
- ORF
open reading frame
- PDA
potato dextrose agar
- PR
pathogenesis related
- PTI
pathogen-associated molecular pattern (PAMP)-triggered immunity
- RT-qPCR
real-time quantitative PCR
- RISC
RNA-induced silencing complex
- RLM-RACE
RNA ligase-mediated rapid amplification of cDNA ends
- RNAi
RNA interference
- ROS
reactive oxygen species
- SA
salicylic acid; siRNA, small interfering RNA
- TSS
transcription start site
Footnote
The nucleotide sequence reported in this paper has been submitted to the GenBank database with accession number KU926967
Acknowledgments
We would like to thank Professor Zhihong Gao and Professor Aisheng Xiong for their critical review of the manuscript.
Disclosures
The authors have no conflicts of interest to declare.