-
PDF
- Split View
-
Views
-
Cite
Cite
Eric Wenzler, Maressa Santarossa, Kevin A Meyer, Amanda T Harrington, Gail E Reid, Nina M Clark, Fritzie S Albarillo, Zackery P Bulman, In Vitro Pharmacodynamic Analyses Help Guide the Treatment of Multidrug-Resistant Enterococcus faecium and Carbapenem-Resistant Enterobacter cloacae Bacteremia in a Liver Transplant Patient, Open Forum Infectious Diseases, Volume 7, Issue 1, January 2020, ofz545, https://doi.org/10.1093/ofid/ofz545
- Share Icon Share
Abstract
Infections due to multidrug-resistant pathogens are particularly deadly and difficult to treat in immunocompromised patients, where few data exist to guide optimal antimicrobial therapy. In the absence of adequate clinical data, in vitro pharmacokinetic (PK)/pharmacodynamic (PD) analyses can help to design treatment regimens that are bactericidal and may be clinically effective.
We report a case in which in vitro pharmacodynamic analyses were utilized to guide the treatment of complex, recurrent bacteremias due to vancomycin-, daptomycin-, and linezolid-resistant Enterococcus faecium and carbapenem-resistant Enterobacter cloacae complex in a liver transplant patient.
Whole-genome sequencing revealed unique underlying resistance mechanisms and explained the rapid evolution of phenotypic resistance and complicated intrahost genomic dynamics observed in vivo. Performing this comprehensive genotypic and phenotypic testing and time-kill analyses, along with knowledge of institution and patient-specific factors, allowed us to use precision medicine to design a treatment regimen that maximized PK/PD.
This work provides a motivating example of clinicians and scientists uniting to optimize care in the era of escalating antimicrobial resistance.
The concomitant increase in bacterial resistance and decrease in novel antimicrobial agents has forced clinicians and scientists to find ways to optimize the use of our current antimicrobial armamentarium. Combination antimicrobial therapy is a commonly utilized optimization strategy, especially against multiply drug-resistant pathogens, to provide synergistic activity and reduce the potential for further resistance development. Combination therapy is routinely recommended for serious infections due to enterococci [1] and is often employed against resistant Gram-negative pathogens, especially carbapenem-resistant Enterobacteriaceae (CRE) [2]. Unfortunately, the optimal combination regimen for these pathogens is unknown, as clinical data are lacking and translation of in vitro data from the benchtop to clinical practice can be challenging. As such, clinicians are often forced to make an educated guess as to the best combination based on external clinical and in vitro data, patient-specific factors, and the pharmacokinetics (PK), pharmacodynamics (PD), and toxicodynamics (TD) of given antimicrobial agents.
In vitro PD models such as time-kill analyses are able to evaluate the bactericidal activity of antibiotics and are the gold standard for evaluating antimicrobial synergy. Unfortunately, these models are not always clinically translatable, as they may use clinically irrelevant drugs, supratherapeutic doses, and/or only evaluate a small set of pathogens, which may not represent the most prevalent strains. To be most useful clinically, these analyses would be performed ex vivo against the specific patient’s infecting pathogen vs the antimicrobial(s) alone and in combinations that are relevant to that patient’s case based on the patient’s organ function, allergies, hospital formulary, etc. This approach would allow for evidence-based therapy optimization and precision medicine against extremely difficult-to-treat pathogens. Herein we provide a motivating example that supports the use of time-kill analyses to guide clinical decision-making for combination antimicrobial therapy in patients with complex and multidrug-resistant infections. We report on the use of pharmacodynamic analyses to help guide the treatment of complex multidrug-resistant Enterococcus faecium and Enterobacter cloacae complex bacteremia in a critically ill immunocompromised liver transplant patient. Additionally, whole-genome sequencing was performed to elucidate the underlying mechanisms of resistance in these pathogens with abstruse phenotypic resistance profiles.
PATIENT CASE
The patient was a 30-year-old female with a medical history of autoimmune hepatitis who received an orthotopic liver transplant (OLT). Approximately 6 months post-transplant, she developed a right lobar hepatic abscess and successive bacteremias due to third-generation cephalosporin-resistant Enterobacter cloacae complex and daptomycin-susceptible (minimum inhibitory concentration [MIC], 4 mg/L), vancomycin-resistant Enterococcus faecium (VRE), for which she received treatment with ertapenem and daptomycin (~11 mg/kg/d), respectively (Figure 1). Blood cultures initially cleared after ~1 week of therapy, but after ~4 weeks of treatment with this combination (~day 29), blood cultures again grew daptomycin-susceptible VRE, whereas cultures from the liver abscess grew daptomycin-resistant (MIC, 16 mg/L) VRE. Ampicillin was added to daptomycin and ertapenem given this elevated MIC to daptomycin, and blood cultures cleared again by day 32. Roughly 10 days later, blood cultures again grew VRE, which was now also daptomycin-resistant (E. faecium UIC1) (Table 1). Accordingly, the dose of daptomycin was increased from ~11 to ~14 mg/kg/d, linezolid was added, and ampicillin was switched to ceftaroline. A tracheal aspirate culture from the same day also grew E. cloacae complex, which was now phenotypically carbapenem-resistant, and therefore ertapenem was switched to levofloxacin. Blood cultures became VRE-negative after ~2 weeks of therapy with this triple combination, and the patient was stable enough to be discharged on ~day 80.
Genotypic and Phenotypic Susceptibilities and Interpretive Categorya of Pathogens to Tested Antimicrobials
. | E. faecium UIC1 . | . | E. faecium UIC2 . | . | E. hormaechei . | . |
---|---|---|---|---|---|---|
Resistance Genes . | aac(6’)-Ii, ant(6)-Ia, aph(3’)-III, dfrG, erm(B), erm(T), msr(C), tet(L), tet(M), vanA . | . | aac(6’)-Ii, aac(6’)-aph(2’’), dfrG, erm(T), msr(C), tet(L), tet(M) . | . | blaACT-7, fosA, mdf(A) . | . |
Antibioticb . | MIC, mg/L . | Interpretive Category . | MIC, mg/L . | Interpretive Category . | MIC, mg/L . | Interpretive Category . |
Ampicillin | >256* | R | >256* | R | ≥16* | I |
Ampicillin-sulbactam | - | - | - | - | >16/8* | R |
Aztreonam | - | - | - | - | >256* | R |
Cefazolin | - | - | - | - | ≥32* | R |
Cefepime | - | - | - | - | ≥32* | R |
Ceftaroline | >64 | NC | ≥128 | NC | ≥8 | R |
Ceftazidime | - | - | - | - | ≥128* | R |
Ceftazidime-avibactam | - | - | - | - | 8* | S |
Ceftolozane-tazobactam | - | - | - | - | - | R |
Ceftriaxone | >256 | NC | ≥128 | NC | ≥128* | R |
Chloramphenicol | 4 | S | ≥32 | R | 16 | I |
Dalbavancind | >2 | R | 0.12 | S | - | - |
Daptomycin | 32* | R | 4* | SDD | - | - |
Eravacycline | - | - | - | - | 1 | S |
Ertapenem | 128 | NC | - | - | ≥64* | R |
Erythromycin | ≥8 | R | 2 | I | - | - |
Fosfomycind | 16 | S | 64 | S | >1024 | R |
Gentamicin | 2* | NC | 8* | NC | ≤1* | S |
Levofloxacin | ≥8 | R | ≥8 | R | 2* | R |
Linezolid | 1* | S | >256* | R | - | - |
Meropenem | - | - | - | - | 16* | R |
Meropenem-vaborbactam | - | - | - | - | 8* | I |
Nitrofurantoin | ≥128 | R | ≥128 | R | 64 | I |
Oritavancind | 0.03 | S | 0.004 | S | - | - |
Piperacillin-tazobactam | - | - | - | - | ≥128* | R |
Plazomicin | - | - | - | - | 1 | S |
Polymyxin Be | - | - | - | - | 0.25* | S |
Quinupristin-dalfopristinf | 0.5* | S | 0.5* | S | - | - |
Rifampin | 2 | I | 0.5 | S | ≥4 | NC |
Tedizolidd | 0.5 | S | ≥8 | R | - | - |
Telavancin | ≥4 | R | 0.06 | S | - | - |
Tetracycline | ≥16 | R | ≥16 | R | ≥16 | R |
Tigecyclineg,* | 2 | NS | 2 | NS | 2* | S |
Tobramycin | - | - | - | - | ≤2* | S |
Trimethoprim-sulfamethoxazole | - | - | - | - | <2/38* | S |
Vancomycin | >256* | R | 1* | S | - | - |
. | E. faecium UIC1 . | . | E. faecium UIC2 . | . | E. hormaechei . | . |
---|---|---|---|---|---|---|
Resistance Genes . | aac(6’)-Ii, ant(6)-Ia, aph(3’)-III, dfrG, erm(B), erm(T), msr(C), tet(L), tet(M), vanA . | . | aac(6’)-Ii, aac(6’)-aph(2’’), dfrG, erm(T), msr(C), tet(L), tet(M) . | . | blaACT-7, fosA, mdf(A) . | . |
Antibioticb . | MIC, mg/L . | Interpretive Category . | MIC, mg/L . | Interpretive Category . | MIC, mg/L . | Interpretive Category . |
Ampicillin | >256* | R | >256* | R | ≥16* | I |
Ampicillin-sulbactam | - | - | - | - | >16/8* | R |
Aztreonam | - | - | - | - | >256* | R |
Cefazolin | - | - | - | - | ≥32* | R |
Cefepime | - | - | - | - | ≥32* | R |
Ceftaroline | >64 | NC | ≥128 | NC | ≥8 | R |
Ceftazidime | - | - | - | - | ≥128* | R |
Ceftazidime-avibactam | - | - | - | - | 8* | S |
Ceftolozane-tazobactam | - | - | - | - | - | R |
Ceftriaxone | >256 | NC | ≥128 | NC | ≥128* | R |
Chloramphenicol | 4 | S | ≥32 | R | 16 | I |
Dalbavancind | >2 | R | 0.12 | S | - | - |
Daptomycin | 32* | R | 4* | SDD | - | - |
Eravacycline | - | - | - | - | 1 | S |
Ertapenem | 128 | NC | - | - | ≥64* | R |
Erythromycin | ≥8 | R | 2 | I | - | - |
Fosfomycind | 16 | S | 64 | S | >1024 | R |
Gentamicin | 2* | NC | 8* | NC | ≤1* | S |
Levofloxacin | ≥8 | R | ≥8 | R | 2* | R |
Linezolid | 1* | S | >256* | R | - | - |
Meropenem | - | - | - | - | 16* | R |
Meropenem-vaborbactam | - | - | - | - | 8* | I |
Nitrofurantoin | ≥128 | R | ≥128 | R | 64 | I |
Oritavancind | 0.03 | S | 0.004 | S | - | - |
Piperacillin-tazobactam | - | - | - | - | ≥128* | R |
Plazomicin | - | - | - | - | 1 | S |
Polymyxin Be | - | - | - | - | 0.25* | S |
Quinupristin-dalfopristinf | 0.5* | S | 0.5* | S | - | - |
Rifampin | 2 | I | 0.5 | S | ≥4 | NC |
Tedizolidd | 0.5 | S | ≥8 | R | - | - |
Telavancin | ≥4 | R | 0.06 | S | - | - |
Tetracycline | ≥16 | R | ≥16 | R | ≥16 | R |
Tigecyclineg,* | 2 | NS | 2 | NS | 2* | S |
Tobramycin | - | - | - | - | ≤2* | S |
Trimethoprim-sulfamethoxazole | - | - | - | - | <2/38* | S |
Vancomycin | >256* | R | 1* | S | - | - |
Abbreviations: -, not tested; CLSI, Clinical and Laboratory Standards Institute; I, intermediate; MIC, minimum inhibitory concentration; NC, no CLSI interpretive category; NS, nonsusceptible; R, resistant; S, susceptible; SDD, susceptible dose-dependent.
aAccording to CLSI M100-S29.
bAgents for which susceptibility testing was initially performed by the clinical microbiology laboratory and were immediately available to the clinical team before additional analyses in the research laboratory are indicated with an “*.” Otherwise, additional minimum inhibitory concentrations reported in this table are those obtained from the research laboratory.
cSusceptibility determined via disk diffusion.
dInterpreted according to CLSI breakpoint for vancomycin-susceptible E. faecalis.
eInterpreted according to CLSI breakpoint for Pseudomonas aeruginosa.
fInterpreted according to CLSI breakpoint for vancomycin-resistant E. faecium.
gInterpreted according to Food and Drug Administration breakpoints for vancomycin-susceptible E. faecalis and Enterobacteriaceae, respectively.
Genotypic and Phenotypic Susceptibilities and Interpretive Categorya of Pathogens to Tested Antimicrobials
. | E. faecium UIC1 . | . | E. faecium UIC2 . | . | E. hormaechei . | . |
---|---|---|---|---|---|---|
Resistance Genes . | aac(6’)-Ii, ant(6)-Ia, aph(3’)-III, dfrG, erm(B), erm(T), msr(C), tet(L), tet(M), vanA . | . | aac(6’)-Ii, aac(6’)-aph(2’’), dfrG, erm(T), msr(C), tet(L), tet(M) . | . | blaACT-7, fosA, mdf(A) . | . |
Antibioticb . | MIC, mg/L . | Interpretive Category . | MIC, mg/L . | Interpretive Category . | MIC, mg/L . | Interpretive Category . |
Ampicillin | >256* | R | >256* | R | ≥16* | I |
Ampicillin-sulbactam | - | - | - | - | >16/8* | R |
Aztreonam | - | - | - | - | >256* | R |
Cefazolin | - | - | - | - | ≥32* | R |
Cefepime | - | - | - | - | ≥32* | R |
Ceftaroline | >64 | NC | ≥128 | NC | ≥8 | R |
Ceftazidime | - | - | - | - | ≥128* | R |
Ceftazidime-avibactam | - | - | - | - | 8* | S |
Ceftolozane-tazobactam | - | - | - | - | - | R |
Ceftriaxone | >256 | NC | ≥128 | NC | ≥128* | R |
Chloramphenicol | 4 | S | ≥32 | R | 16 | I |
Dalbavancind | >2 | R | 0.12 | S | - | - |
Daptomycin | 32* | R | 4* | SDD | - | - |
Eravacycline | - | - | - | - | 1 | S |
Ertapenem | 128 | NC | - | - | ≥64* | R |
Erythromycin | ≥8 | R | 2 | I | - | - |
Fosfomycind | 16 | S | 64 | S | >1024 | R |
Gentamicin | 2* | NC | 8* | NC | ≤1* | S |
Levofloxacin | ≥8 | R | ≥8 | R | 2* | R |
Linezolid | 1* | S | >256* | R | - | - |
Meropenem | - | - | - | - | 16* | R |
Meropenem-vaborbactam | - | - | - | - | 8* | I |
Nitrofurantoin | ≥128 | R | ≥128 | R | 64 | I |
Oritavancind | 0.03 | S | 0.004 | S | - | - |
Piperacillin-tazobactam | - | - | - | - | ≥128* | R |
Plazomicin | - | - | - | - | 1 | S |
Polymyxin Be | - | - | - | - | 0.25* | S |
Quinupristin-dalfopristinf | 0.5* | S | 0.5* | S | - | - |
Rifampin | 2 | I | 0.5 | S | ≥4 | NC |
Tedizolidd | 0.5 | S | ≥8 | R | - | - |
Telavancin | ≥4 | R | 0.06 | S | - | - |
Tetracycline | ≥16 | R | ≥16 | R | ≥16 | R |
Tigecyclineg,* | 2 | NS | 2 | NS | 2* | S |
Tobramycin | - | - | - | - | ≤2* | S |
Trimethoprim-sulfamethoxazole | - | - | - | - | <2/38* | S |
Vancomycin | >256* | R | 1* | S | - | - |
. | E. faecium UIC1 . | . | E. faecium UIC2 . | . | E. hormaechei . | . |
---|---|---|---|---|---|---|
Resistance Genes . | aac(6’)-Ii, ant(6)-Ia, aph(3’)-III, dfrG, erm(B), erm(T), msr(C), tet(L), tet(M), vanA . | . | aac(6’)-Ii, aac(6’)-aph(2’’), dfrG, erm(T), msr(C), tet(L), tet(M) . | . | blaACT-7, fosA, mdf(A) . | . |
Antibioticb . | MIC, mg/L . | Interpretive Category . | MIC, mg/L . | Interpretive Category . | MIC, mg/L . | Interpretive Category . |
Ampicillin | >256* | R | >256* | R | ≥16* | I |
Ampicillin-sulbactam | - | - | - | - | >16/8* | R |
Aztreonam | - | - | - | - | >256* | R |
Cefazolin | - | - | - | - | ≥32* | R |
Cefepime | - | - | - | - | ≥32* | R |
Ceftaroline | >64 | NC | ≥128 | NC | ≥8 | R |
Ceftazidime | - | - | - | - | ≥128* | R |
Ceftazidime-avibactam | - | - | - | - | 8* | S |
Ceftolozane-tazobactam | - | - | - | - | - | R |
Ceftriaxone | >256 | NC | ≥128 | NC | ≥128* | R |
Chloramphenicol | 4 | S | ≥32 | R | 16 | I |
Dalbavancind | >2 | R | 0.12 | S | - | - |
Daptomycin | 32* | R | 4* | SDD | - | - |
Eravacycline | - | - | - | - | 1 | S |
Ertapenem | 128 | NC | - | - | ≥64* | R |
Erythromycin | ≥8 | R | 2 | I | - | - |
Fosfomycind | 16 | S | 64 | S | >1024 | R |
Gentamicin | 2* | NC | 8* | NC | ≤1* | S |
Levofloxacin | ≥8 | R | ≥8 | R | 2* | R |
Linezolid | 1* | S | >256* | R | - | - |
Meropenem | - | - | - | - | 16* | R |
Meropenem-vaborbactam | - | - | - | - | 8* | I |
Nitrofurantoin | ≥128 | R | ≥128 | R | 64 | I |
Oritavancind | 0.03 | S | 0.004 | S | - | - |
Piperacillin-tazobactam | - | - | - | - | ≥128* | R |
Plazomicin | - | - | - | - | 1 | S |
Polymyxin Be | - | - | - | - | 0.25* | S |
Quinupristin-dalfopristinf | 0.5* | S | 0.5* | S | - | - |
Rifampin | 2 | I | 0.5 | S | ≥4 | NC |
Tedizolidd | 0.5 | S | ≥8 | R | - | - |
Telavancin | ≥4 | R | 0.06 | S | - | - |
Tetracycline | ≥16 | R | ≥16 | R | ≥16 | R |
Tigecyclineg,* | 2 | NS | 2 | NS | 2* | S |
Tobramycin | - | - | - | - | ≤2* | S |
Trimethoprim-sulfamethoxazole | - | - | - | - | <2/38* | S |
Vancomycin | >256* | R | 1* | S | - | - |
Abbreviations: -, not tested; CLSI, Clinical and Laboratory Standards Institute; I, intermediate; MIC, minimum inhibitory concentration; NC, no CLSI interpretive category; NS, nonsusceptible; R, resistant; S, susceptible; SDD, susceptible dose-dependent.
aAccording to CLSI M100-S29.
bAgents for which susceptibility testing was initially performed by the clinical microbiology laboratory and were immediately available to the clinical team before additional analyses in the research laboratory are indicated with an “*.” Otherwise, additional minimum inhibitory concentrations reported in this table are those obtained from the research laboratory.
cSusceptibility determined via disk diffusion.
dInterpreted according to CLSI breakpoint for vancomycin-susceptible E. faecalis.
eInterpreted according to CLSI breakpoint for Pseudomonas aeruginosa.
fInterpreted according to CLSI breakpoint for vancomycin-resistant E. faecium.
gInterpreted according to Food and Drug Administration breakpoints for vancomycin-susceptible E. faecalis and Enterobacteriaceae, respectively.
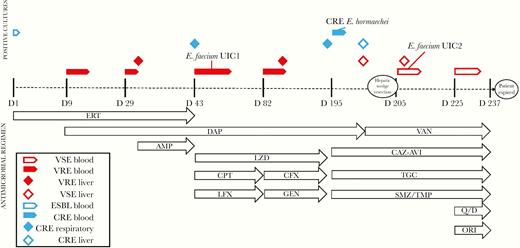
Evolution of bacterial resistance and schematic of antimicrobial administration over the post-transplant infectious period. Timeline is not to scale. AMP, ampicillin; CAZ-AVI, ceftazidime-avibactam; CFX, ceftriaxone; CPT, ceftaroline; DAP, daptomycin; ERT, ertapenem; GEN, gentamicin; LFX, levofloxacin; LZD, linezolid; ORI, oritavancin; Q/D, quinipristin-dalfopristin; SMZ/TMP, sulfamethoxazole-trimethoprim; TGC, tigecycline; VAN, vancomycin.
Three days after discharge, the patient was re-admitted with fevers, and meropenem was briefly added empirically to daptomycin, linezolid, and ceftaroline. Blood and hepatic abscess cultures again were positive for daptomycin-resistant VRE. Based on results from time-kill analyses of E. faecium UIC1 (Figure 2), therapy was switched to daptomycin, ceftriaxone, and gentamicin. Linezolid was continued by the primary medical team. This combination cleared her bacteremia in <1 week, and she was subsequently discharged on day 20 of this admission (~day 100 overall). Follow-up blood cultures remained negative for 3 months (~days 100–190) while maintained on this regimen, with the exception of gentamicin, which was discontinued after ~30 days (~day 130) due to elevated serum creatinine.
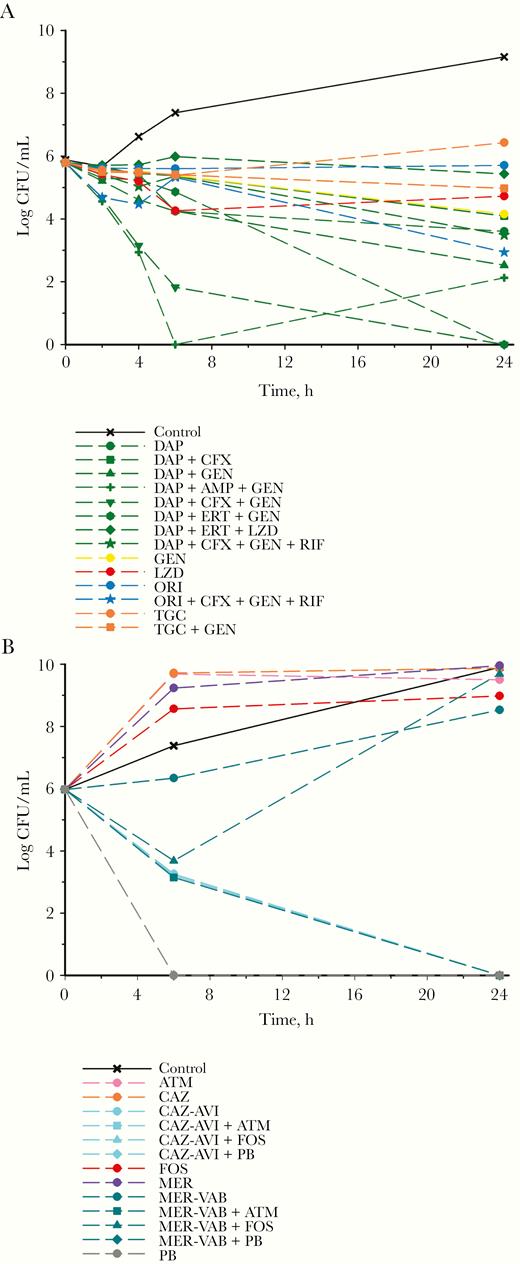
Mean log10 CFU/mL vs time profile for each individual drug and combination against Enterococcus faecium UIC1 (A) and Enterobacter hormaechei (B). Curves represent average concentrations for triplicate experiments. Abbreviations: AMP, ampicillin; ATM, aztreonam; CAZ, ceftazidime; CAZ-AVI, ceftazidime-avibactam; CFX, ceftriaxone; DAP, daptomycin; ERT, ertapenem; FOS, fosfomycin; GEN, gentamicin; LZD, linezolid; MER, meropenem; MER-VAB, meropenem-vaborbctam; ORI, oritavancin; PB, polymyxin B; RIF, rifampin; TGC, tigecycline.
Approximately 11 months post-transplant, the patient was again readmitted with acute kidney injury and hypervolemia. Blood and respiratory cultures from this admission demonstrated carbapenem-resistant, carbapenemase-negative E. cloacae complex according to the BioFire FilmArray BCID panel (in vitro diagnostic [IVD]; BioFire Diagnostics, LLC, Salt Lake City, UT, USA), Xpert Carba-R (IVD; Cepheid, Sunnyvale, CA, USA), and Enterobacter spp. via the Verigene BC-GN system (research-use only [RUO]; Nanosphere, Northbrook, IL, USA) (Table 1). Ceftazidime-avibactam demonstrated bactericidal activity alone against the CRE in time-kill analyses (Figure 2) and was therefore initiated, along with sulfamethoxazole/trimethoprim and tigecycline, based on in vitro susceptibilities. Blood cultures cleared within 3 days. She remained on quadruple drug therapy (daptomycin, ceftriaxone, gentamicin, and linezolid) for her previous VRE infections until repeat hepatic abscess cultures grew a daptomycin- and vancomycin-susceptible, linezolid-resistant E. faecium (E. faecium UIC2) (Table 1). As such, antibiotics were switched from daptomycin, ceftriaxone, gentamicin, and linezolid to vancomycin (~day 195–200) (Figure 1), although blood cultures continued to grow vancomycin-susceptible E. faecium despite additional therapy with quinupristin/dalfopristin and oritavancin (added on ~day 225) (Figure 1). Finally, the patient developed massive pulmonary hemorrhage and multisystem organ failure and ultimately succumbed to her disease ~8 months after initially discovering the hepatic abscess.
METHODS
Bacteria and Susceptibility Testing
Initial organism identification and susceptibility determination were performed via matrix-assisted laser-desorption ionization time-of-flight mass spectrometry (MALDI-TOF MS) (Bruker Daltonics, Billerica, MA, USA) and MicroScan Walkaway (Beckman Coulter, Brea, CA, USA), respectively, in the clinical microbiology laboratory. All subsequent testing was performed in the research laboratory. Identification and resistance marker detection was subsequently confirmed via Verigene blood culture assay (NanoSphere, Northbrook, IL, USA). For phenotypic testing of the E. cloacae complex isolate, the modified Hodge test, carbapenem inactivation method, modified carbapenem inactivation method, and extended-spectrum beta-lactamase (ESBI) disk diffusion testing were performed according to clinical and laboratory standards institute (CLSI) guidelines [3]. Additionally, the metallo-beta-lactamase (MBL) Etest (bioMerieux, Marcy-L’Etoile, France) and Carba5 lateral flow immunoassay (Hardy Diagnostics, Santa Maria, CA, USA) were performed according to the manufacturer’s instructions.
Analytical grade ampicillin, avibactam, aztreonam, ceftazidime, ceftriaxone, daptomycin, ertapenem, fosfomycin, gentamicin, linezolid, meropenem, oritavancin, polymyxin B, rifampin, tigecycline, and vancomycin (Sigma-Aldrich, St. Louis, MO, USA), along with vaborbactam (MedChemExpress, Monmouth Junction, NJ, USA), were obtained commercially for broth microdilution testing. IVD Etest strips for ceftazidime-avibactam (bioMerieux, Marcy-l’Etoile, France), RUO Etest strips for eravacycline and plazomicin (Liofilchem, Roseto degli Abruzzi, Italy), and GPALL3F and FDANDPF Sensititre AST plates (ThermoFisher Scientific, Waltham, MA, USA) were utilized according to the manufacturer’s instructions. Minimum inhibitory concentrations (MICs) were determined in triplicate according to CLSI guidelines [3], with 0.002% polysorbate-80 added to assays containing oritavancin and the Ca2+ content of CAMHB increased to 50 mg/L for assays containing daptomycin. Nontissue culture-treated microtiter plates were used for all oritavancin assays to prevent any loss of drug potency [4]. Modal MIC values are reported.
Time-Kill Experiments
Time-kill experiments were performed as previously described [5–7]. Antimicrobial agents selected for time-kill analyses alone and in combination were based on known or presumed in vitro susceptibility or synergy, institutional formulary, patient’s organ function, antimicrobial stewardship considerations, and discussion with the infectious diseases consult team. Maximum free drug plasma concentrations (fCmax) of each antibiotic were used according to the dose being administered to the patient clinically (Supplementary Table 1).
Whole-Genome Sequencing
Each of the patient’s 3 isolates (E. faecium UIC1, E. faecium UIC2, and E. cloacae complex) retrospectively underwent whole-genome sequencing to identify antimicrobial resistance mechanisms. Genomic DNA was extracted using the QIAmp and HT DNA Kit (Qiagen, Hilden, Germany) and library-prepared using the Nextera XT library prep kit from Illumina. Paired-end genome sequencing was performed on an Illumina MiSeq (Illumina, San Diego, CA, USA) 2×150-bp configuration (GENEWIZ, Inc., South Plainfield, NJ, USA). Adapter sequences were trimmed, and low-quality bases were removed using BBDuk 37.64. De novo genome assembly was performed using SPAdes 3.10 [8]. Antimicrobial resistance genes were initially identified by BLAST-searching the derived contigs for each organism against the ResFinder 3.1 [9] and CARD-RGI [10] databases. Single nucleotide polymorphisms in the liaFSR operon and cls gene (cardiolipin synthetase) in E. faecium were identified vs reference E. faecium ATCC 700221 (accession number: CP014449). To identify possible linezolid resistance–conferring mutations in the E. faecium isolates, linezolid resistance in enterococci (LRE) finder was used [11]. To identify additional carbapenem resistance–conferring mutations in the E. cloacae complex isolate, ompC and ompF sequences were compared with reference sequences from E. cloacae 1537504 (accession numbers: KY086510.1 and KY086519.1).
To determine the genetic relatedness of the patient’s 2 E. faecium isolates, comparative genomics were used for the assembled sequences of E. faecium UIC1 and UIC2. Pairwise whole-genome alignment was performed with MUMmer 3.0 [12] to determine mismatches and the percent identity of the sequences. A mummerplot was generated to identify regions of the sequences with gaps or inversions. Each E. faecium sequence was also mapped to reference vanA plasmids pV24-5 and pS177 (accession numbers: CP036156 and HQ115078) using Bowtie2 [13].
RESULTS
Susceptibility
Phenotypic susceptibilities for each pathogen against the tested antimicrobial agents are displayed in Table 1. Notably, the E. faecium UIC1 isolate was daptomycin- and vancomycin-resistant but susceptible to the oxazolidinones, whereas E. faecium UIC2 was daptomycin- and vancomycin-susceptible, but oxazolidinone-resistant. The only agent with interpretive criteria for E. faecium that both isolates were susceptible to was quinupristin-dalfopristin, although fosfomycin and oritavancin had MICs within the susceptible range for E. faecalis.
The E. cloacae complex isolate was extensively drug-resistant and remained susceptible only to ceftazidime-avibactam, eravacycline, gentamicin, plazomicin, polymyxin B, tigecycline, tobramycin, and trimethoprim-sulfamethoxazole. Despite carbapenem resistance, all phenotypic tests for carbapenemase production were negative.
Time-Kill Analyses
Time-kill analyses were performed on E. faecium UIC1 and E. cloacae complex in order to inform clinical treatment (Figure 2). Time-kill analyses on E. faecium UIC1 were performed during the second episode of VRE bacteremia (Figure 1), although due to the time required for completion, the patient cleared her bacteremia before the availability of these results. As such, they were utilized to design the antimicrobial regimen during the third episode of bacteremia (Figure 1). Against E. faecium UIC1, no single drug was bactericidal. Daptomycin plus gentamicin was bactericidal (3.27 log10 colony-forming units [CFU]/mL reduction at 24 hours), along with the triple combinations of daptomycin plus ampicillin and gentamicin (3.67 log10 CFU/mL reduction at 24 hours), daptomycin plus ertapenem and gentamicin (5.79 log10 CFU/mL reduction at 24 hours), and daptomycin plus ceftriaxone and gentamicin (5.79 log10 CFU/mL reduction at 24 hours). Time-kill analyses against the Enterobacter isolate were performed as soon as the carbapenem-resistant isolate was detected in blood cultures (~day 195) (Figure 1). Against the E. cloacae complex, ceftazidime-avibactam and polymyxin B alone and together were bactericidal and achieved bacterial eradication at 24 hours (5.97 log10 CFU/mL reduction for each). Meropenem-vaborbactam in combination with aztreonam also resulted in bactericidal activity, synergy, and bacterial eradication at 24 hours (5.97 log10 CFU/mL reduction).
Whole-Genome Sequencing
Molecular analysis of E. faecium UIC1 revealed resistance genes for aminoglycosides (aac(6’)-Ii, ant(6)-Ia, aph(3’)-III), trimethoprim (dfrG), macrolides/lincosamides/streptogramin B (erm(B), erm(T), msr(C)), tetracyclines (tet(L), tet(M)), and vancomycin (vanA). Whole-genome sequencing (WGS) of E. faecium UIC2 revealed resistance genes for aminoglycosides (aac(6’)-li), trimethoprim (dfrG), macrolides/lincosamides/streptogramin B (msr(C), erm(T)), and tetracyclines (tet(L)). Although mutations in the liaFRS operon and cardiolipin synthase gene (cls) have been previously shown to confer elevated MICs to daptomycin, the liaFRS and cls sequences were identical between E. faecium UIC1 and UIC2, and the liaF genes remained unaltered compared with E. faecium ATCC 700221. However, amino acid substitutions known to increase daptomycin MICs were detected in liaR (W73C) and liaS (T120A) in both E. faecium isolates. Each isolate also had amino acid substitutions (L38V, V173G, I432V) in the cls gene compared with E. faecium ATCC 700221, although these mutations in the cls gene have not yet been shown to confer daptomycin resistance alone [14]. The LRE finder did not identify any mutations in the 23S rRNA or in the optrA, cfr, cfr(B), and poxtA genes encoding linezolid resistance in enterococci.
Comparative genomics revealed that E. faecium UIC1 and E. faecium UIC2 were closely aligned, with 99.994% nucleotide similarity across an alignment of 2 913 393 base pairs. Furthermore, there were not any gaps or translocations between the 2 E. faecium sequences. The E. faecium UIC1 sequence, which harbored vanA, covered 92.2% and 91.5% of reference vanA plasmids pV24-5 and pS177, whereas E. faecium UIC2 only covered 63.5% and 53.3%, respectively.
Whole-genome sequencing of the E. cloacae complex revealed that the specific species within the complex was E. hormaechei and that the ampC-type β-lactamase blaACT-7 and the fosfomycin-modifying enzyme fosA were detected. Additional analysis of the ompC and ompF outer-membrane porin channels revealed premature stop codons at amino acid positions 82 and 252, respectively.
DISCUSSION
We report the case of a medically complex and vulnerable patient suffering from recurrent and persistent bacteremia due to E. faecium and E. cloacae complex secondary to a post-transplantation liver abscess. Both of these pathogens had unique resistance mechanisms and phenotypic susceptibility profiles, making selection of optimal antimicrobial therapy extremely challenging. In addition, limited robust clinical data exist to assist antimicrobial selection and treatment of serious endovascular infections due to infrequently encountered MDR pathogens. As such, pharmacodynamic analyses were performed to help guide antimicrobial therapy for this patient in the setting of conflicting genotypic–phenotypic resistance and rapidly changing within-host susceptibility profiles during the course of therapy. Time-kill analyses were able to identify the most bactericidal antimicrobial regimens, and when employed clinically, these regimens demonstrated success in clearing CRE bacteremia and keeping the VRE infection controlled for several months.
Our group and others have previously implemented pharmacodynamic analyses to guide antimicrobial therapy in patients [15–18]. However, to our knowledge, the enclosed example represents the first report that leverages this approach for either VRE or E. cloacae complex and adds to the accumulating evidence showing a potential benefit of pharmacodynamic analyses for difficult-to-treat infections. Further, the results of our time-kill analyses are in agreement with previous work demonstrating synergy between daptomycin and either β-lactams or aminoglycosides against VRE [19–22], including daptomycin-nonsusceptible strains, but to our knowledge this is the first modern study to examine relevant triple and quadruple antimicrobial combinations [23–26]. Finally, our work adds to the literature supporting the in vitro activity of ceftazidime-avibactam against CRE, even for isolates with MICs at or near the susceptibility breakpoint [27].
In addition to the PD analyses, retrospective whole-genome sequencing helped identify genetic resistance determinants for each of the patient’s isolates and explain the evolution of phenotypic resistance over time in response to antimicrobial therapy. After development of daptomycin resistance in the initial VRE isolate post-treatment, high sequence identity in conjunction with the patient’s clinical course strongly supports that E. faecium UIC2 evolved from E. faecium UIC1. Treatment of E. faecium UIC1 with linezolid likely selected for a linezolid-resistant subpopulation that was subsequently obtained from blood cultures as E. faecium UIC2. Unfortunately, whole-genome sequencing and use of the LRE finder did not identify the underlying mechanism for linezolid resistance in E. faecium UIC2. Linezolid-resistant E. faecium lacking all common resistance mechanisms have been previously reported in the literature [28–30] and suggest the presence of additional unidentified mechanisms. Conversely, E. faecium UIC2 reverted back to a vanA-negative, vancomycin-susceptible phenotype, likely due to the loss of a plasmid or transposon containing vanA and ant(6)-Ia, aph(3’)-III, and erm(B) from E. faecium UIC1 (Table 1), which have previously been shown to reside together on vanA plasmids in E. faecium [31, 32]. A previous report on the genomic dynamics of E. faecium also demonstrated that the loss of plasmids or transposons is associated with intrapatient variations in vancomycin resistance and virulence during infection in immunocompromised patients [33].
Unique to this case, intrahost changes in susceptibility occurred within the E. cloacae complex isolate in addition to the E. faecium. This rapid evolution of E. cloacae complex resistance from third-generation cephalosporin-resistant to CRE phenotype, in addition to the inability of commercially available genotypic and phenotypic tests to detect the presence of a carbapenemase in these isolates, contributed to the difficulty in selecting timely and effective antimicrobial therapy. Whole-genome sequencing was able to identify the species as E. hormaechei, 1 of 6 genetically related species within the E. cloacae complex [34]. Genome analysis also detected the presence of blaACT-7, an inducible plasmid-mediated serine class C β-lactamase [35], along with mutations in the outer membrane porins ompC and ompF. To our knowledge, this is the first report of a clinical case of infection with a blaACT-7-producing pathogen [36]. Overexpression of blaACT in conjunction with impaired function of outer membrane porins has previously been shown to induce carbapenem resistance [37] and may explain the reduced susceptibility observed to the carbapenems, ceftazidime-avibactam, and meropenem-vaborbactam in the E. cloacae complex in our patient. Taken together, the whole-genome sequencing data may enable our optimized antimicrobial regimens to be translated to future patients infected with organisms harboring similar resistance mechanisms. These data also highlight the importance of repeated phenotypic susceptibility testing in the clinical arena, especially in vulnerable patients with persistent infections for which source control is unachievable.
The case presented herein highlights the complexity of treating rapidly evolving, extremely drug-resistant pathogens, especially in critically ill and/or immunocompromised patients. Performing comprehensive genotypic and phenotypic susceptibility and time-kill analyses, along with knowledge of institution- and patient-specific factors, allowed us to use precision medicine to design a treatment regimen that maximized PK and PD. Although we felt that this approach helped optimize treatment for this patient, it is obviously not feasible or required for all patients, and cases for which these types of analyses are useful should be carefully selected. Importantly, this type of approach has not been definitively shown to improve patient outcomes, and in our case the patient ultimately succumbed to her illnesses despite optimization of antimicrobial therapy, likely due in part to an inability to achieve complete source control. Nevertheless, we provide a motivating example of the important role of genotypic, phenotypic, PK/PD, and clinical information coming together to optimize the treatment of a very challenging patient case. As bacterial resistance and the medical complexity of our patients continue to increase while the antimicrobial development pipeline dwindles, clinicians and researchers need to continue to work together to optimize patient care and achieve precision medicine.
Supplementary Data
Supplementary materials are available at Open Forum Infectious Diseases online. Consisting of data provided by the authors to benefit the reader, the posted materials are not copyedited and are the sole responsibility of the authors, so questions or comments should be addressed to the corresponding author.
Acknowledgments
Data availability. This Whole Genome Shotgun project has been deposited at GenBank under the accession numbers VSTA00000000 (E. faecium UIC1), VSTB00000000 (E. faecium UIC2), and VSTC00000000 (E. hormaechei).
Disclaimer. The content of this manuscript is solely the responsibility of the authors and does not necessarily represent the official views of the National Institutes of Health.
Ethical approval. This study was approved by the Loyola University Institutional Review Board with a waiver of consent granted.
Financial support. Z.P.B. was supported by the National Center for Advancing Translational Sciences (NCATS), National Institutes of Health, under Grant KL2TR002002. Bioinformatics analysis in the project described was performed in part by the UIC Research Informatics Core, supported in part by the NCATS through Grant UL1TR002003. There was no external funding for this study.
Potential conflicts of interest. E.W. serves on the speaker’s bureau for Melinta Therapeutics and Astellas Pharma and on the Advisory Board for GenMark Diagnostics and Shionogi. A.H. has received research funding from BioFire Diagnostics, LLC, and Beckman Coulter. All other authors certify no potential conflicts of interest. All authors have submitted the ICMJE Form for Disclosure of Potential Conflicts of Interest. Conflicts that the editors consider relevant to the content of the manuscript have been disclosed.
References
- phenotype
- vancomycin
- bacteremia
- combined modality therapy
- daptomycin
- drug resistance, microbial
- enterobacter cloacae
- enterococcus faecium
- genome
- genotype
- immunocompromised host
- liver transplantation
- infections
- pharmacodynamics
- pathogenic organism
- linezolid
- antimicrobials
- precision medicine
- carbapenem resistance
- whole genome sequencing
Comments