-
PDF
- Split View
-
Views
-
Cite
Cite
Chia-Lin Tseng, K Liang Zeng, Eric A Mellon, Scott G Soltys, Mark Ruschin, Angus Z Lau, Natalia S Lutsik, Rachel W Chan, Jay Detsky, James Stewart, Pejman J Maralani, Arjun Sahgal, Evolving concepts in margin strategies and adaptive radiotherapy for glioblastoma: A new future is on the horizon, Neuro-Oncology, Volume 26, Issue Supplement_1, March 2024, Pages S3–S16, https://doi.org/10.1093/neuonc/noad258
- Share Icon Share
Abstract
Chemoradiotherapy is the standard treatment after maximal safe resection for glioblastoma (GBM). Despite advances in molecular profiling, surgical techniques, and neuro-imaging, there have been no major breakthroughs in radiotherapy (RT) volumes in decades. Although the majority of recurrences occur within the original gross tumor volume (GTV), treatment of a clinical target volume (CTV) ranging from 1.5 to 3.0 cm beyond the GTV remains the standard of care. Over the past 15 years, the incorporation of standard and functional MRI sequences into the treatment workflow has become a routine practice with increasing adoption of MR simulators, and new integrated MR-Linac technologies allowing for daily pre-, intra- and post-treatment MR imaging. There is now unprecedented ability to understand the tumor dynamics and biology of GBM during RT, and safe CTV margin reduction is being investigated with the goal of improving the therapeutic ratio. The purpose of this review is to discuss margin strategies and the potential for adaptive RT for GBM, with a focus on the challenges and opportunities associated with both online and offline adaptive workflows. Lastly, opportunities to biologically guide adaptive RT using non-invasive imaging biomarkers and the potential to define appropriate volumes for dose modification will be discussed.
Background and Clinical Rationale
Fractionated radiotherapy (RT) concurrent with temozolomide (TMZ) is the standard upfront treatment for glioblastoma (GBM) after surgery. Despite advances in molecular profiling, surgical techniques, neuro-imaging and systemic therapies, there have been minimal changes in the RT component for decades. In particular, RT volumes do not reflect advancements in treatment planning, image guidance, and radiation delivery technologies; the delineation of the gross tumor volume (GTV) and clinical target volume (CTV) are still based on the postoperative MRI which represents a static snapshot of the disease and surrounding anatomy prior to treatment.
Radiotherapy Margins, Patterns of Failure, and Clinical Implications
In radiotherapy, GTV represents the gross demonstrable extent of malignant growth, which in GBM has been historically defined as the postoperative residual T1w enhancing disease and resection cavity. The CTV denotes the demonstrated malignant growth and volumes suspected to harbor subclinical/microscopic disease that must be encompassed within the therapeutic irradiation volume, which consists of an anatomical margin beyond that of the GTV. Lastly, the planning target volume (PTV) consists of a geometric margin around the CTV to account for variations in internal movement, patient motion, and setup uncertainties to ensure that the intended dose is delivered to the CTV. While the approach to delineation of the GTV is fairly consistent worldwide, despite international contouring recommendations defining the CTV1–3 (Table 1) there are large variations in margin strategies ranging from 0.5 to 3.0 cm of normal-appearing brain tissue to be included beyond the GTV. Some protocols (NCT00884741, NCT00304031) even stipulate the inclusion of all regions of T2-FLAIR hyperintensity, at least in the first phase of a two-phase approach, due to the uncertainty of what is tumor-associated edema versus infiltrative tumor.
Comparison of Radiotherapy Margins by Cooperative Groups and selected Studies
Society/study . | Planning . | GTV . | CTV . |
---|---|---|---|
MR-Linac International Consortium Research Group1 | Single phase | Resection cavity and residual enhancing disease on T1w MRI | 15 mm beyond GTV, respecting anatomic barriers to spread. Consensus recommendations on anatomic barriers are described. |
ESTRO-EANO (2023)3 | Single phase | Resection cavity + residual enhancing tumor on T1w MRI Optional: PET-based biologic tumor volume or FLAIR alteration in setting of non-enhancing molecular profile-based GBM | 15 mm expansion of GTV in 3D, reduced by anatomic barriers. Include non-contrast-enhancing FLAIR disease with variable/no margin. |
ESTRO-EANO (2016)102 | Single phase | Resection cavity + enhancing disease on T1w MRI | 20–30 mm beyond GTV, with optional inclusion of edema. |
NRG (2020)2 | Sequential boost (46 Gy then to 54 or 60 Gy) | GTV1: T2/FLAIR hyperintensity GTV2: resection cavity + T1w enhancement | CTV1: 20 mm beyond GTV1 and GTV2 with anatomic trimming. CTV2: 20 mm beyond GTV2 with anatomic trimming, with special consideration to the brainstem, optic chiasm and nerves. |
ABTC24 | Sequential boost (46 Gy then to 54 or 60 Gy) | GTV1: T1w enhancing and non-enhancing tumor volume (T2 or FLAIR) GTV2: T1w enhancing tumor volume | CTV1: 5 mm beyond GTV1 and GTV2 with anatomic trimming. CTV2: 5 mm beyond GTV2 with anatomic trimming. |
Azoulay et al.103 | Single phase | Resection cavity, residual enhancing tumor, nodular non-enhancing tumor | 5 mm beyond GTV, respecting anatomic barriers, no attempt to include peritumoral edema specifically. |
UNITED20 | Single phase MR-Linac online adapted | Resection cavity and contrast-enhancing residual on T1w MRI | Minimum 5 mm beyond GTV, with consideration to include abnormal T2/FLAIR hyperintensity, respecting anatomic barriers. |
Society/study . | Planning . | GTV . | CTV . |
---|---|---|---|
MR-Linac International Consortium Research Group1 | Single phase | Resection cavity and residual enhancing disease on T1w MRI | 15 mm beyond GTV, respecting anatomic barriers to spread. Consensus recommendations on anatomic barriers are described. |
ESTRO-EANO (2023)3 | Single phase | Resection cavity + residual enhancing tumor on T1w MRI Optional: PET-based biologic tumor volume or FLAIR alteration in setting of non-enhancing molecular profile-based GBM | 15 mm expansion of GTV in 3D, reduced by anatomic barriers. Include non-contrast-enhancing FLAIR disease with variable/no margin. |
ESTRO-EANO (2016)102 | Single phase | Resection cavity + enhancing disease on T1w MRI | 20–30 mm beyond GTV, with optional inclusion of edema. |
NRG (2020)2 | Sequential boost (46 Gy then to 54 or 60 Gy) | GTV1: T2/FLAIR hyperintensity GTV2: resection cavity + T1w enhancement | CTV1: 20 mm beyond GTV1 and GTV2 with anatomic trimming. CTV2: 20 mm beyond GTV2 with anatomic trimming, with special consideration to the brainstem, optic chiasm and nerves. |
ABTC24 | Sequential boost (46 Gy then to 54 or 60 Gy) | GTV1: T1w enhancing and non-enhancing tumor volume (T2 or FLAIR) GTV2: T1w enhancing tumor volume | CTV1: 5 mm beyond GTV1 and GTV2 with anatomic trimming. CTV2: 5 mm beyond GTV2 with anatomic trimming. |
Azoulay et al.103 | Single phase | Resection cavity, residual enhancing tumor, nodular non-enhancing tumor | 5 mm beyond GTV, respecting anatomic barriers, no attempt to include peritumoral edema specifically. |
UNITED20 | Single phase MR-Linac online adapted | Resection cavity and contrast-enhancing residual on T1w MRI | Minimum 5 mm beyond GTV, with consideration to include abnormal T2/FLAIR hyperintensity, respecting anatomic barriers. |
Comparison of Radiotherapy Margins by Cooperative Groups and selected Studies
Society/study . | Planning . | GTV . | CTV . |
---|---|---|---|
MR-Linac International Consortium Research Group1 | Single phase | Resection cavity and residual enhancing disease on T1w MRI | 15 mm beyond GTV, respecting anatomic barriers to spread. Consensus recommendations on anatomic barriers are described. |
ESTRO-EANO (2023)3 | Single phase | Resection cavity + residual enhancing tumor on T1w MRI Optional: PET-based biologic tumor volume or FLAIR alteration in setting of non-enhancing molecular profile-based GBM | 15 mm expansion of GTV in 3D, reduced by anatomic barriers. Include non-contrast-enhancing FLAIR disease with variable/no margin. |
ESTRO-EANO (2016)102 | Single phase | Resection cavity + enhancing disease on T1w MRI | 20–30 mm beyond GTV, with optional inclusion of edema. |
NRG (2020)2 | Sequential boost (46 Gy then to 54 or 60 Gy) | GTV1: T2/FLAIR hyperintensity GTV2: resection cavity + T1w enhancement | CTV1: 20 mm beyond GTV1 and GTV2 with anatomic trimming. CTV2: 20 mm beyond GTV2 with anatomic trimming, with special consideration to the brainstem, optic chiasm and nerves. |
ABTC24 | Sequential boost (46 Gy then to 54 or 60 Gy) | GTV1: T1w enhancing and non-enhancing tumor volume (T2 or FLAIR) GTV2: T1w enhancing tumor volume | CTV1: 5 mm beyond GTV1 and GTV2 with anatomic trimming. CTV2: 5 mm beyond GTV2 with anatomic trimming. |
Azoulay et al.103 | Single phase | Resection cavity, residual enhancing tumor, nodular non-enhancing tumor | 5 mm beyond GTV, respecting anatomic barriers, no attempt to include peritumoral edema specifically. |
UNITED20 | Single phase MR-Linac online adapted | Resection cavity and contrast-enhancing residual on T1w MRI | Minimum 5 mm beyond GTV, with consideration to include abnormal T2/FLAIR hyperintensity, respecting anatomic barriers. |
Society/study . | Planning . | GTV . | CTV . |
---|---|---|---|
MR-Linac International Consortium Research Group1 | Single phase | Resection cavity and residual enhancing disease on T1w MRI | 15 mm beyond GTV, respecting anatomic barriers to spread. Consensus recommendations on anatomic barriers are described. |
ESTRO-EANO (2023)3 | Single phase | Resection cavity + residual enhancing tumor on T1w MRI Optional: PET-based biologic tumor volume or FLAIR alteration in setting of non-enhancing molecular profile-based GBM | 15 mm expansion of GTV in 3D, reduced by anatomic barriers. Include non-contrast-enhancing FLAIR disease with variable/no margin. |
ESTRO-EANO (2016)102 | Single phase | Resection cavity + enhancing disease on T1w MRI | 20–30 mm beyond GTV, with optional inclusion of edema. |
NRG (2020)2 | Sequential boost (46 Gy then to 54 or 60 Gy) | GTV1: T2/FLAIR hyperintensity GTV2: resection cavity + T1w enhancement | CTV1: 20 mm beyond GTV1 and GTV2 with anatomic trimming. CTV2: 20 mm beyond GTV2 with anatomic trimming, with special consideration to the brainstem, optic chiasm and nerves. |
ABTC24 | Sequential boost (46 Gy then to 54 or 60 Gy) | GTV1: T1w enhancing and non-enhancing tumor volume (T2 or FLAIR) GTV2: T1w enhancing tumor volume | CTV1: 5 mm beyond GTV1 and GTV2 with anatomic trimming. CTV2: 5 mm beyond GTV2 with anatomic trimming. |
Azoulay et al.103 | Single phase | Resection cavity, residual enhancing tumor, nodular non-enhancing tumor | 5 mm beyond GTV, respecting anatomic barriers, no attempt to include peritumoral edema specifically. |
UNITED20 | Single phase MR-Linac online adapted | Resection cavity and contrast-enhancing residual on T1w MRI | Minimum 5 mm beyond GTV, with consideration to include abnormal T2/FLAIR hyperintensity, respecting anatomic barriers. |
At the level of the individual radiation oncologist, the challenge of differentiating edema versus infiltrative tumor, and inconsistent anatomic cropping to limit the CTV margin from boundaries such as the dura, likely contribute to contouring variation. However, despite the variations in CTV definition and nuances in application of various MRI sequences, the proportion of central or in-field failures is similar with the majority of failures occurring in the GTV (Table 2). For example, Paulsson et al. evaluated CTV margins of 5-, 10-, and 15–20 mm in 161 patients with GBM, and found that there was no statistically significant difference in failure rates within the 60 Gy volume and 46 Gy volume (2-phases), and that the overall rate of in-field failure was approximately 80%.4 This is consistent with a more recent study applying a limited 5 mm CTV margin and treatment with 5 stereotactic fractions concurrent with TMZ, where 11% had marginal progression within the 5–20 mm margin of which only 1 patient was identified who could have benefited from a larger CTV.5
Selected Studies on Patterns of Progression Following Chemoradiotherapy for Glioblastoma
Reference . | CTV margin* . | % In-field . | Definition of patterns of failure . | Comments . |
---|---|---|---|---|
Paulsson et al.4 | 5 mm 10 mm 15–20 mm | 79% 77% 87% | In-field = failure within the 60 Gy isodose line | N = 161; PTV not specified; No statistically significant difference in in-field failures between different CTV margins |
Mendoza et al.5 | 5 mm (total treatment margin) | 63% | In-field = recurrent tumor within or contiguous with the 5 mm margin Marginal = recurrent tumor between 5 and 20 mm margin | N = 30; PTV = 0 mm Three patients (11%) with marginal failure; only 1 patient (4%) may have dosimetrically benefited from a conventional 20 mm margin |
McDonald et al.104 | Phase I: 5 mm (on T2-FLAIR) Phase II: 5 mm (on GTV) | 93% | In-field or central = 81–100% of recurrent tumor volume within the 60 Gy isodose line Marginal = 20–80% of recurrent tumor volume within the 60 Gy isodose line Distant = <20% of recurrent tumor volume within the 60 Gy isodose line | N = 62; PTV = 3–5 mm Two patients (5%) had marginal failure, and 1 patient (2%) had distant failure |
Brandes et al.105 | 20–30 mm | 72% | In-field = ≥ 80% of the tumor recurrence resided within the 95% prescription isodose surface Marginal = 20–80% of the recurrent tumor was within the 95% prescription isodose surface | N = 95; PTV = up to 5 mm Five patients (6.3%) had marginal failure, and 17 patients (21.5%) had distant failure |
Gebhardt et al.24 | Phase I: 5 mm (on T2-FLAIR) Phase II: 5 mm (on GTV) | 81% | In-field = > 80% of the recurrent tumor was within the 95% isodose line Marginal = > 20% but ≤ 80% of the recurrent tumor was within the 95% isodose line | N = 95; PTV = 5 mm 14 patients (15%) had only distant failures |
Milano et al.106 | Phase I: 20 mm (on T2-FLAIR) Phase II: 20–25 mm (on GTV) | 80% (central) | Central = growth of original tumor or development of tumor(s) arising from surgical cavity In-field = new tumor entirely within the 95% isodose line Marginal = new lesion crosses the 95% isodose line Distant = new lesion outside of the 95% isodose line | N = 54; PTV not specified; 33% developed new in-field recurrence, and 20% developed distant failures |
Petrecca et al.107 | 20 mm | 85% | Resection margin = located at or in continuity with resection cavity Distant = all other recurrent locations | N = 20; PTV = 5 mm Two patients (10%) developed distant only recurrence |
Sherriff et al.108 | 15–20 mm | 77% (central) | Central = progression of residual tumor enhancement, within 2 cm of the original mass Distant = relapse > 4 cm from original tumor edge | N = 71; PTV = 5 mm 16 (22%) had distant failure |
Kumar et al.16 | RTOG approach: Phase 1: 20 mm (on GTV + T2-FLAIR) Phase 2: 25 mm (on GTV) MDACC approach: Phase 1: 20 mm (on GTV) Phase 2: 5 mm (on GTV) | 88% (central or in-field) 87% (central or in-field) | Central = >95% of recurrent tumor volume inside 95% isodose volume In-field = >95% of recurrent tumor volume between 80% and 95% isodose volume Marginal = >95% of recurrent tumor volume between 20% and 80% isodose volumes Distant = >95% of recurrent tumor volume beyond 20% isodose volume | Total N = 50 (both arms); PTV = 5 mm Two patients (12.5%) with marginal failure, no distant failure One patient (6.25%) with marginal failure, and 1 patient (6.25%) with distant failure |
Tu et al.109 | Phase 1: 20 mm Phase 2: 5 mm | 69% | In-field = >80% of recurrent tumor covered by 95% isodose line of boost plan (phase 2) Marginal = 20–80% of recurrent tumor inside 95% isodose line of boost plan (phase 2) Distant = <20% of recurrent tumor inside 95% isodose line of boost plan (phase 2) | N = 68; PTV = 5 mm 12 patients (17.7%) had distant failure; all recurrences were within 2 cm of original GTV, and 94.8% within 1 cm of original GTV |
Zheng et al.110 | Phase 1: 20 mm Phase 2: 10 mm | 84% (central or in-field) | Central = >95% of recurrent tumor volume within the 60 Gy isodose line In-field = 80–95% of recurrent tumor volume within the 60 Gy isodose line Marginal = 20–80% of recurrent tumor volume within the 60 Gy isodose line Distant = <20% of recurrent tumor volume within the 60 Gy isodose line | N = 55; PTV = 3 mm One patient (1.8%) had marginal recurrence and 11 patients (20.0%) had distant recurrences |
Reference . | CTV margin* . | % In-field . | Definition of patterns of failure . | Comments . |
---|---|---|---|---|
Paulsson et al.4 | 5 mm 10 mm 15–20 mm | 79% 77% 87% | In-field = failure within the 60 Gy isodose line | N = 161; PTV not specified; No statistically significant difference in in-field failures between different CTV margins |
Mendoza et al.5 | 5 mm (total treatment margin) | 63% | In-field = recurrent tumor within or contiguous with the 5 mm margin Marginal = recurrent tumor between 5 and 20 mm margin | N = 30; PTV = 0 mm Three patients (11%) with marginal failure; only 1 patient (4%) may have dosimetrically benefited from a conventional 20 mm margin |
McDonald et al.104 | Phase I: 5 mm (on T2-FLAIR) Phase II: 5 mm (on GTV) | 93% | In-field or central = 81–100% of recurrent tumor volume within the 60 Gy isodose line Marginal = 20–80% of recurrent tumor volume within the 60 Gy isodose line Distant = <20% of recurrent tumor volume within the 60 Gy isodose line | N = 62; PTV = 3–5 mm Two patients (5%) had marginal failure, and 1 patient (2%) had distant failure |
Brandes et al.105 | 20–30 mm | 72% | In-field = ≥ 80% of the tumor recurrence resided within the 95% prescription isodose surface Marginal = 20–80% of the recurrent tumor was within the 95% prescription isodose surface | N = 95; PTV = up to 5 mm Five patients (6.3%) had marginal failure, and 17 patients (21.5%) had distant failure |
Gebhardt et al.24 | Phase I: 5 mm (on T2-FLAIR) Phase II: 5 mm (on GTV) | 81% | In-field = > 80% of the recurrent tumor was within the 95% isodose line Marginal = > 20% but ≤ 80% of the recurrent tumor was within the 95% isodose line | N = 95; PTV = 5 mm 14 patients (15%) had only distant failures |
Milano et al.106 | Phase I: 20 mm (on T2-FLAIR) Phase II: 20–25 mm (on GTV) | 80% (central) | Central = growth of original tumor or development of tumor(s) arising from surgical cavity In-field = new tumor entirely within the 95% isodose line Marginal = new lesion crosses the 95% isodose line Distant = new lesion outside of the 95% isodose line | N = 54; PTV not specified; 33% developed new in-field recurrence, and 20% developed distant failures |
Petrecca et al.107 | 20 mm | 85% | Resection margin = located at or in continuity with resection cavity Distant = all other recurrent locations | N = 20; PTV = 5 mm Two patients (10%) developed distant only recurrence |
Sherriff et al.108 | 15–20 mm | 77% (central) | Central = progression of residual tumor enhancement, within 2 cm of the original mass Distant = relapse > 4 cm from original tumor edge | N = 71; PTV = 5 mm 16 (22%) had distant failure |
Kumar et al.16 | RTOG approach: Phase 1: 20 mm (on GTV + T2-FLAIR) Phase 2: 25 mm (on GTV) MDACC approach: Phase 1: 20 mm (on GTV) Phase 2: 5 mm (on GTV) | 88% (central or in-field) 87% (central or in-field) | Central = >95% of recurrent tumor volume inside 95% isodose volume In-field = >95% of recurrent tumor volume between 80% and 95% isodose volume Marginal = >95% of recurrent tumor volume between 20% and 80% isodose volumes Distant = >95% of recurrent tumor volume beyond 20% isodose volume | Total N = 50 (both arms); PTV = 5 mm Two patients (12.5%) with marginal failure, no distant failure One patient (6.25%) with marginal failure, and 1 patient (6.25%) with distant failure |
Tu et al.109 | Phase 1: 20 mm Phase 2: 5 mm | 69% | In-field = >80% of recurrent tumor covered by 95% isodose line of boost plan (phase 2) Marginal = 20–80% of recurrent tumor inside 95% isodose line of boost plan (phase 2) Distant = <20% of recurrent tumor inside 95% isodose line of boost plan (phase 2) | N = 68; PTV = 5 mm 12 patients (17.7%) had distant failure; all recurrences were within 2 cm of original GTV, and 94.8% within 1 cm of original GTV |
Zheng et al.110 | Phase 1: 20 mm Phase 2: 10 mm | 84% (central or in-field) | Central = >95% of recurrent tumor volume within the 60 Gy isodose line In-field = 80–95% of recurrent tumor volume within the 60 Gy isodose line Marginal = 20–80% of recurrent tumor volume within the 60 Gy isodose line Distant = <20% of recurrent tumor volume within the 60 Gy isodose line | N = 55; PTV = 3 mm One patient (1.8%) had marginal recurrence and 11 patients (20.0%) had distant recurrences |
*On GTV unless otherwise specified.
Selected Studies on Patterns of Progression Following Chemoradiotherapy for Glioblastoma
Reference . | CTV margin* . | % In-field . | Definition of patterns of failure . | Comments . |
---|---|---|---|---|
Paulsson et al.4 | 5 mm 10 mm 15–20 mm | 79% 77% 87% | In-field = failure within the 60 Gy isodose line | N = 161; PTV not specified; No statistically significant difference in in-field failures between different CTV margins |
Mendoza et al.5 | 5 mm (total treatment margin) | 63% | In-field = recurrent tumor within or contiguous with the 5 mm margin Marginal = recurrent tumor between 5 and 20 mm margin | N = 30; PTV = 0 mm Three patients (11%) with marginal failure; only 1 patient (4%) may have dosimetrically benefited from a conventional 20 mm margin |
McDonald et al.104 | Phase I: 5 mm (on T2-FLAIR) Phase II: 5 mm (on GTV) | 93% | In-field or central = 81–100% of recurrent tumor volume within the 60 Gy isodose line Marginal = 20–80% of recurrent tumor volume within the 60 Gy isodose line Distant = <20% of recurrent tumor volume within the 60 Gy isodose line | N = 62; PTV = 3–5 mm Two patients (5%) had marginal failure, and 1 patient (2%) had distant failure |
Brandes et al.105 | 20–30 mm | 72% | In-field = ≥ 80% of the tumor recurrence resided within the 95% prescription isodose surface Marginal = 20–80% of the recurrent tumor was within the 95% prescription isodose surface | N = 95; PTV = up to 5 mm Five patients (6.3%) had marginal failure, and 17 patients (21.5%) had distant failure |
Gebhardt et al.24 | Phase I: 5 mm (on T2-FLAIR) Phase II: 5 mm (on GTV) | 81% | In-field = > 80% of the recurrent tumor was within the 95% isodose line Marginal = > 20% but ≤ 80% of the recurrent tumor was within the 95% isodose line | N = 95; PTV = 5 mm 14 patients (15%) had only distant failures |
Milano et al.106 | Phase I: 20 mm (on T2-FLAIR) Phase II: 20–25 mm (on GTV) | 80% (central) | Central = growth of original tumor or development of tumor(s) arising from surgical cavity In-field = new tumor entirely within the 95% isodose line Marginal = new lesion crosses the 95% isodose line Distant = new lesion outside of the 95% isodose line | N = 54; PTV not specified; 33% developed new in-field recurrence, and 20% developed distant failures |
Petrecca et al.107 | 20 mm | 85% | Resection margin = located at or in continuity with resection cavity Distant = all other recurrent locations | N = 20; PTV = 5 mm Two patients (10%) developed distant only recurrence |
Sherriff et al.108 | 15–20 mm | 77% (central) | Central = progression of residual tumor enhancement, within 2 cm of the original mass Distant = relapse > 4 cm from original tumor edge | N = 71; PTV = 5 mm 16 (22%) had distant failure |
Kumar et al.16 | RTOG approach: Phase 1: 20 mm (on GTV + T2-FLAIR) Phase 2: 25 mm (on GTV) MDACC approach: Phase 1: 20 mm (on GTV) Phase 2: 5 mm (on GTV) | 88% (central or in-field) 87% (central or in-field) | Central = >95% of recurrent tumor volume inside 95% isodose volume In-field = >95% of recurrent tumor volume between 80% and 95% isodose volume Marginal = >95% of recurrent tumor volume between 20% and 80% isodose volumes Distant = >95% of recurrent tumor volume beyond 20% isodose volume | Total N = 50 (both arms); PTV = 5 mm Two patients (12.5%) with marginal failure, no distant failure One patient (6.25%) with marginal failure, and 1 patient (6.25%) with distant failure |
Tu et al.109 | Phase 1: 20 mm Phase 2: 5 mm | 69% | In-field = >80% of recurrent tumor covered by 95% isodose line of boost plan (phase 2) Marginal = 20–80% of recurrent tumor inside 95% isodose line of boost plan (phase 2) Distant = <20% of recurrent tumor inside 95% isodose line of boost plan (phase 2) | N = 68; PTV = 5 mm 12 patients (17.7%) had distant failure; all recurrences were within 2 cm of original GTV, and 94.8% within 1 cm of original GTV |
Zheng et al.110 | Phase 1: 20 mm Phase 2: 10 mm | 84% (central or in-field) | Central = >95% of recurrent tumor volume within the 60 Gy isodose line In-field = 80–95% of recurrent tumor volume within the 60 Gy isodose line Marginal = 20–80% of recurrent tumor volume within the 60 Gy isodose line Distant = <20% of recurrent tumor volume within the 60 Gy isodose line | N = 55; PTV = 3 mm One patient (1.8%) had marginal recurrence and 11 patients (20.0%) had distant recurrences |
Reference . | CTV margin* . | % In-field . | Definition of patterns of failure . | Comments . |
---|---|---|---|---|
Paulsson et al.4 | 5 mm 10 mm 15–20 mm | 79% 77% 87% | In-field = failure within the 60 Gy isodose line | N = 161; PTV not specified; No statistically significant difference in in-field failures between different CTV margins |
Mendoza et al.5 | 5 mm (total treatment margin) | 63% | In-field = recurrent tumor within or contiguous with the 5 mm margin Marginal = recurrent tumor between 5 and 20 mm margin | N = 30; PTV = 0 mm Three patients (11%) with marginal failure; only 1 patient (4%) may have dosimetrically benefited from a conventional 20 mm margin |
McDonald et al.104 | Phase I: 5 mm (on T2-FLAIR) Phase II: 5 mm (on GTV) | 93% | In-field or central = 81–100% of recurrent tumor volume within the 60 Gy isodose line Marginal = 20–80% of recurrent tumor volume within the 60 Gy isodose line Distant = <20% of recurrent tumor volume within the 60 Gy isodose line | N = 62; PTV = 3–5 mm Two patients (5%) had marginal failure, and 1 patient (2%) had distant failure |
Brandes et al.105 | 20–30 mm | 72% | In-field = ≥ 80% of the tumor recurrence resided within the 95% prescription isodose surface Marginal = 20–80% of the recurrent tumor was within the 95% prescription isodose surface | N = 95; PTV = up to 5 mm Five patients (6.3%) had marginal failure, and 17 patients (21.5%) had distant failure |
Gebhardt et al.24 | Phase I: 5 mm (on T2-FLAIR) Phase II: 5 mm (on GTV) | 81% | In-field = > 80% of the recurrent tumor was within the 95% isodose line Marginal = > 20% but ≤ 80% of the recurrent tumor was within the 95% isodose line | N = 95; PTV = 5 mm 14 patients (15%) had only distant failures |
Milano et al.106 | Phase I: 20 mm (on T2-FLAIR) Phase II: 20–25 mm (on GTV) | 80% (central) | Central = growth of original tumor or development of tumor(s) arising from surgical cavity In-field = new tumor entirely within the 95% isodose line Marginal = new lesion crosses the 95% isodose line Distant = new lesion outside of the 95% isodose line | N = 54; PTV not specified; 33% developed new in-field recurrence, and 20% developed distant failures |
Petrecca et al.107 | 20 mm | 85% | Resection margin = located at or in continuity with resection cavity Distant = all other recurrent locations | N = 20; PTV = 5 mm Two patients (10%) developed distant only recurrence |
Sherriff et al.108 | 15–20 mm | 77% (central) | Central = progression of residual tumor enhancement, within 2 cm of the original mass Distant = relapse > 4 cm from original tumor edge | N = 71; PTV = 5 mm 16 (22%) had distant failure |
Kumar et al.16 | RTOG approach: Phase 1: 20 mm (on GTV + T2-FLAIR) Phase 2: 25 mm (on GTV) MDACC approach: Phase 1: 20 mm (on GTV) Phase 2: 5 mm (on GTV) | 88% (central or in-field) 87% (central or in-field) | Central = >95% of recurrent tumor volume inside 95% isodose volume In-field = >95% of recurrent tumor volume between 80% and 95% isodose volume Marginal = >95% of recurrent tumor volume between 20% and 80% isodose volumes Distant = >95% of recurrent tumor volume beyond 20% isodose volume | Total N = 50 (both arms); PTV = 5 mm Two patients (12.5%) with marginal failure, no distant failure One patient (6.25%) with marginal failure, and 1 patient (6.25%) with distant failure |
Tu et al.109 | Phase 1: 20 mm Phase 2: 5 mm | 69% | In-field = >80% of recurrent tumor covered by 95% isodose line of boost plan (phase 2) Marginal = 20–80% of recurrent tumor inside 95% isodose line of boost plan (phase 2) Distant = <20% of recurrent tumor inside 95% isodose line of boost plan (phase 2) | N = 68; PTV = 5 mm 12 patients (17.7%) had distant failure; all recurrences were within 2 cm of original GTV, and 94.8% within 1 cm of original GTV |
Zheng et al.110 | Phase 1: 20 mm Phase 2: 10 mm | 84% (central or in-field) | Central = >95% of recurrent tumor volume within the 60 Gy isodose line In-field = 80–95% of recurrent tumor volume within the 60 Gy isodose line Marginal = 20–80% of recurrent tumor volume within the 60 Gy isodose line Distant = <20% of recurrent tumor volume within the 60 Gy isodose line | N = 55; PTV = 3 mm One patient (1.8%) had marginal recurrence and 11 patients (20.0%) had distant recurrences |
*On GTV unless otherwise specified.
The volume of brain irradiated in GBM, which is primarily determined by the CTV, may have important clinical toxicity implications. For example, hippocampal dosimetry has been shown to impact neurocognitive function in patients with low- and high-grade glioma treated with RT.6–8 An interesting analysis of a randomized phase II trial evaluating proton vs. photon therapy for patients with GBM, showed an association of radiation-induced grade 3 + lymphopenia with whole-brain mean dose and the brain volumes receiving 5–40 Gy.9 However, no impact of proton therapy was shown with respect to cognitive preservation rates.10 It is worth noting that the treatment volume for GBM does not purposely include any active bone marrow although the glymphatic pathway, first described in 2012,11 may in part mediate the observed immune system changes from radiation. How this may impact patient outcomes or systemic therapeutic options such as immunotherapy is a topic of future research, although to date results from immunotherapy for GBM have been disappointing.12
Another important clinical consideration when modifying, particularly when reducing, the CTV margin is the potential to miss the target, and if such strategies are to be considered then more rigorous serial imaging during a course of treatment becomes more compelling to ensure that the GTV is not changing. This is a challenge and a departure from standard practice where imaging of the brain occurs at the time of planning prior to RT, and subsequently at 4–6 weeks post completion of RT to assess treatment response. One of the first studies to demonstrate the value of repeat imaging during a course of RT evaluated the utility of a sequential boost approach. Manon et al. reported that 80% of the boost target volumes (12 of 15 cases) would have resulted in a geographic miss had they been contoured using the initial planning MRI rather than with a new MRI taken mid treatment.13 More recently, Stewart et al. in a large prospective MR imaging study assessed tumor dynamics during a standard 6-week course of RT, found GTV migration distances (maximum linear displacements of the GTV when compared to planning images) greater than 5 mm in 46% of patients at fraction 10, 50% of patients at fraction 20, and 52% of patients at 1-month after treatment completion, respectively.14 Similarly, the same study also found migration distances greater than 5 mm in the CTV, defined by a 1.5 cm isotropic expansion beyond GTV, respecting anatomic barriers, in 54% of patients at fraction 10, 58% of patients at fraction 20, and 57% of patients at 1-month after treatment completion. A second study by Bernchou et al. confirmed these findings, observing large variations in GTV extent that could occur early in the treatment course.15 Evolution of post-surgical imaging changes is complex and may be associated with ischemic changes, presence of edema, steroid administration, cavity dynamics, and tumor growth. Nevertheless, these data highlight that the target does not remain static during a course of treatment as previously thought. Collectively, the data support that there are indeed clinically meaningful dynamics encompassing both volumetric and morphological changes and, we surmise, opportunities to refine RT practice with modern techniques.
Reduced Margin RT With Adaptation Based on Anatomic Changes During RT
By taking advantage of modern RT technologies, potential reductions in both CTV and planning target volume (PTV) margins can be realized. Furthermore, considerations for routine treatment plan adaptation may allow for reductions in the normal brain irradiated which in turn may improve the therapeutic ratio. This hypothesis is supported by Kumar et al. who led a phase II randomized trial of 50 patients comparing 2 CTV margin strategies both delivered in a sequential boost fashion.16 In arm 1, patients were treated per RTOG protocol, with the phase I CTV encompassing a 2 cm margin beyond both GTV and FLAIR hyperintense volume, and the phase II CTV encompassing a 2.5 cm margin beyond the GTV. In arm 2, patients were treated with a more contracted phase I CTV of 2 cm margin beyond the GTV without inclusion of all peritumoral edema, and a phase II CTV of only 0.5 cm margin beyond the GTV. Arm 2 which used a more limited margin approach showed significant improvements in progression-free-survival, overall survival, and quality-of-life domains including global functioning, physical, emotional, social, and role functioning as compared to those who were planned with larger CTV margins. Although the results of Kumar et al.’s study are promising, the small sample size and unavailability of molecular-based diagnostic information are acknowledged. Paulsson et al. reviewed 161 patients with GBM treated with CTV margins ranging from 5 to 20 mm using a shrinking field technique.4 In patients treated with 5-, 10-, and 15- to 20-mm CTV, 79%, 77%, and 86% experienced failures in the 60 Gy volume, respectively. No statistically significant differences were observed between patients treated with the varying margins with regards to 60 Gy failure (P = .76), 46 Gy failure (P = .51), or marginal failure (P = .73). Overall, 80% of patients receiving TMZ failed within the 60 Gy volume. Although the historical concern was increasing the risk of marginal failure with smaller CTV margins, these studies suggest that recurrence patterns were not different and consistent with other studies evaluating patterns of failure as summarized in Table 2.
Image guidance, at present, is based on cone-beam CT (CBCT) images on a conventional linear accelerator (Linac), which must rely on bony alignment as the soft tissue cannot be reliably visualized. This technology has resulted in a reduction of the PTV from historically 5–10 mm to 3 mm, with the latter recommended in a recent guideline by ESTRO-EANO.3,17 The PTV is designed by definition to ensure adequate dose delivery to the CTV, however, accurate delineation of the CTV based on appropriate soft tissue visualization is necessary to achieve the goals of treatment.
Serial imaging of the tumor with online or offline MRI during RT helps to ensure the safety of a CTV margin reduction strategy due to superior soft tissue visualization and alignment of treatment fields to tumor. The feasibility of using an integrated or hybrid MR-Linac to treat high grade glioma with onboard MR imaging has been reported with acceptable treatment times. The reports also support the clinical utility of this technology in identifying patients requiring re-planning due to significant tumor- and/or surrounding anatomy-related changes beyond originally planned margins.18,19
Clinical trials are ongoing to explore the use of online adaptive approaches with reduced CTV with MR-Linac technology in GBM.20–22 The UNITED trial (NCT04726397) has completed accrual and represents the first prospective study on an MR-Linac examining a treatment strategy utilizing a reduced CTV margin of 5 mm with optional inclusion of adjacent FLAIR signal. On the study, a weekly gadolinium enhanced T1w MRI was acquired, and the GTV and CTV were re-contoured online with re-optimized adaptive plans. The primary endpoint was patterns of failure and the results are anticipated to advance knowledge and inform the direction with respect to CTV margin reduction strategies. The optional inclusion of additional FLAIR signal at the discretion of the treating physician, however, may limit the analysis with respect to the recurrence pattern. Overall, the strategy to reduce the volume irradiated is hypothesized to translate into a more favorable toxicity profile for dose escalation strategies which are currently being investigated in the elderly population.22
RT for GBM should reflect modern advancements in treatment delivery and imaging, with strong considerations for appropriate CTV margins that reflect patterns of failure while balancing the avoidance of normal tissues with the risk of marginal failure. The integration of MRI into the treatment workflow23, and ability to routinely perform adaptive RT when treatment volumes change, may represent a new era in RT design aimed at improving toxicity profiles, clinical care and ultimately survival outcomes. The purpose of this review is to discuss adaptive RT strategies for GBM, the requirements and technical elements of both online and offline adaptation and future directions with advanced imaging to improve outcomes in patients.
Adaptation Strategies and Technical Elements
General Requirements for All Strategies
Adaptive RT strategies are broadly classified into 2 categories: (1) online occurring with the patient in treatment position, and (2) offline occurring with the patient not on the treatment couch. Offline treatment adaptation can be triggered by anatomic changes such as: those observed by verification imaging including the development of hemorrhage which can be seen on CBCT, weight gain/loss leading to a change in separation and/or the ill-fitting of the mask, through the incorporation of a pre-specified MRI taken during a course of treatment which is then used for re-planning, or clinical changes in the patient prompting diagnostic imaging confirming a need for adaptation which is then incorporated into the treatment plan. Online imaging is now a reality with the integration of an MRI with a Linac (MR-Linac) enabling daily imaging and tracking of tumor dynamics and FLAIR changes. The general workflow and adaptive elements in adaptive RT strategies are shared regardless of the system used. As shown in Figure 1, there are multiple pathways to achieve adaptive RT, including online (with an MR-Linac) and/or offline (eg with a conventional CBCT-based Linac) workflows, MR-only or combined CT- and MR-based planning, and using fixed or continuous time points for adaptation. The subsequent sections highlight the commonalities and important differences between online and offline approaches.
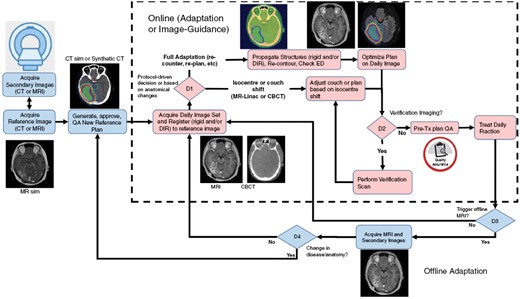
High level workflow diagram outlining online and offline adaptive elements for adaptive brain radiotherapy. Reference imaging can be CT-based with a co-registered MRI, or MR-based with either a co-registered CT for bulk density assignment or a method to convert the MRI directly to synthetic CT. In the online adaptation section (inside the dashed box), an onboard imager (either MRI or CBCT based) is used to acquire daily image sets, which are used to either shift the isocentre to the patient position (or vice versa) or to re-contour and re-optimize the treatment plan based on changes in anatomy via decision D1. The clinic may also wish to perform a verification scan prior to treatment (D2), although this is not a requirement. The trigger for offline adaptation (D3) may be either at a fixed time point (example after fraction 19 out of 30 fractions) or triggered by an observation at the treatment unit (eg swelling, mask not fitting, etc.). If an offline MRI is acquired and the anatomy/disease has changed then a decision (D4) to use that scan to adapt the plan to the current anatomy and generate a new reference plan could be made, which would feed back into the online workflow. The figure is primarily illustrative of the various workflows that may exist and is not meant to prescriptive.
Time and Resource Considerations
Beyond standard RT requirements in treatment of GBM, additional patient and resource factors should be considered with adaptive approaches. Due to additional time required for imaging, especially with online workflows, claustrophobia and the ability to lie flat for extended periods are considerations. Treatment on an MR-Linac requires longer allotted time slots compared to a conventional Linac, even when only accounting for rigid translational shifts.18 A full adaptive workflow accounting for both positional changes and deformations in targets and organs-at-risk (OARs) requires additional time and will be addressed in later sections.
Offline adaptive approaches, where either imaging or re-planning or both are repeated either before or after delivery of a radiotherapy fraction, can partially negate the impact of these potential sources of discomfort for patients; however, this necessitates an additional visit for the patient and the optimal time point for re-planning require further study as daily offline imaging is not feasible. Prospective imaging studies support that the majority of volumetric and morphologic changes in the GTV occur before fraction 20,14,15 suggesting that in a standard 6-week course of RT, re-simulation (CT and MRI) at fraction 19 of treatment with intent to deliver fractions 24–30 on a new plan may be a practical approach. This is also consistent with the time frame used in 2-phase sequential treatment paradigms.2,24 Further work is needed to examine factors that can identify patients who may need re-planning sooner or not at all given the significant resources required in this process. For example, tumor molecular features such as MGMT methylation status may have implications in morphology and migration distance during a course of chemoradiotherapy (Figure 2) and may guide patient selection, although these data are still preliminary and requires validation.25
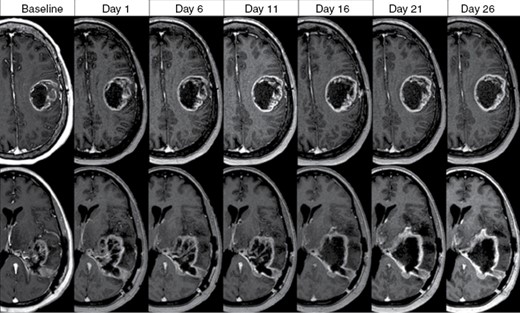
Tumor dynamics in 2 patients with MGMT-promotor unmethylated glioblastoma treated with concurrent chemoradiotherapy (60 Gy in 30 fractions) with contrast-enhanced T1w MRI acquired weekly demonstrating progressive increase in enhancing tumor/cavity during the treatment course.
General Technical Elements
MRI.—For adaptive brain RT, having high geometric-fidelity, high quality MR images for soft tissue and disease visualization and localization is of utmost importance. Geometric accuracy depends on multiple factors including B0 magnetic field inhomogeneity and gradient nonlinearity at the system level, and chemical shift artifacts and magnetic susceptibility at the patient/object level.26,27 Having a uniform B0 field, along with an understanding of the magnitude of gradient nonlinearities and how they can be corrected for with appropriate pulse sequence modifications and/or reconstruction methods is recommended, along with an understanding of the tradeoffs such as bandwidth versus signal-to-noise ratio, and the effects of using fast imaging sequences such as echo-planar imaging.26 Establishing an MRI QA program that characterizes and monitors distortion as well as a team of MRI experts is recommended for MR simulation, as well as the on-board integrated MRI used for image guidance.28–31
Gadolinium.—The use of an exogenous contrast agent such as gadolinium-based agents are necessary to directly visualize brain tumors with high contrast. Regardless of the workflow, contrast will likely be used during MR simulation to define the target at initiation of treatment. For plan adaptation, whether offline or online, the optimal frequency and timing of contrast-injection is unknown. Longitudinal use of gadolinium has been reported in MR-guided radiotherapy, although not specific to the brain,32 but since gadolinium carries with it a finite risk of toxicity, the frequency of use should be kept to the minimum level needed to achieve the clinical intent.33,34 Intravoxel incoherent motion (IVIM) is a method to measure microscopic water motion which can provide quantitative metrics for diffusion, reflective of structural changes, and perfusion, and reflective of movement of blood in microvasculature.35 This method has been shown to be useful in differentiating high grade glioma from low grade glioma, and in predicting treatment outcomes during chemoradiation for GBM patients,36,37 and may serve as a possible alternative or supplement to gadolinium contrast.
Pseudo/synthetic CT generation.—For MR-based workflows, whether online or offline, dose calculation can only be performed with electron density information. To facilitate this, some MRI vendors have implemented sequences (eg, MRCAT, Philips) that generate a synthetic CT directly from an MRI sequence.38 Most other methods include merging information from a CT and MR scan of the same patient together to generate a synthetic CT. For example, in the Elekta Unity MR-Linac workflow, contours made on the patient CT are used to derive bulk (or mean) electron density values that are then propagated to the MR of the same patient via co-registration for bulk density assignment.26,39 Subsequently, in the online adaptive setting, the bulk density assignment can be propagated forward to each new daily image set the same way that target/OAR contours are propagated. On the ViewRay MRIdian system, an adaptive deformable image registration workflow is utilized to map the simulation CT to the simulation MRI and subsequently daily MRIs for electron density information.40 A key step in the MR-based workflow is to ensure that the dosimetric accuracy of plans calculated on MR images is clinically acceptable relative to how the same plans are computed on calibrated CT scans.
PTV margins.—The magnitude of the PTV margin for brain depends on several sources of uncertainty including: machine-related uncertainties, motion-related uncertainties, and localization related uncertainties.41 With respect to the machine, an understanding of the spatial integrity of the multileaf collimator (MLC) positions and the accuracy of the MR-to-MV isocenter calibration are two examples of geometric uncertainties that could affect targeting accuracy. Patient motion within the mask is another source of uncertainty. Localization and setup uncertainties include: the ability (or lack thereof) to account for rotational setup errors, the geometric fidelity of the onboard imaging system, the accuracy of image co-registration and the accuracy of the corrective strategy for accounting for setup errors (eg couch motion accuracy, etc).41 Generally this margin for fractionated RT, whether on a Linac with image guidance provided with CBCT imaging or a MR-Linac, should be 3 mm.3,41 Recent work have shown feasibility of real-time tracking in other anatomic sites on both the ViewRay and Elekta MR-Linac systems which may potentially allow future reduction in PTV.42,43
Online Adaptive RT
As CBCT on a traditional Linac usually offers positional verification based on bony anatomy only, online (ie, with patient in treatment position) adaptation necessitates an integrated MR-Linac for intracranial soft-tissue visualization. The challenges of this approach include potentially lengthy on-table times impacting patient comfort (contrast administration, MRI acquisition, contouring, full re-planning, QA, all in treatment position) and, therefore, resource constraints (fewer patients per day, reduced throughput on an integrated unit, staffing of physicians and physicists to perform online adaptive RT). Advantages may be significant, as the ability to visualize soft tissue daily provides opportunities to safely reduce CTV margins through plan adaptation and more immediate recognition of anatomic shifts.
Initial experiences in online adaptive RT for GBM have been described on both the Unity and MRIdian A3i MR-Linacs. The Unity MR-Linac combines a 1.5 T MR with a Linac, and the initial experience using rigid translations described by Tseng et al.18 demonstrated 30% of the cases exhibiting soft tissue or target changes prompting offline adaptive re-planning. Trials in adaptive RT using the MR-Linac with reduced CTV margin and weekly online re-planning after gadolinium-enhanced MR acquired on the MR-Linac table itself are ongoing, and explore the feasibility of this approach for dose escalation.20–22 The MRIdian A3i combines a linear accelerator with 0.35 T MR-capability and now includes a dedicated brain treatment package (BrainTxTM) including brain coil and integrated immobilization systems and specific intracranial sequences. Initial experiences on this unit reported fluctuations in dimensions of tumor, edema, and cavity during a course of treatment,44 with subsequent simulated adaptive RT planning of 10 cases demonstrating reduction in dose to normal brain tissues with adaptation, including reduction in dose to the hippocampus.45,46 La Rosa et al. reported imaging and workflow in treatment of a patient undergoing chemoradiotherapy for GBM demonstrating similar on-treatment times (35.3 min per fraction), though no adaptation occurred.19 Gadolinium was given every 5 fractions to visualize the surgical cavity and, in this report of a single patient, significant decreases in volumes of up to 87% of the FLAIR and 68% of the cavity were observed supporting the non-static nature of tumor bed dynamics during a course of treatment.
In the offline pre-treatment preparation step, CT- and MRI-simulation are undertaken to generate a reference plan. The simplest approach utilizes an isocenter or couch shift online to account for setup uncertainty, after which re-optimization of the treatment plan occurs. In the setting of full online adaptation, targets and OAR are either manually re-contoured or deformed to reflect the anatomy on the day of treatment. After generation of an online plan, the new plan is reviewed and approved, and an independent pre-treatment QA (dose check) is performed, subsequent to which treatment is delivered.
Offline Adaptive RT
Offline adaptive RT can be triggered by visualization of soft-tissue or target changes observed during a treatment course, either via planned imaging at specified intervals or due to a clinical change in the patient prompting diagnostic imaging.
Owing to data demonstrating clinically relevant tumor dynamics during a standard 6-week course of chemoradiotherapy, a planned CT, and MRI re-simulation may be performed mid-treatment to allow adaptation. In this workflow, the new CT is used as the primary data set, with imported initial contours, and image fusion of the initial planning MRI and re-simulation MRI. The radiation oncologist reviews the imaging and determines whether a re-plan is required.
A significant challenge of adaptive RT strategies is the immense resource utilization required. This includes human resources required for RT simulation, planning, bookings, quality assurance, and scanner time on both CT- and MR-simulation. There may be competing departmental resource constraints amongst different disease sites which will have significant implications in adaptation strategies. Technical advancements in the treatment planning system (TPS), allowing for deformable registration, auto-segmentation, and contour propagation, along with systems automating dose monitoring and accumulation may ultimately create more efficiency.
Previous studies utilizing a 2-phase approach have confirmed target volume changes of GBM during a treatment course and benefits of improving target coverage and dose reduction of normal brain tissue with off-line adaptation before proceeding with phase 2.47–49 The resource requirements and utilization of an offline adaptive approach with planned re-planning depend on the organization of the radiation oncology department, and the optimal timing and frequency can be challenging to determine. There may be a sub-population of patients where adaptation is not required, and these resources can be directed to other needs. Further study is required to identify the appropriate patients and thresholds used to trigger re-planning while considering the intended benefit, which may vary from institution to institution based on availability of resources.
Future Directions
With greater access to MR, GBM which was previously thought of as being static has now been shown to demonstrate significant tumor dynamics during a course of treatment. Future advancements in RT delivery can build upon these data to facilitate adaptive planning strategies with the aim of improving survival outcomes and reducing toxicities. Frequent adaptation of RT plans requires significant resources, and further work is needed to improve efficiency. Adaptation inherently opens opportunities for unique dosing strategies and integration of imaging-based biomarkers that may have a prognostic/predictive role. The safety of CTV margin reduction through online adaptation with respect to risk of marginal failure is currently the subject of ongoing investigation.20–22 With CTV margin reduction, contour variability may become a greater issue and consensus contouring recommendations exist for CT-MRI and MRI-only workflows.1 One challenge in particular is the variability in the inclusion of T2-FLAIR signal. Imaging-based biomarkers are of specific interest in the differentiation between tumor-associated edema and infiltrative disease,50 and ultimately may aid radiation oncologists in decision-making in this process.
Improving Efficiency in Adaptive RT
There are at least several areas where potential improvements can be made in the adaptive process. For example, improvements in auto-segmentation via more sophisticated software algorithms and/or hardware (eg, incorporating a 6-degree of freedom couch) would lead to substantial reduction in time and resources. Moreover, automation of certain re-planning elements can reduce the anticipated increase in workload with adaptive RT. Deformable image registration addresses anatomical shifts and deformations between the initial planning and images acquired at the time of adaptation. The algorithms used are often unique to the TPS and permit subsequent features such as contour propagation, dose warping and summation.
Manual re-contouring of targets and OARs is time consuming and can prolong treatment times in online adaptive approaches. Automated methods to re-contour, or at least to provide a starting point would improve the efficiency of this process. As a basic approach, contours can be propagated via a deformable algorithm, and subsequently manually adjusted. This may be challenging, as soft-tissue changes do not necessarily follow shifts in bony anatomy. An alternative is auto-segmentation that can be atlas-based or deep-learning-based.51 It has previously been shown that semi-automated OAR propagation improves the accuracy of the reported dose to the OAR.52
Integrating these features, adaptive RT affords another opportunity in that it allows assessment of the total delivered dose (dose summation/accumulation). Summation of total dose during a treatment course in theory can allow for accurate assessment of potential over- or under-dosage to tissues and to ensure therapeutic goals are met. Nevertheless, this is a subject of further study in the context of changing anatomy over time and how to appropriately interpret the accumulated dose.
Dosing Strategies
Improved serial visualization of soft tissue targets during an adapted course of RT may allow for safe strategies in dose escalation to improve outcomes. In younger patients who are considered candidates for 6 weeks of chemoradiation, the NRG-BN001 trial reported preliminary results evaluating 229 patients randomized between standard-dose RT (60 Gy in 30 fractions) vs. dose-escalated RT to 75 Gy in 30 fractions.53 All patients were treated using photons and a 2-phase approach where the standard-dose arm included a 2 cm margin beyond the T1w contrast-enhancing volume and the dose-escalated arm used only a 5 mm margin beyond the T1w contrast-enhancing volume in phase 2. It should be noted that radiotherapy planning was primarily based on the post-operative MRI obtained within 72 h of resection, although a repeat planning MRI was allowed if more than 3 weeks have elapsed between surgical resection and radiation planning. Median OS was 18.7 months after dose-escalated RT and 16.3 months after standard-dose RT. The difference between the arms were not statistically significant, nor was the difference between median progression-free-survival (PFS). The trial has been closed to accrual and the results of a third dose-escalated arm with proton therapy is still anticipated.
The standard treatment of the elderly patient with GBM is a 3-week course of RT (40 Gy in 15 fractions) delivered with concurrent TMZ.54 A contributor to the poorer outcomes in the elderly population may be that the hypofractionated regimen (40 Gy in 15 fractions, EQD2: 42.7 Gy2 with α/β = 8) is not biologically equivalent to 60 Gy in 30 fractions, although a 6-week course has been shown to be poorly tolerated by patients older than 65.55,56 A number of studies have evaluated a dose-escalated 3-week RT regimen (roughly biologically equivalent to 60 Gy in 30 fractions) with encouraging results.57–61 MR-guided adaptive RT strategies are well suited for this approach both from an efficacy and safety perspective. Routine adaptation will ensure minimization of tumor under-dosing due to the non-static nature of GBM. Furthermore, a reduced CTV margin would be safer to evaluate in this context, especially in a vulnerable elderly population. This strategy is being evaluated in a prospective setting.22
A recent report from the RANO resect group retrospectively compiled data from 7 centers and demonstrated prognostic significance of the extent of resection in overall survival outcomes, leading to 4 proposed RANO categories of extent of resection (EOR): supramaximal contrast-enhancing resection, maximal contrast-enhancing resection, submaximal contrast-enhancing resection, and biopsy.62 In this work, the majority of patients (82.5%) were treated with chemoradiation per EORTC-26981/22981,63 but RT details with respect to margin and dosimetry were not provided. The proposed RANO categories may serve for stratification and overall design of clinical trials; however, the implication of these data on radiation dosing or margin strategies based on EOR is unclear but may be a topic of future investigation.
Functional Imaging Strategies on MR-Linac
Acquisition of MRI routinely during a course of chemoradiotherapy affords potential novel imaging sequences to assess progression or response that can individualize therapy using noninvasive biomarkers. Some techniques, such as diffusion weighted imaging (DWI) and dynamic contrast-enhanced (DCE) perfusion were initially developed on the earliest MRI systems at low field (0.3–0.5 T), while other techniques such as chemical exchange saturation transfer (CEST) were initially demonstrated using 3 T and 7 T MRI,64,65 but not only has it now been translated to the 1.5 T setting,66 it has been shown to be feasible on the MR-Linac.67,68 Advanced functional MR techniques that characterize cellular, biophysical, microstructural, and metabolic features of tumors that are known to be associated with radiosensitivity and radioresistance. These properties include tumor cell density (DWI), metabolism (chemical exchange saturation transfer—CEST and magnetization transfer—MT, as well as magnetic resonance spectroscopy), vascularity and blood flow (perfusion), hypoxia (with T1/T2 relaxometry), as well as identification of functional brain networks (with blood oxygenation level dependent BOLD imaging).
Metabolic imaging is promising for adaptive CTV margin definition. CEST MRI is capable of acquiring metabolic information and is sensitive to low (millimolar) metabolite concentrations.69 A particular version of CEST called amide proton transfer (APT-CEST) exploits the proton exchange in amide bonds found in proteins and peptides.70,71 APT has been shown to predict treatment response in GBM,64,65,72 discriminate between different CNS tumor types,73–76 distinguish radiation necrosis from tumor,73–77 and differentiate pseudoprogression from true progression.78 A related technique uses the magnetization transfer (MT) effect to assess white matter degradation, and also has been shown to predict treatment response in GBM.66,79 A limitation is that it is challenging to collect all the aforementioned imaging sequences in a single treatment fraction and imaging session. However, it is possible to perform different imaging sequences over multiple days, and then use the combined information to make updates to a treatment plan, for example, at weekly temporal resolution. As a proof-of-principle, a daily multiparametric imaging protocol has been developed for use with a 1.5 T MR-Linac which includes DWI, CEST, MT, BOLD, and T1/T2 mapping (Figure 3).18,67 Each sequence was obtained at separate treatment fractions, with up to 1 week between repeated measurements for certain sequences. Application of this protocol demonstrated that in vivo CEST and MT images could be successfully obtained within a clinical MR-Linac workflow, with CEST signal changes over time being detectable in individual patients.

Example multiparametric MRI protocol on a 1.5 T MR-Linac: The standard structural images (pre-contrast T1-weighted and FLAIR) and quantitative maps (T1 relaxation time, T2 relaxation time, apparent diffusion coefficient (ADC), MT semisolid fraction, CEST asymmetry and the default mode network functional connectivity map from resting-state fMRI) from the same slice are shown. The T1-weighted, FLAIR, ADC and CEST maps were acquired at fraction 14, BOLD at fraction 15, and MT, T1 and T2 maps at fraction 27. The GTV for fraction 14 is outlined on the T1-weighted image.
On a low-field MR-Linac system, longitudinal acquisition of diffusion MRI has been shown to be feasible during a radiotherapy course, although not specifically for brain tumors.80 Although low signal-to-noise (SNR) ratio has been a concern in low field systems given the linear dependence of the SNR as a function of the magnetic field strength, the T1 relaxation rates of tissues usually decreases with field strength, therefore partially mitigating the SNR loss, and the diffusion EPI readout may benefit from the lower field strength due to reduced absolute off-resonance frequencies. A multicontrast multiparametric MRI method, STrategically Acquired Gradient Echo (STAGE), originally introduced on diagnostic 3.0 T MRIs, has been optimized for 0.35 T to acquire routine quantitative MRI during a treatment course on a 0.35 T MR-Linac. In a pilot study of brain tumor patients, differential maps for R2* and T1 maps were shown to be sensitive to local tumor changes.68 An example of a daily multiparametric MRI protocol on a 0.35 T MR-Linac is shown in Figure 4. Another emerging technique, known as magnetic resonance fingerprinting (MRF), whereby data is acquired by continuously varying pulse sequence parameters such as flip angel and repetition time, initially characterized on high-field MRI simulators and the 1.5 T MR-Linac,81,82 was demonstrated to be feasible at 0.35 T to potentially permit rapid, reproducible acquisition of T1 and T2 maps on low-field MR-guided RT systems.83
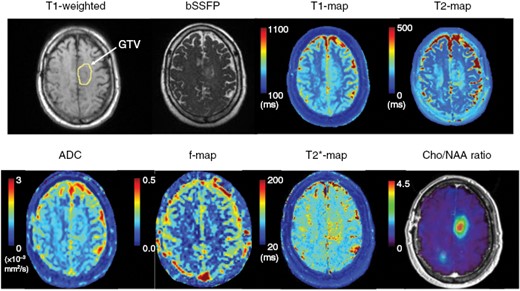
Example daily multiparametric MRI protocol on a 0.35 T MR-Linac. The default imaging type on the system is balanced steady state free precession (bSSFP), which is fluid bright similar to T2-weighted images. Also shown is T1-weighted without contrast with a yellow circle indicating the enhancing tumor component. Quantitative maps from STAGE (T1, T2, T2*) and DWI (ADC and IVIM f-map) are demonstrated. From the same patient, a Cho/NAA map (relative to normal-appearing white matter) from sMRI acquired at 3 T is also provided.
Adaptive Radiotherapy Based on Physiologic Changes During RT
An important question is how might advanced imaging techniques be incorporated into margin definitions or an individualized adaptive treatment paradigm? One possibility is to use the functional techniques to create a target region for dose escalation. A thorough review on the integration of biologically informed multi-modality imaging in the treatment of GBM is presented in the accompanying article in the supplement. Hyperperfused tumor with an elevated tumor cerebral blood volume (CBV) and hypercellular tumor as reflected by low apparent diffusion coefficient (ADC) values have been shown to be associated with worse PFS,84,85 and these regions may extend outside of conventionally defined target volumes.86,87 Further, changes in diffusion and perfusion metrics over a 6-week course of chemoradiation has been shown to be predictive of early progression.37 Studies have reported the feasibility of implementing a workflow with multiparametric DCE- and DWI-guided radiation therapy, which permits the identification of resistant sub-regions during chemoradiation for GBM patients.88,89 Early phase 2 data adopting a dose escalation strategy to these sub-regions have shown encouraging outcomes with respect to overall survival, neurocognitive function, symptom burden, and quality of life.90 The proportion of in-field failure on this study was lower than that expected based on historical data. Proton magnetic resonance spectroscopic imaging (MRSI) permits characterization of metabolic properties of tumors, and MRSI parameters have been found to predict for PFS and OS in GBM patients.91 A recently published randomized trial in GBM patients treated with dose-escalation to MRSI abnormalities vs. standard dose fractionation over 6 weeks confirmed the safety of dose escalation to 72 Gy in 2.4 Gy fractions per day but did not show benefit in overall survival. It is noteworthy that RT on this trial was non-adapted and used standard CTV margins.92 Another single-arm pilot study from a multi-institutional group used a higher resolution and sensitivity whole-brain MRSI technique termed spectroscopic MRI (sMRI) to identify high-risk areas often extending far outside of the T1-enhancement. These were boosted to 75 Gy in 30 fractions and showed promising survival and toxicity outcomes.93 Several centers have published prospective, non-randomized outcomes on the use of 18F-DOPA PET/CT-guided or 18FET-PET-guided radiotherapy for GBM, using both conventionally fractionated and hypofractionated regimens of photon and proton RT.94–98 It is worth noting that grade 3+ radiation necrosis has been observed in up to 13–40% on these studies. Finally, a proof-of-principle study evaluated the ability to retrospectively generate dose-escalated radiation plans based on MT semi-solid macromolecular fraction mapping. Dose-escalated plans were successfully generated delivering 115–120% of the original prescription to a target boost region defined by selecting the sub-volume of the GTV with low semi-solid fraction values, with the rationale being that progressors have lower semi-solid fraction values compared to non-progressors.13 Ongoing research includes expanding the spatial coverage for CEST and MT with efficient whole-brain acquisitions based on fast saturation transfer approaches.99
Additional work relates to better understanding of specific patterns of failure100 which could help to improve future targeting of areas and would be relevant to guide alternative strategies such as dose escalation and inform smart boosting strategies in conjunction with CTV margin adaptation. More studies are needed to determine which of the functional imaging maps can best predict the areas of response or recurrence following treatment, ideally on a per-patient basis. This may involve spatially assessing the maps with respect to relevant outcome metrics including locations of failure/recurrence, PFS and overall survival. Evaluating different tumor sub-regions (generated by delineation or automatic segmentation methods at a suitable threshold for each parameter map) or using a radiomics or deep-learning approach could be strategies for predicting the likelihood of failure.101 For example, a probability map of recurrence could be estimated for each patient, where “high-risk” regions are targeted with an escalated dose. Accurate predictions would also need to consider tissue displacement due to growth or shrinkage of the tumor and/or cavity. Once the recurrence region can be predicted with sufficient confidence, then steps will be needed to generate a feasible/safe dose plan. New dose-escalated treatments aim to improve response and lower recurrence rates but may result in other adverse effects including increased risk of radiation necrosis for high doses. As such, refinement may require serial clinical studies, for example, using new patient outcomes from those adaptive treatments, to generate increasingly accurate predictions of recurrence and tumor targets.
Conclusions
The integration of MRI into routine RT practice has enabled visualization of GBM tumor dynamics throughout a course of chemoradiation. Adaptive RT strategies, whether online or offline, combined with CTV margin reduction with MR image guidance have the potential to improve the therapeutic ratio. Within the adaptive RT framework, novel dosing strategies and incorporation of functional imaging are areas of active investigation.
Supplement sponsorship
This article appears as part of the supplement “Pushing the Boundaries of Radiation Technology for the Central Nervous System,” sponsored by Varian Medical Systems.
Conflict of interest
C.T.: Travel accommodations/expenses and honoraria for past educational seminars by Elekta, belongs to the Elekta MR-Linac Consortium, and is an advisor/consultant with Sanofi and Abbvie. EAM: Research Grants: ViewRay, Inc. Travel accommodations/expenses: ViewRay, Inc. S.S.: Research Grant: Novocure, Inc. Consultant: Novocure, Inc., Accuray, Inc. MR: Co‐inventor of and owns associated intellectual property specific to the image‐guidance system on the Gamma Knife Icon, none related to this work. A.S.: Consultant with Elekta AB, BrainLAB, Merck, Abbvie, Roche. Vice president of International Stereotactic Radiosurgery Society (ISRS) and past Vice Chair of the Elekta MR-Linac Consortium. Past educational seminars with AstraZeneca, Elekta AB, Varian, BrainLAB. Research Grants: Elekta AB, Varian. Travel accommodations/expenses: Elekta, Varian, BrainLAB.
Funding
None.
References
- neuroimaging
- magnetic resonance imaging
- radiation therapy
- glioblastoma
- biological markers
- surgical procedures, operative
- technology
- diagnostic imaging
- neoplasms
- radiochemotherapy
- functional magnetic resonance imaging
- simulators
- radiotherapy systems, linear accelerator
- workflow
- radiation therapy, adaptive
- gross tumor volume
- clinical target volume
- molecular profiling