-
PDF
- Split View
-
Views
-
Cite
Cite
Chris Jones, Matthias A. Karajannis, David T. W. Jones, Mark W. Kieran, Michelle Monje, Suzanne J. Baker, Oren J. Becher, Yoon-Jae Cho, Nalin Gupta, Cynthia Hawkins, Darren Hargrave, Daphne A. Haas-Kogan, Nada Jabado, Xiao-Nan Li, Sabine Mueller, Theo Nicolaides, Roger J. Packer, Anders I. Persson, Joanna J. Phillips, Erin F. Simonds, James M. Stafford, Yujie Tang, Stefan M. Pfister, William A. Weiss, Pediatric high-grade glioma: biologically and clinically in need of new thinking, Neuro-Oncology, Volume 19, Issue 2, 1 February 2017, Pages 153–161, https://doi.org/10.1093/neuonc/now101
- Share Icon Share
Abstract
High-grade gliomas in children are different from those that arise in adults. Recent collaborative molecular analyses of these rare cancers have revealed previously unappreciated connections among chromatin regulation, developmental signaling, and tumorigenesis. As we begin to unravel the unique developmental origins and distinct biological drivers of this heterogeneous group of tumors, clinical trials need to keep pace. It is important to avoid therapeutic strategies developed purely using data obtained from studies on adult glioblastoma. This approach has resulted in repetitive trials and ineffective treatments being applied to these children, with limited improvement in clinical outcome. The authors of this perspective, comprising biology and clinical expertise in the disease, recently convened to discuss the most effective ways to translate the emerging molecular insights into patient benefit. This article reviews our current understanding of pediatric high-grade glioma and suggests approaches for innovative clinical management.
In a remarkably short period of time, our understanding of the origin and biological features of childhood brain tumors has been revolutionized through the application of genome- and epigenome-wide molecular profiling techniques.1 The resulting data have led to a fundamental reclassification of these diseases; moving from a solely morphology-based categorization to molecular-based separation into subgroups with meaningful clinical correlation, particularly in terms of age at presentation, anatomical location, and prognosis.1–6 Most importantly, these subgroups appear to have distinct cellular origins and biological drivers that could lead to specific and effective therapeutic targets.7 Despite these scientific advances, clinical trials for children with gliomas are usually based on previously tested and often ineffective regimens that ignore the key biological differences between tumors in adults and children. Furthermore, these studies are largely underpowered to detect potential subgroup-specific efficacy among an unselected patient population.
Biological advances are occurring rapidly in the field of pediatric high-grade gliomas (pHGGs; classified as World Health Organization [WHO] astrocytomas grades III and IV), for which there have been no significant improvements in survival in decades.8 In general, clinical trial design has not kept pace with the recent biological advances and there is a risk of recapitulating the mistakes of the past. At a recent symposium held in San Francisco on February 5–8, 2015 a working group of scientists and clinicians met to discuss how to better define diffusely infiltrative gliomas in children and to identify new ways to translate biological results into more effective patient care. In particular, we focused on a fundamental question facing the field: what key aspects of the biology of pHGG and diffuse intrinsic pontine glioma (DIPG) can drive clinical practice forward, and how do neuro-oncologists utilize this new biology to develop, run, and analyze clinical trials likely to improve outcome? (Box 1).
Subdivision of HGGs is based on an arbitrary histological classification system and age cutoff is not reflective of their unique disease biology, and is unhelpful for describing diffusely infiltrating gliomas of children and young adults.
Integration of genetics, epigenetics, and clinicopathological features better defines robust tumor subgroups with significant translational relevance.
While grouping patients into large cohorts reduces the number of patients needed for a clinical trial, heterogeneity in those groups limits the ability to interpret the data. Trials need to have clearly “defined” patients with the appropriate target to be eligible for a clinical trial using a treatment against that target.
Children are the healthiest dying patients but they do die of their tumors. Funders, regulatory agencies, pharma, and clinicians have to work together to develop strategies that limit unnecessary risk but also offer reasonable hope to these patients. They cannot be ignored simply because they are children and/or because their tumors are rare.
Unique Biological Drivers and Distinct Therapeutic Targets
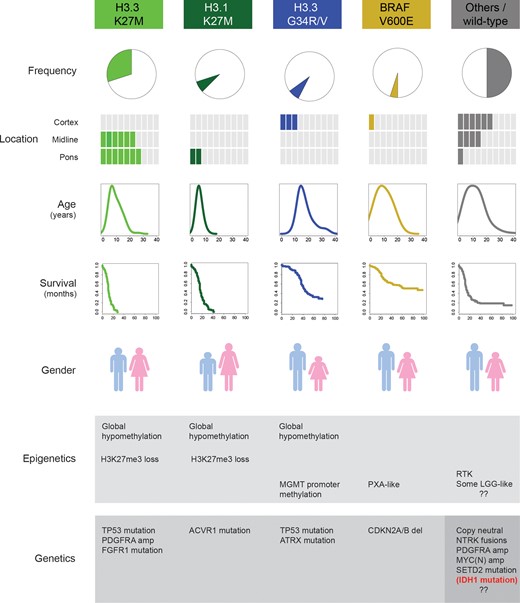
Biologically and clinically defined subgroups of pediatric infiltrating glioma. Specific, selective, recurrent, and mutually exclusive mutations in the genes encoding the histone H3.3 (H3F3A) and H3.1 (HIST1H3B, HIST1H3C) variants, along with BRAF V600E, mark distinct subgroups of disease in children and young adults. There are clear differences in location, age at presentation, clinical outcome, gender distribution, predominant histology and concurrent epigenetic and genetic alterations. The remaining half of tumors comprising these diseases harbor numerous, partially overlapping putative drivers or other (epi)genomic characteristics, but as yet do not form well-validated biological and clinical subgroups. The small proportion of children (mostly adolescents) with IDH1 mutations represent the lower tail of age distribution of an otherwise adult subgroup, and are excluded here.
These mutations rewire the epigenome17–20 and deliver potent and distinct oncogenic insults to susceptible pools of progenitors cells, likely originating early in neurodevelopment.18,21 These distinct origins are also reflected in the anatomical distribution of tumors carrying the mutations, with H3.3 G34R/V found exclusively in the cerebral hemispheres, H3.3 K27M distributed throughout the midline structures (including the thalamus, brainstem, cerebellum, and spine), and H3.1 K27M restricted to the pons22–25 (Fig. 1). Indeed more than 85% of DIPGs, a nonsurgically resectable glial tumor of the pons which may display histological features ranging from grade II to grade IV, harbor a K27M mutation in one or other histone variant, with H3.1 mutant tumors displaying a younger age, distinct clinicopathological and radiological features, and a slightly longer survival time.26 This mutation confers loss of the transcriptionally repressive trimethyl mark at lysine 27 on the histone tail, which may be easily detected by immunohistochemistry,27,28 and the first preclinical studies targeting this mechanism in DIPG model systems are pointing the way to future novel clinical interventions.29–31 The relationship of these tumors to the remaining histone wild-type cases originally diagnosed as DIPG is uncertain. A small number of histologically low-grade glioneuronal tumors have been described that harbor both H3 K27M and BRAF V600E mutations, and these may have an improved prognosis compared with typical high-grade K27M tumors (although the numbers remain small).32,33 Thus, in very rare exceptions, K27M mutation can be compatible with longer survival.
Although both histone H3 mutations lead to a reduction in DNA methylation throughout the epigenome, K27M globally and G34R/V mostly at subtelomeric regions,21 there are notable exceptions. Of particular clinical relevance is hypermethylation of the MGMT gene promoter region, which encodes a DNA repair enzyme associated with resistance in adult glioblastoma to alkylating agents such as temozolomide (TMZ).34 The role of TMZ resistance is not entirely clear in children.35–38 This may be due to MGMT methylation being predominantly found in the H3.3 G34R/V subgroup and less frequent in tumors with K27M mutations,39 likely contributing to the lack of clinical response to TMZ in most HGG including DIPG across numerous trials.40–43 Aside from possessing a distinct epigenetic profile, these histone-defined subgroups frequently co-segregate with differential secondary genetic alterations, such as ATRX in the H3.3 G34R/V group,8,22FGFR1 in thalamic H3.3 K27M,24 and seemingly uniquely in human cancer,44ACVR1 mutations in H3.1 K27M DIPG,22–25 a subgroup in which the otherwise common TP53 mutations are absent (Fig. 1).
Despite the ability to subclassify tumors on the basis of histone H3 mutations, more than half of all childhood diffusely infiltrating gliomas do not fall into these categories. A small proportion (<5%) harbor hotspot mutations in the IDH1/2 genes associated with a global hypermethylation (“G-CIMP”)21,45 and likely represent the younger tail of an age distribution for these tumors, which peaks at around 40–45 years of age, as reviewed elsewhere.5 A larger group (5%–10%) of predominantly cortical tumors harbor an activating BRAF V600E mutation,46 have histological and epigenetic similarities to pleomorphic xanthoastrocytoma,39 and have a better clinical outcome. Unlike lower-grade gliomas with mitogen-activated protein kinase pathway activation, they frequently co-segregate with CDKN2A/B (p16) deletion39,47 (Fig. 1). Patients whose tumors have these mutations are candidates for target-driven clinical trials48,49 and provide a paradigm for translational progress in this disease (below).
The remaining tumors form a heterogeneous group with numerous potential driver events, which are poorly defined in terms of distinct molecular and clinicopathological features (Fig. 1). Mutations in the histone methyltransferase SETD2 may extend the proportion of tumors with dysregulated H3K36 trimethylation,50 the likely consequence of H3.3 G34R/V mutation,18 though the functional overlap between these mutations has not been determined. They otherwise frequently harbor alterations associated with receptor tyrosine kinase activation, most commonly through amplification and/or mutation of PDGFRA51,52 (and in contrast to adult glioblastoma,53 only rarely through EGFR involvement2,5), though this is also often found in association with H3.3 K27M mutation. Gene fusions involving the NTRKs 1–3 are found in a small proportion of cases, often in infants,22 and may overlap with a group of low-grade gliomas with similar genetic alterations.54MYCN (and to a lesser extent, MYC) amplifications are also seen, although it is unclear to what extent they mark a distinct subgroup either in nonbrainstem tumors or DIPG.22–25 Together, integrated genetic and epigenetic characterization of pHGG and DIPG may allow for the delineation of distinct prognostic risk groups which will inform future clinical trial interpretation—for instance, a high-risk group based upon K27M mutation and/or amplification of PDGFRA, MYCN, etc, and an intermediate group enriched for G34R/V and IDH1 mutations.39
Finally, there is a significant proportion of nonhistone, non-IDH1, non-BRAF mutated tumors with remarkably stable genome profiles,9,12,22,25,39 for whom archetypal genetic events remain elusive. Some of these may eventually be reclassified as other entities on the basis of their epigenetic profiles; however, there likely remains a uniquely pediatric group of these highly malignant tumors which thus far defy definition by classical “driver” molecular alterations.
Tumor Microenvironment of the Childhood Brain
The developmental context in which pHGGs arise may provide further insights into the pathobiology and potential therapeutic targets for this group of tumors. While the cell of origin for pHGGs is still controversial,55 multiple lines of evidence point to a neural precursor cell, possibly in the oligodendroglial lineage.31,56,57 Consistent with this hypothesis, pHGGs occur in relatively discrete spatial and temporal patterns21 that coincide with waves of developmental myelination in the childhood and adolescent brain.57,58 The observation that elevated neuronal activity promotes the proliferation of oligodendroglial lineage precursor cells59 prompted an examination of the role active neurons may play in the pHGG microenvironment. Excitatory neuronal activity was found to robustly promote the growth of pHGG, including both pediatric cortical glioblastoma and DIPG.60 Neuronal activity-regulated pHGG growth depends upon activity-regulated secretion of neuroligin-3 (a synaptic adhesion molecule that promotes glioma proliferation through stimulation of the phosphatidylinositol-3 kinase–mammalian target of rapamycin pathway) and brain-derived neurotrophic factor.60 These observations highlight the manner in which pHGGs “hijack” mechanisms of development and plasticity in the childhood brain, and may elucidate novel therapeutic strategies in the future.
Immune cells, particularly tumor-associated macrophages (TAMs), are known to play an important role in the microenvironment of low-grade pediatric gliomas and in adult high-grade gliomas.61 Mouse models of low-grade glioma reveal a tumor growth-promoting effect of TAMs.62,63 Similarly, in adult gliomas the number of cells in the tumor mass immunopositive for the activated microglia/macrophage marker CD68 increases with increasing tumor grade,64 and M2 phenotype microglia appear to promote glioblastoma growth.65 The effects of TAMs on glioma growth and progression appear to depend on the TAM activation state along the M1–M2 spectrum, with M2 phenotype TAMs promoting tumor growth and M1 phenotype TAMs potentially inhibiting growth.65 Gene expression data from pediatric infiltrating astrocytoma demonstrate enrichment in expression of M1 and M2 microglia/macrophage gene signatures,66 and differences in TAM phenotype may explain the observation that in pilocytic astrocytomas of childhood, tumor recurrence correlates inversely with CD68+ TAMs.67 As in other gliomas, microglia account for approximately one third of the cellular mass of DIPG.68 The functional role TAMs may play in DIPG and other infiltrating gliomas of childhood remains to be determined.
While our understanding of TAMs in pediatric infiltrating gliomas remains incomplete, the potential of harnessing the power of the immune system in pediatric gliomas is presently under exploration using a variety of immune-modulatory strategies, including blockade of programmed cell death protein 1 (ClinicalTrials.gov identifiers NCT01952769 and NCT02359565) and tumor vaccine strategies (NCT01400672 and NCT00874861). It should be noted that one blocker trial of programmed cell death protein 1 using pembrolizumab (NCT02359565) was stopped for safety concerns, highlighting the precarious location of the pons for inflammation and subsequent edema.
A Clinical Conundrum and a Way Forward
Despite a significant number of prospective clinical trials for children with HGG, either at initial diagnosis or recurrence, over the past 4 decades, there has been little improvement in patient outcome. It is important to note that while most studies examining adult brain tumors are limited to WHO grade IV astrocytomas (ie, glioblastoma), the majority of pHGG clinical trials have included both WHO grade III (anaplastic astrocytoma) and grade IV tumors. The first prospective, randomized clinical trial for children with HGG was published in 1989 by the Children's Cancer Study Group and showed a significant improvement in outcome using adjuvant radiation therapy (RT) followed by pCV chemotherapy (prednisone, chloroethyl-cyclohexyl nitrosourea [CCNU], and vincristine) compared with RT alone.69 In this study, the addition of chemotherapy led to a dramatic increase in 5-year progression-free survival, from 16% to 46%, and as a result no subsequent cooperative group study used an “RT-only” arm going forward. The survival rates on both treatment arms on this particular trial far exceeded what has been observed in more recent studies; a subsequent retrospective central review demonstrated inclusion of large numbers of low-grade gliomas on the older HGG study,70 explaining the discrepant results. Contemporary trials using updated neuropathology criteria and central review have shown 3-year event-free survival (EFS) and overall survival (OS) rates of ∼10% and 20%, respectively.35 The successor Children's Cancer Group Study (CCG-945) performed between 1985 and 1990 comparing preradiation and postradiation chemotherapy with the 8-in-1 regimen to postradiation procarbazine, CCNU, and prednisone, also demonstrated the difficulty in utilization of institutional diagnosis; on a retrospective, central review 51 of 172 tumors were classified as discordant.70,71 The majority of the differences were again inclusion of low-grade tumors. Patients with HGGs in this study had a similar poor OS rate of 20% at 5 years. It demonstrated that the strongest factors associated with more favorable outcome were extent of resection (>90% resection), low methylation-inhibited binding protein 1 indices, and non-overexpression of p53.
Single-agent TMZ, when administered during and after RT, has been shown to significantly prolong EFS and OS in adults with glioblastoma compared with RT alone; however, the use of a similar regimen in children in the Children's Oncology Group (COG) single-arm study ACNS0126 did not improve outcome compared with historical controls treated with different adjuvant chemotherapy regimens.35 While expression of O6-DNA methylguanine-methyltransferase was confirmed as a prognostic marker, the predictive value for benefit from TMZ has not been demonstrated as in the adult setting.34,72 In the subsequent COG HGG trial, ACNS0423, CCNU was added to TMZ during maintenance, and the final study results have not been published. However, 1-year OS was reported as 100% in patients with isocitrate dehydrogenase 1 (IDH1) mutant tumors versus 81% in those with IDH wild-type tumors (P = .03, one-sided log-rank test),73 underscoring the strong prognostic value of IDH1 mutations in pediatric HGG patients, similar to adults.
The most recent COG HGG trial, ACNS0822, compared 2 different experimental arms with vorinostat or bevacizumab during RT with a control arm with TMZ during RT. Patients on all 3 arms received bevacizumab during maintenance therapy post RT. The study was initially planned as a “pick-the-winner” phase II design to be advanced into phase III testing, but the study was permanently closed in 2014 during phase II, as no arm showed any clear superiority.74
In patients with pHGG treated on the German HIT-GBM-C cooperative group study with intensive chemotherapy during and after RT (cisplatin, etoposide, and vincristine, with one cycle of cisplatin, etoposide, and ifosfamide during the last week of radiation, and subsequent maintenance chemotherapy followed by oral valproic acid), survival was better than that seen in prior HIT-GBM studies in the subgroup of patients with HGG who had undergone gross total resection (5-year OS rate 63% vs 17% for the historical control group, P = .003, log-rank test).75 Molecular data were not provided, however, rendering the data difficult to interpret.
The use of adjuvant pCV in addition to RT provided no survival benefit in patients with brainstem tumors, including DIPG.76 Furthermore, no subsequent DIPG trial has shown convincing benefit of any adjuvant therapy beyond RT alone, independent of whether the chemotherapy is given before, during, and/or after RT.43 Additionally, the use of higher doses of RT, given in hyperfractionated regimens, to cumulative doses as high as 7800 cGy, has shown no survival benefit and increased neurotoxicity.77,78
The studies mentioned above are but a small number of those that have attempted to improve the outcome of nonbrainstem HGG and DIPG. Unfortunately, based on a limited understanding of the biology and heterogeneity of these tumors, most studies resulted in added toxicity and little activity. Even with the increased recognition of the clinical and biological differences observed between different subgroups of tumors, they were treated as a single uniform group. Incorporation of biology into diagnostic evaluations has been slow, and until recently robust markers were not available. There was reluctance by regulatory agencies and physicians to put patients at increased risk for obtainment of tissue for biological assessment unless the results were used to guide therapy, especially for DIPGs, as DIPGs arise in an eloquent brain area for which resection cannot be accomplished, and biopsy was considered to carry substantial risk. DIPGs frequently are associated with typical radiological features on MRI; a decision to forgo surgical biopsy resulted in limited tissue being available for subsequent molecular analysis. Excessive caution and the inability to use biological information to guide clinical studies resulted in a very limited understanding of the biology of DIPGs.
Only through the efforts of centers that performed surgical biopsies on patients prior to treatment, coupled with increased emphasis on rapid obtainment of autopsy tissue for molecular analysis, did the field advance more in 5 years than in the prior 50 years combined. The initial reports on the heterogeneity within DIPG13 and identification of potential targets79 were the first in rapid succession that made the current conference even possible. As described above, the identification of the chromatin mutations, their association with aberrant pathway activation that appears to segregate into different groups, and the identification of a new target not previously associated with any cancer has changed the very landscape by which we think about these tumors.
The growing recognition of HGG, especially pHGG, as a biologically diverse group of tumors rather than a single disease, has profound implications for the design and planning of future clinical trials. Mounting evidence indicates that clinical behavior more closely follows tumor biology (ie, molecular genetics and epigenetics) rather than histopathological grading or traditional clinical factors, such as extent of resection. Moreover, novel and emerging drugs for the treatment of HGG will likely target only a subset of pHGG, resulting in relatively small eligible populations for targeted clinical trials.
Any therapy, whether a conventional cytotoxic chemotherapy or a targeted novel agent, will fail to have any clinical benefit if it does not achieve sufficient drug concentrations at the target tissue. The issue of poor drug delivery, as a result of the blood–brain barrier, may be a major reason for failure in both adult and pediatric diffuse HGG. Studies in adult glioblastoma have investigated drug levels of targeted agents in post-exposure resected tumors and have demonstrated both inter- and intratumoral variation of tumor drug concentrations, confirming concerns of poor drug delivery as a cause of failure.80–82 A phase II study of the ErbB inhibitor lapatinib in children with refractory CNS tumors has reported low intratumoral drug concentrations (10%–20% of simultaneous plasma sample) similar to results in an adult glioblastoma.82,83 To date, direct measurement of tumor drug concentrations in DIPG has not been reported, and it is postulated that the blood–brain barrier is more intact and hence a greater barrier than in other nonbrainstem CNS tumors. As promising agents with a strong biological rationale emerge, it is essential that the ability to achieve required target drug concentrations is studied in both accurate preclinical models and, if appropriate, confirmed in proof of mechanism clinical trials measuring tumor drug concentrations in post-biopsy/surgical resection.
Recent efforts to establish patient-derived pHGG cell cultures and orthotopic xenograft models29,56,84 have enabled preclinical testing. In addition to confirming the relative insensitivity of pHGGs to traditional chemotherapies, such efforts in DIPG have demonstrated the potential therapeutic efficacy of epigenetic modifying agents targeting histone demethylases and histone deacetylases.29,30 Both classes of agents promote restoration of histone-3 K27 trimethylation through differing mechanisms, and demonstrate synergy when used in combination.29 A phase I clinical trial of the histone deacetylase inhibitor panobinostat in children with DIPG was set to open in late 2015 within the Pediatric Brain Tumor Consortium (PBTC 047).
These studies highlight the therapeutic implications of targeting epigenetic lesions induced by histone mutations. However, the impact of K27M and G34R/V on the global chromatin landscape is still largely unknown. For example, it is highly likely that K27me3 loss in K27M causes downstream consequences on other histone marks or chromatin machinery which can be targeted pharmacologically. Evidence for this comes from work showing decreased DIPG proliferation when inhibiting menin, a member of the trithorax group, which is a complex that antagonizes K27me3, depositing polycomb repressive complex.31 This suggests that mechanistic studies of how K27M and G34R/V mutations impact the global epigenetic landscape will provide further insight into prognostic and rational, targeted therapeutic strategies in DIPG.
The existing trial paradigms (ie, separate DIPG vs supratentorial HGG studies, and even pediatric vs adult HGG studies) are being greatly challenged by molecular subgrouping. For example, future trials targeting K27M mutant tumors should include patients regardless of anatomical location.39 On the other hand, given that IDH1 mutant tumors are much more common in adults, the inclusion of IDH mutant tumors on pediatric studies without a specific rationale generally makes little sense (and perhaps should be included in a separate stratum of the adult trials). While BRAF V600E represents an attractive target in HGG, the design of appropriately powered clinical trials with BRAF V600E inhibitors in the upfront setting will be daunting, especially as the enthusiasm for randomized studies with a control arm will be low. The specific challenges relate to the very small patient numbers, as well as the fact that BRAF V600E mutant tumors include secondary HGG arising via malignant transformation of a low-grade lesion, and tend to have a more favorable outcome compared with other HGG.85 As a result, our ability to plan and conduct adequately powered studies in this population requires international collaboration among clinical centers and needs to somehow incorporate first-world molecular diagnostic capabilities for patients in third-world countries. For patients with recurrent BRAF V600E mutant tumors, including HGG, the feasibility of clinical trials is far greater, and such studies are already under way (NCT01677741, NCT01748149). It is expected that these trials will generate valuable preliminary data on the efficacy of BRAF V600E targeted therapies in HGG, including durability of response.
Conclusions
The general challenges for future clinical trial design in pediatric HGG are 4-fold: (i) lack of currently actionable alterations in a large proportion of patients, (ii) intratumor heterogeneity and molecular pathway redundancy, (iii) issues with drug delivery including poor blood–brain barrier penetration of many molecular targeted agents, and (iv) small subsets of patients for each given biology and target expression. While novel clinical trial designs are needed, large-scale studies using adaptive clinical trial designs as proposed for adult glioblastoma86 will only be feasible in the much smaller pediatric population through international collaboration. A more promising design could involve real-time molecular diagnostics ideally involving multiple areas of the tumor (including the contrast-enhancing as well as the nonenhancing, infiltrative components), and a “personalized medicine” approach to custom-select medications with a matching target profile and favorable pharmacokinetics, including blood–brain barrier penetration. Such an approach is currently being explored in a pilot trial testing the feasibility of molecular profiling in adults with recurrent/progressive glioblastoma (Clinical Trials.gov identifier NCT02060890). Another is being performed in children and young adults with newly diagnosed and recurrent DIPGs through the Pacific (Pediatric) Neuro-Oncology Consortium (PNOC). Additional layers of complexity that remain poorly understood but will need to be addressed include intratumoral heterogeneity and clonal evolution at the single cell level,87,88 as well as the role of the tumor microenvironment,60 tumor metabolism,89 and tumor immunology,90 and how to exploit them therapeutically.
Funding
The meeting was supported by The National Brain Tumor Society, The German Cancer Research Centre (DKFZ) and the University of California San Francisco (UCSF) Helen Diller Family Comprehensive Cancer Center.
Acknowledgments
We acknowledge the administrative assistance of Melissent Zumwalt and Nu Truong for meeting logistics.
Conflict of interest statement. None declared.
References
Author notes
Corresponding Author: Chris Jones, PhD, FRCPath, Divisions of Molecular Pathology and Cancer Therapeutics, The Institute of Cancer Research, 15 Cotswold Road, Sutton, Surrey, UK ([email protected]).