-
PDF
- Split View
-
Views
-
Cite
Cite
Anwesha Dasgupta, John R Prensner, Upstream open reading frames: new players in the landscape of cancer gene regulation, NAR Cancer, Volume 6, Issue 2, June 2024, zcae023, https://doi.org/10.1093/narcan/zcae023
- Share Icon Share
Abstract
The translation of RNA by ribosomes represents a central biological process and one of the most dysregulated processes in cancer. While translation is traditionally thought to occur exclusively in the protein-coding regions of messenger RNAs (mRNAs), recent transcriptome-wide approaches have shown abundant ribosome activity across diverse stretches of RNA transcripts. The most common type of this kind of ribosome activity occurs in gene leader sequences, also known as 5′ untranslated regions (UTRs) of the mRNA, that precede the main coding sequence. Translation of these upstream open reading frames (uORFs) is now known to occur in upwards of 25% of all protein-coding genes. With diverse functions from RNA regulation to microprotein generation, uORFs are rapidly igniting a new arena of cancer biology, where they are linked to cancer genetics, cancer signaling, and tumor-immune interactions. This review focuses on the contributions of uORFs and their associated 5′UTR sequences to cancer biology.
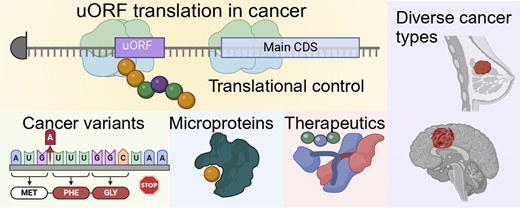
Introduction
Cancer is characterized by uncontrolled cell proliferation that results in the invasion of surrounding tissues and metastasis to distant organs. This complex set of behaviors requires cancer cells to manipulate the cellular mechanisms needed to maintain both the replication of genetic material as well as its processing into the RNAs and proteins that orchestrate cellular activity. Among the fundamental cell processes co-opted in cancer is mRNA translation, which is one of the most energetically expensive processes in the cell and is often dysregulated in malignancies (1–5). While the basic tenets of RNA translation have been investigated in cancer for over 50 years (6,7), technological advances continue to unveil new truths about how cancers alter RNA translation.
Perhaps foremost among these discoveries has been the recent appreciation of upstream open reading frames (uORFs) as a novel player in the realm of cancer and RNA translation. uORFs are characterized by short sequences initiated by a start codon (canonical AUG or non-AUG) within a 5′UTR and terminated by its in-frame termination codon upstream or overlapped with the adjacent canonical coding sequence of an mRNA (Figure 1A) (8,9). uORFs may be extremely small – perhaps only several amino acids – but may also be above 100 codons in length in some cases (10–16). Although uORFs have been observed as a feature of some mRNAs for nearly 35 years (17), they largely eluded deep scientific inquiry in cancer biology until contemporary methods have focused attention on their contributions to gene regulation in cancer.
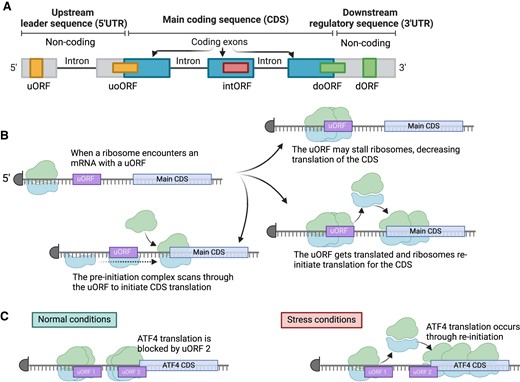
Mechanisms of translation of upstream open reading frames. (A) A schematic overview of the types of non-canonical open reading frames (ORF) observed within messenger RNAs (mRNAs). Here, the annotated coding sequence (CDS) for the mRNA is indicated in blue, with non-coding sequences in gray. Individual boxes indicate where each class of non-canonical ORF would be found on an mRNA transcript. uORF, upstream open reading frame. uoORF, upstream overlapping open reading frame. intORF, internal open reading frame. doORF, downstream overlapping open reading frame. dORF, downstream open reading frame. (B) Possible impacts of ribosome interactions with a uORF, including stalling of the ribosomes, re-initiation of ribosome translation for the main CDS, and scanning through the uORF to the main CDS. (C) A mechanistic paradigm for ATF4 translation in normal and stress conditions. Here, two uORFs are present. In normal conditions, cells re-initiate ATF4 translation but in stress conditions ribosomes are stalled on uORF1 and uORF2, without re-initiation of ATF4 translation. Figure was created with Biorender.com.
In this review, our goal is to consolidate the present knowledge of the impact of cancer on uORFs and their role in the regulation of RNA translation. Our primary emphasis will be on the mechanisms by which uORFs mediate translation initiation in cancer and contribute to the generation of cancer-associated microproteins or peptide products. Along with this focus, we will touch on pertinent examples within the 5′UTR of mRNAs that are relevant to cancer. Lastly, we will summarize the potential clinical relevance of uORFs and paradigms for developing anti-cancer therapies based on uORFs.
Basic mechanisms of mRNA translation
An mRNA transcript consists of a primary open reading frame or primary coding sequence (CDS) in the 5′ to 3′ direction, the 5′ and 3′ untranslated regions (UTRs). mRNA translation is a highly orchestrated complex process that involves numerous ribosome subunits and translation cofactors (such as eukaryotic initiation factors, eIFs) to decode mRNA into amino acids, polypeptides, and ultimately functional proteins (18). While several mechanisms of mRNA translation exist, the most common is a well-described cap-dependent translation initiation model, which posits that the 43S pre-initiation complex (PIC) initiates from the 5′-cap-structure and scans down the mRNA until it identifies a fitting initiation site followed by 60S subunit attachment, to begin translation (19).
This scanning mechanism of translation begins at the 5′ end of the mRNA, at the 7-methylguanosine (m7G) cap structure (18,20,21). Translation is initiated by the assembly of the eIF4F cap-binding complex at the 5′ m7G cap, where the cap-binding protein eukaryotic initiation factor 4E (eIF4E), interacts with eukaryotic initiation factor 4A, an RNA helicase (eIF4A), and eukaryotic initiation factor 4G (eIF4G), a scaffold protein. Poly(A) binding protein (PABP) attaches to the 3′ poly(A) tail interacts with eIF4G, facilitating the circularization of mRNA. The assembly of 43S PIC begins with methionyl initiator tRNA (Met-tRNAi) forming a ternary complex (TC) with guanosine triphosphate (GTP)–bound eukaryotic initiation factor 2 (eIF2). The assembly of the TC with 40S to form the 43S PIC is supported by eukaryotic initiation factors 1, 1A, 3 and 5 (eIF1, eIF1A, eIF3, and eIF5). Following unwinding of the mRNA by eIF4A, eukaryotic initiation factor 4B (eIF4B), and eIF4F, the 43S PIC gets recruited to the m7G-capped 5′ end aided by eIF4G and eIF3 interaction. The PIC starts scanning the mRNA 5′UTR in the 5′ to 3′ direction in an ATP-dependent manner, with partial hydrolysis of the eIF2-bound GTP to GDP in the TC, until it encounters an AUG start codon in the optimal Kozak sequence context. Recognition of AUG initiates hydrolysis of the GTP bound to eIF2, creating a stable 48S PIC (22). The subsequent release of eIF2-GDP is followed by the attachment of the 60S ribosome subunit, facilitated by eukaryotic initiation factor 5B (eIF5B), leading to an 80S initiation complex ready for protein synthesis (22,23). Exceptions to the scanning mechanism are mRNAs that show a complete absence of 5′UTRs (24) or extremely short 5′UTRs (∼12 nucleotides on average), which undergo scanning-free initiation (25).
5′UTRs in gene regulation and cancer
Although conventionally thought not to be translated into proteins, UTRs can uniquely influence translation as a virtue of their structured and unstructured elements (26). Throughout the evolutionary journey from invertebrates to humans, the average length of the 5′UTR has remained mainly stable, at around 53–218 nucleotides (27–29), despite the size of the genome and the length of 3′UTRs experiencing a notable expansion (30,31).
The influence of 5′UTR structured or unstructured components on translation initiation and efficiency in eukaryotic mRNAs is significant (18,23,26,27,29). A recent high-throughput screening study have shown dramatic changes in the efficiency of protein translation based upon synthetic 5′UTR plasmids (32). In higher eukaryotes, individual genes can exhibit a wide variation in 5′UTR lengths (26,28) with humans having the longest average length of 5′UTRs, 218 nucleotides (26,30,33). This substantial variability brings the possibility of enhanced regulation of specific mRNA subsets through sequence dependent structured or unstructured elements. Elements such as pseudoknots, RNA G-quadruplexes, hairpins, upstream AUGs (uAUGs) and uORFs hinder translation (26). Confronted with such 5′UTR elements, a minority of genes may also employ internal ribosomal entry sites (IRESs) to facilitate cap-independent translation initiation.
A growing body of literature has linked the regulation of gene activity in cancer to the 5′UTR region (see prior reviews (34–37)). For example, some gene 5′UTRs regions are structurally altered or mutated, including classic cancer genes such as TP53, where variants modulate its translation and may contribute to tumorigenesis (38,39). Separately, some oncogenes preferentially control G-quadruplexes, such as the c-MYC transcription factor (40). However, perhaps the most active area for oncogenic dysregulation of the 5′UTR is through uORFs. This review presents the current state of understanding for uORF-related dysregulation in cancer, focusing on recent discoveries in the field.
Upstream open reading frames as genic elements
One of the fascinating aspects of mRNA translation, appreciated even 30+ years ago, is that the first AUG codon encountered on an mRNA is not always the one employed for protein synthesis from that particular mRNA (17). This indicates that the scanning mechanism of translation initiation possesses the ability to discriminate among AUGs to initiate the production of proteins.
However, this general perception has become ever more nuanced with technological advances that have shown frequent occupancy of ribosomes on the 5′UTR leader sequences of gene mRNAs. These discoveries have been fueled by increasing utilization of ribosome profiling (Ribo-Seq) as a technique that maps ribosome footprints on RNAs in a transcriptome-wide manner (14,16,41). Ribo-Seq has revealed numerous non-canonical translational events occurring in uORFs that are initiated by either canonical uAUGs or alternative translational initiation sites (non-AUG start codons) (10,42–44). While more than half of all human 5′UTR sequences contain uAUG codons and almost all eukaryotic 5′UTRs contain alternative start codons (10–12,43), transcriptome-wide mapping of RNA translation by Ribo-Seq has generally estimated that only selective uORFs are translated, likely numbering in the ∼3000–15 000 range and existing in ∼10–25% of human mRNAs (8,13,45–48). While highly prevalent, uORFs are therefore not ubiquitously translated, and the biological mechanisms governing why specific uORFs are translated and others are not, remains unclear.
It is commonly believed that the primary function of uORFs is to inhibit the translation of the main CDS as they capture the scanning ribosome during the initial phase of translation (11,12). uORFs are also known for their role in controlling protein expression amidst cellular stress responses (15,49–52). However, the regulatory impact on translation rates of uORF-associated downstream CDSs depends on specific characteristics of the transcript, such as the length, number, position, and peptide sequence of individual uORFs, as well as the sequence context surrounding the initiation and termination codons of the uORFs (53,54).
Features of uORF translation
uORFs are able suppress translation through various mechanisms (11,44,55,56). Despite this overarching inhibitory function, there are particular circumstances in which uORFs allow the ribosome to resume scanning and reinitiate translation downstream (15,57–59). Upon encountering a uORF the following three situations are possible: (a) the uORF gets translated but CDS translation is inhibited because recognition of the uORF stop codon can activate non-sense mediated decay (NMD), (b) the uORF gets translated, and with a certain probability, downstream CDS translation is re-initiated, (c) the PIC simply scans through the uORF (termed leaky scanning) (Figure 1B). Determining which of these outcomes will prevail is influenced by a multitude of cellular conditions, making it challenging to predict without a detailed analysis of specific cases. While it has been proposed that reinitiating translation at the CDS could allow messenger RNAs (mRNAs) to avoid triggering nonsense-mediated decay (NMD) (60,61), recent findings suggest that, while translation reinitiation is more likely to occur following small uORFs, it does not reliably prevent NMD (62).
Two classic examples of gene regulation mediated by uORFs are apparent in the translational regulation of ATF4 and GCN4 mRNAs. These cases, which are reviewed in depth elsewhere (63), reflect rapid cellular changes in the control of protein synthesis based upon cellular resources. During low-nutrient conditions or cell stress, cells can activate mechanisms such as General Amino Acid Control (GAAC) and Integrated Stress Response (ISR) that reduces widespread protein synthesis through phosphorylation of eIF2α while also activating translation of specific mRNAs like GCN4 and ATF4. The GCN4 mRNA contains four short uORFs (2–3 codons) and the ATF4 mRNA includes at least two uORFs (51,64–66). In both cases, these uORFs serve to impart rapid control of protein synthesis of the GCN4 and ATF4 proteins.
Elegant mechanistic work has delineated clear ribosome behavior for the ATF4 uORFs (Figure 1C). In this case, ATF4 harbors a three-codon long uORF1 and a longer uORF2 that overlaps with the CDS (65). Under regular physiological conditions the proportion of phosphorylated eIF2α is lower, therefore the translation process advances from uORF1 to re-initiating at the start codon of the second downstream uORF2. Conversely, in situations where cells are under stress and the proportion of phosphorylated eIF2α is higher, the translation of uORF1 instead proceeds to re-initiate at the start codon of the CDS. uORF2 overlaps with and ends downstream from the starting point of the ATF4 coding sequence, hence efficient synthesis of the ATF4 protein doesn’t occur unless uORF2 is bypassed due to leaky scanning during cell stress (15,57,65). Upon reaching the stop codon of uORF1, scanning ribosomes must procure a fresh TC in order to re-initiate at a downstream start codon. The duration of time required to obtain a new TC is directly associated with the extent of phosphorylated eIF2α (63). This mechanism represents a rapid stress response to cell nutrient changes, triggering key transcription factors while dampening overall protein synthesis.
Regulation of cancer genes by uORFs
uORFs are prevalent in cancer-associated genes (13,15,44). Hence, the role of uORF-mediated control on the translation of oncogenic mRNAs has been an intriguing area of research (Figure 2A). mRNA translation can be initiated by cap-dependent or cap-independent mechanisms. Cancer cells often trigger pathways that block canonical cap-dependent translation which endangers their survival. To overcome the blockade, cancer cells exploit alternative cap-independent initiation mechanisms involving 5′UTR elements such as uORFs, IRESs, or non-uAUG initiation (2,67,68). The proto-oncogene c-MYC has an IRES in its 5′ leader, which was detected to carry a mutation associated with increased c-MYC expression in multiple myeloma patients (69). It was also shown to be essential for c-MYC induction, in colorectal cancer (70). Because of this, efforts to target c-MYC by disruption of its IRES have also been explored (71,72).
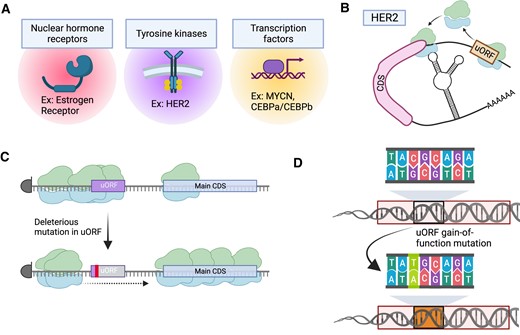
uORF-mediated regulation of oncogenic processes. (A) Example genes and oncogenic processes that have direct regulation by uORFs. (B) A mechanistic model for uORF-based regulation of HER2 including re-initiation of HER2 translation using structured RNA elements in the 3′UTR. (C) Deleterious mutations in uORFs may reduce their ability to stall ribosomes, thereby leading to derepression of main CDS translation. Here, the red line indicates a deleterious mutation. (D) Gain-of-function mutations in the 5′UTR of genes may cause the de novo creation of a uORF through the generation of a novel ATG start codon sequence. Figure was created with Biorender.com.
More broadly, while several early studies have suggested that oncogenes may be enriched in uORFs using in silico analyses (17,73), large-scale systematic studies of uORF translation in cancers are lacking. Nevertheless, it is notable that multiple well-established oncogenes harbor uORFs. One key example is the human epidermal growth factor receptor 2 (HER2). HER2 belongs to the epidermal growth factor receptor family, functions as a tyrosine kinase and is vital for cellular proliferation and hence, the onset of cancer. Approximately 15–30% of breast cancer and 10–30% of gastric or gastroesophageal cancers exhibit an increased expression or amplification of HER2. Its overexpression is also implicated in a variety of other cancers, including ovarian, endometrial, and bladder cancers (74). The mammalian HER2 5′UTR contains a short uORF that has been found to significantly inhibit the HER2 CDS expression (75,76). Key to the strong suppressive effect of this uORF are both the optimal Kozak sequence surrounding its AUG and the extremely short distance (5 nucleotides) between its 3′ end and the primary CDS (75). HER2 translation only occurs due to leaky scanning of the uORF or reinitiation at the CDS after the uORF translation. However, breast cancer cells with HER2 overexpression exhibit the ability to counteract this uORF mediated inhibition via a tumor-cell-specific translation control mechanism. The HER2 3′UTR was shown to harbor a translational derepression element which can bind to RNA-binding proteins Hu Antigen R (HUR) and heterogeneous nuclear ribonucleoprotein A1 (hnRNPA1) to counteract this inhibition (77). In the absence of the 3′UTR, the termination and reinitiation at the HER2 CDS are reportedly inefficient (77). Hence, uORF mediated HER2 mRNA overexpression contributes to the oncogenic potential of the tumor cells (Figure 2B).
The regulatory role of uORFs in oncogenes is further illustrated by the CCAAT/enhancer binding proteins (C/EBPα and C/EBPβ), which are transcription factors vital in the regulation of cell differentiation and proliferation (78). The generation of various C/EBP isoforms from specific C/EBPβ and C/EBPα mRNAs, which begin translation at different sites, leads to isoforms with distinct segments of the amino terminus and diverse capacities in gene regulation and control of cell growth (79). Crucially, a uORF preserved through evolution in both C/EBPα and C/EBPβ mRNAs underpins the regulated initiation at these alternative translation sites (78). Disruption in the translation control that results in aberrant levels of C/EBPα and C/EBPβ isoforms, or the expression of truncated forms, can obstruct terminal differentiation in cells and is linked with the onset of acute myeloid leukemia and breast cancer, respectively (44).
Whether uORFs are universal, or even predominant, in cancer genes remains a topic of debate. Nevertheless, the number of individual cancer genes regulated by uORFs continues to grow (80), including potential isoform-specific regulation in the case of MYCN in neuroblastoma (81) and estrogen receptor in breast cancer (82). Moreover, substantial increases in research on RNA modifications in cancer, particularly RNA methylation as N6-methyladenosine (m6A), have shown that m6A readers, such as YTHDF1 and YTHDF3, may be important in diverse cancer contexts (83,84). While the role of m6A has received significant attention in cancer (85,86), the functional relevance of uORF-specific m6A modifications has not been widely elucidated (35,87).
5′UTR mutations in cancer may impact uORFs
Genetic variation in 5′UTRs is a common feature in cancer, though the role of most of this genetic variation has unclear functional consequences. In the most famous examples, mutations in 5′UTRs can lead to dramatic activation of oncogenes through the creation of transcription factor binding sites in the gene promoter regions (88–90). However, increasing notice has been given to the consequences of 5′UTR mutations that impact uORFs. These mutations can have two conceptual effects: first, an existing uORF may be disrupted; second, a mutation can create a de novo uORF by generating a novel AUG or a non-AUG start codon.
In the first case of mutations that occur in existing uORFs, several large-scale efforts have attempted to estimate these mutations using data from the Cancer Genome Atlas (91). High-throughput screening of uORF-associated variants has proposed a catalogue of about 400 such cancer-related uORF loss-of-function mutations (92). The major limitation to these studies is the lack of a comprehensive statistical model for variant effect and statistical enrichment for variants in uORFs in the local DNA sequence context. Such models are required to the nomination of recurrently-mutated CDS regions (such as MuTect (93)), but efforts in the area of non-coding variants in cancer have only begun to develop rigorous statistical tests which have not been widely applied to uORF sequences (94,95).
While the lack of appropriately designed analyses for variants in uORFs is a major limitation, it is also true that some variants have been functionally investigated and are likely to represent loss-of-function events for the uORF, which in turn may trigger the translational activation of proto-oncogenes (Figure 2C). This situation may occur for uORFs in the EPHB1 and MAP2K6 genes, which code for the ephrin receptor B1 (EPHB1) and a mitogen-activated protein kinase kinase 6 (MAP2K6), respectively (92). Heterozygous loss-of-uAUG mutations have been suggested in a range of genes like the Src family tyrosine kinase BLK proto-oncogene (BLK) in a colon adenocarcinoma, EPHB1 in a breast cancer and a colon cancer xenograft, the Janus kinase 2 (JAK2) in chronic lymphocytic leukemia, and MAP2K6 in colon adenocarcinoma (92). Another mutation was observed in the chromodomain helicase DNA binding protein 1-like gene (CHD1L) within a colon cancer xenograft model. The somatic nature of these mutations related to uORFs was verified for both BLK and CHD1L variants. Meanwhile, the alteration found in MAP2K6 was determined to be an inherited germline variant upon examining control tissues from the patients. These mutations have been associated with heightened levels of protein synthesis for EPHB1 and MAP2K6, indicating that the elimination of a uORF might amplify the translation of the main downstream protein-coding sequence, which could play a role in the development of cancer (92).
Nevertheless, rigorous studies continue to be needed to separate the potential effects of 5′UTR variants on gene regulation via a uORF-related mechanism or an alternative mechanism that impacts the 5′UTR in other ways. A large-scale functional analysis of 5′UTR variants in prostate cancer found many mutations that impacted RNA translation efficiency, yet many were not due to a uORF (96). It is likely that some observed variants in uORFs in pan-cancer analyses of TCGA data impart their major effects through a uORF-independent mechanism.
uORF-modulating variants in cancer
A second scenario is where a cancer variant creates a new uORF through a de novo start codon (Figure 2D). Indeed, the formation of new uORFs by genetic variants is well-documented in various Mendelian disorders. The clinical phenotype arises due to the downregulation of CDS of the Mendelian gene, which is caused by the introduction of a novel repressive uORF when a de novo variant creates a new AUG start codon (97–100). The abundant 5′UTR variants in Mendelian disorders has led to the creation of specific tools, such as UTRannotator (101), to assist in their interpretation, but such tools are lacking in the context of cancer.
Yet, while these genetic events have gained more visibility in Mendelian disorders, their presence in cancer has been observed for at least 20 years in cancer research. The transition from the G1 phase to mitosis within the cell cycle is governed by a set of proteins including cyclins, cyclin-dependent kinases (CDKs) and CDK inhibitor proteins (CDIs), a regulation that is often compromised in cancerous cells (102). A key tumor-suppressing protein called p16INK4a, which is frequently rendered inactive in various cancers, is produced by the cyclin dependent kinase inhibitor (CDKN2A) gene, situated on a routinely deleted chromosome locus 9p21 (103). p16INK4a acts to prevent the assembly of the cyclin dependent kinase (CDK) 4/6 and cyclin D proteins to form the CDK4/6-cyclin D complex, thereby preserving the retinoblastoma protein (Rb) and leading to the halt of cell cycle progression in the G1 phase. Beyond mutations within its coding sequence (103), a sole point mutation in the 5′UTR of the CDKN2A gene creates a novel uORF (104). The translation initiated from this uORF can lead to decreased levels of the CDKN2A protein, which is observed in familial melanoma cases (104).
Similarly, the protein p27KIP1, produced by the CDKN1B gene, acts as a barrier to cell cycle progression from the G1 to S phase, making it essential for cell fate decisions (55). In human cancers, p27KIP1 is often found at reduced levels or mis-localized, but it is rarely subject to mutations, distinguishing it as a non-traditional tumor suppressor (105). CDKN1B translation involves an IRES as well as a uORF. In situations of cellular stress, translation of CDKN1B mediated by the IRES facilitates the expression of p27KIP1 through cap-independent initiation (55,106). Moreover, a four-nucleotide deletion that triggers a frameshift in the uORF of CDKN1B that results in an extended upstream peptide, found to lower re-initiation at the CDS of CDKN1B. This mutation was identified in a patient with tumors in the pituitary gland and the endocrine pancreas (55). The alteration in the uORF causes a reduction in CDKN1B protein levels, likely due to a shortened space between the stop codon of the uORF and the start codon (AUG) of the main coding sequence. This shortening could influence the rate at which ribosomes re-initiate translation on the main coding sequence, thereby increasing susceptibility to tumor formation (54,73,107).
Lastly, a study involving 1134 individuals with neurofibromatosis type 2 (NF2) identified a novel genetic insertion in the 5′UTR region of the NF2 gene in two unrelated participants, with evidence of inheritance in their families. The mutation introduces a new uAUG, potentially leading to a short uORF. This insertion is thought to extend a pre-existing translated uORF in the NF2 5′UTR into an out-of-frame overlapping reading frame, which could reduce NF2 protein translation—a phenomenon consistent with the disease's loss-of-function pathology. Confirmation of this effect awaits further experimental validation (100).
In rare cases, it is possible for uORF-creation by a cancer variant may inversely increase the main CDS translation. For example, a mutation in the excision repair cross-complementation group 5 (ERCC5) gene generates an additional uORF that boosts ERCC5 CDS protein expression post-DNA damage, conferring resistance to DNA-damaging platinum-based chemotherapy (108).
Regulation of cancer genes through non-canonical start sites
Contrary to previous belief that eukaryotic translation predominantly commences at an AUG start codon, multiple other non-AUG start codons are also actively used, albeit less frequently (10,43,48,109). Notably, near-cognate start codons enable translation initiation at varying efficiencies. Although AUG remains the most recognized start codon, CUG is the second most frequently employed for uORFs in the human genome, succeeded by GUG, ACG, and AUU in terms of effectiveness (10,43,48,110). Among these are the near-cognate start codons (CUG, GUG, UUG) which have well-characterized roles in initiation translation in conventional CDSs (109,111), including critical cancer genes such as the proto-oncogene c-MYC (112,113) and Fibroblast Growth Factor 2 (FGF2) (114,115).
As an exemplary case, c-MYC can generate two protein isoforms via different start codons: the c-Myc 2 (p64) isoform originates from an AUG codon, while c-Myc 1 (p67) arises from an upstream CUG codon. Under amino acid scarcity and high cell density, the CUG-initiated c-Myc 1 isoform is favored for translation (116). This isoform possesses enhanced DNA-binding activity and controls genes that suppress cell proliferation (117). Its overexpression can inhibit cell culture growth. Notably, in some cancers such as human Burkitt's lymphomas, c-Myc 1 inactivation is common, indicating that the loss of this CUG-initiated protein may confer a growth benefit to the tumor cells (113).
Likewise, differential usage of AUG or CUG start codons can influence FGF2 as well. Canonically, FGF2 can produce an 18-kDa protein isoform via the AUG start codon, which is typically found in the cytoplasm or secreted. However, using four upstream in-frame CUG codons, FGF2 can also create longer nuclear-targeted protein isoforms. Intriguingly, these alternate CUG-initiated isoforms are unique to transformed cells and have been linked to promoting cell immortality and tumorigenic potential in vitro (114,115,118,119).
Non-canonical start sites in uORFs
Non-AUG start codons are particularly common in uORFs (42), where they may account for up to 50% of initiation codons (45). In one study of neuroblastoma cell lines, non-AUG start codons predominated, with the prevalence of CUG start codons being approximately equal to AUG start codons (120). While the impact of cancer variants that may create a non-AUG initiation codon has not been systematically explored, initial observations suggest that the CUG codon may be the most frequently modified initiation codon (43). Indeed, cancer variants that disrupt a CUG start codon might be more prevalent than those affecting an AUG (43). However, when mutations that disrupt the CUG codon occur, their impact on the translation of the main CDS can vary: sometimes they interfere with the main CDS translation, while other times they lead to the utilization of an alternative, non-AUG start codon within the uORF (43).
The reasons behind the higher prevalence of non-AUG start codons in certain situations, such as cancer, are not well comprehended. Nevertheless, it could be related to widespread alterations in the accuracy of start codon selection in cancer cells. A number of eIFs, such as the cap-binding protein eIF4E, experience dysregulation in cancerous cells. These disturbances in the relative amounts, or stoichiometry, of eIFs can affect the overall precision of translation initiation and the selection of the start codon (42,121–123).
Signatures of uORF regulation by oncogenes
Just as uORFs may regulate oncogenes, oncogenes can also regulate uORFs. Numerous oncogenes, including transcription factors, can attach to the promoter areas of genes, potentially activating gene function before the translation of the main CDS commences (Figure 3A). In addition, many transcription factors can bind directly to RNA, although the significance of this interaction in the context of cancer remains unclear in the majority of instances (124).
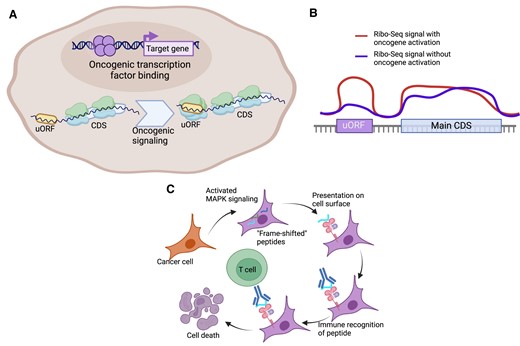
Cancer-directed regulation of uORFs in cancer. (A) Oncogenic signaling via transcription factors or other pathways may result in the accumulation of ribosomes on an uORF, leading to uORF translation. (B) Some oncogenes, such as SOX2, result in a selective increase in ribosome abundance on uORFs using Ribo-Seq. In these cases, the translation of the main CDS may or may not be impacted. The illustration shown here represents a potential Ribo-Seq pattern that would be observed with selective upregulation of uORF translation with the addition of oncogenic signaling. (C) Activation of MAP kinase pathway signaling through oncogenic RAS or BRAF alleles induce ribosomal frame-shifting, particularly in the setting of essential amino acid such as tryptophan starvation. This translation infidelity may produce presented peptide antigens that are recognized by T cells for cancer cell killing. Figure was created with Biorender.com.
Two intriguing examples, however, include the c-MYC and SOX2 oncogenes (Figure 3B). Using an elegant mouse model of SOX2-mediated skin carcinogenesis, Sendoel et al. demonstrated that the translation of uORFs changes significantly upon oncogene initiation (125). Further analyses revealed that these distinctive uORFs coincide with the subsequent main CDS involved in cancer mechanisms, including stem cell pluripotency and Wnt/β-catenin signaling. This suggests that the process of initiating translation during tumorigenesis may selectively target uORFs linked to genes involved in cancer (125). Interestingly, majority of these uORFs initiated with CUG or GUG start codon, subsequently boosting the translational efficiency of the mRNAs related to oncogenes (125).
Likewise, c-MYC can also affect the cancer-specific translation of mRNAs, covering both the main CDSs and uORFs (126,127). Whether this effect is more reliant on the binding of c-MYC to the genomic promoter locus or on the induction of unique RNA-binding proteins is yet to be determined (126). Nevertheless, ectopic expression of c-MYC in a lymphoma model significantly increased the prevalence of uORF translation, particularly in genes with known involvement in cancer-associated pathways such as mTOR signaling and E2F targets (126).
Oncogene induced changes in translation during tumorigenesis
Another potential mechanism for uORF regulation by oncogenes is the inadvertent translation of non-canonical open reading frames leading to significant changes in global translation due to changes induced by oncogenes in the functioning of eIFs. These changes can alter the translation of uORFs globally during tumorigenesis, making certain groups of cancer-related mRNAs resistant to the overall decrease in protein production typically seen in stem-like or cancerous cells. For example, several oncogenes have been implicated in the activity of the translation initiation factor, eIF2A, including AKT (128), ERBB2 (129), WDR4 (130) and SOX2 (125); the eIF2A locus is also amplified in multiple cancers (125). One downstream result of activation of eIF2A in cancer is the translation of non-AUG start codons, which may include known cancer genes such as VHL-alpha (131) as well as non-AUG uORFs in cancer-related mRNAs (125). These results could indicate potential therapeutic approaches targeting eIF2A-mediated translation, or the regulatory effects of uORFs, for the development of new cancer treatments.
When cancer cell lines face a shortage of essential amino acids, such as tryptophan, they frequently exhibit ribosomal frameshifting, a process associated with MAPK pathway activation via oncogenic RAS. Inhibitors of the RAS pathway can reduce this frameshifting, but resistance to these drugs can lead to its recurrence. Nevertheless, the resulting abnormal proteins can be presented on the cell surface, triggering T cell-mediated recognition and elimination of the tumor cells (Figure 3C) (132). This phenomenon of frameshifting is likely to affect uORF translation which could contribute to the unique behavior of the transformed cells, though concrete data addressing this topic remain sparse. Translational reprogramming of BRAF-mutant melanoma cells has similarly been connected to metabolic states and metabolic response to targeted BRAF therapies (133). While the generalizability of these observations to other oncogenes has not been systematically explored, mechanisms of mRNA mis-translation are well-documented in several cellular stress states (134–136), suggesting that this phenomenon is likely present in cancer, though its significance is yet to be established in the context of uORFs.
Cancer-relevant microproteins produced by uORFs
The most well-described role of uORFs is to impact translational regulation on mRNAs; yet this may not be the only role. By virtue of being translated, uORFs may also make polypeptide sequences or small proteins, called microproteins (13,137–139). A subset of these microproteins may operate as bioactive molecules in the cancer cell, directly contributing to cancer biology via the protein product of the uORF rather than through the impact of the uORF on translation of the downstream CDS. Indeed, high-throughput functional screening of uORFs and other non-canonical open reading frames has shown surprisingly high rates of bioactive function (13,140). Although uORF-encoded microproteins have only recently been explored, several key examples have emerged, which collectively demonstrate the capacity for uORF-encoded microproteins to be unique biological actors at the protein level.
Initial work using CRISPR-based screening has demonstrated not only genetic essentiality of some uORF loci in the cancer genome, but also cancer cell survival phenotypes that are rescued by re-introduction of the uORF protein (13,140). One prominent example is the uORF encoded in the 5′UTR of the ASNSD1 gene, alternately termed ASNSD1-uORF or ASDURF (Figure 4A). This uORF encodes a stable protein well-detected in mass spectrometry (141–143). Our recent work in medulloblastoma has shown that the ASNSD1-uORF protein is selectively upregulated by cMYC and MYCN, leading to its essentiality in promoting medulloblastoma cell survival through proteomic regulation of target cell cycle proteins via the prefoldin-like complex (127).
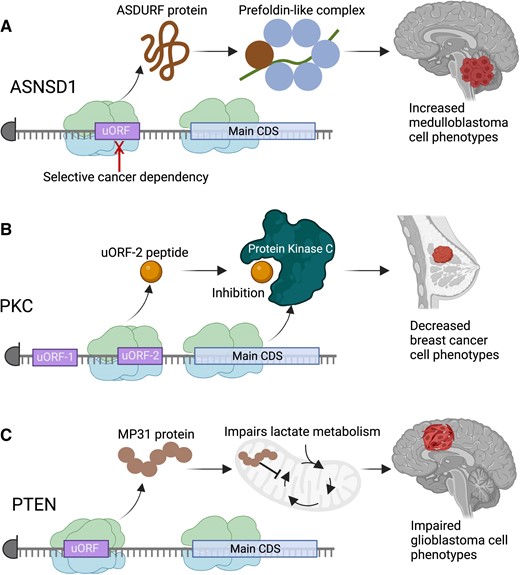
Examples of cancer-involved microproteins. (A) The ASNSD1 gene translates a microprotein from an uORF that has been found with interact with the prefoldin-like complex to drive medulloblastoma cell survival. (B) A microprotein translated from the second uORF in the PKC gene, termed the uORF-2 peptide, is able to inhibit the kinase activity of certain PKC forms, leading to overall antagonism of breast cancer phenotypes by the microprotein. (C) A microprotein translated from the 5′UTR of the PTEN tumor suppressor gene, termed MP31, antagonizes lactate metabolism in glioblastoma, causing a reduction in glioblastoma aggressiveness. The MP31 microprotein is deleted with PTEN deletions in glioblastoma, contributing to increased cancer phenotypes in that setting. Figure was created with Biorender.com.
Additional work in breast cancer has highlighted the ability for the second uORF (uORF-2) in the protein kinase C-eta (PKC-η) gene to encode a microprotein that suppresses breast cancer progression through direct binding and modulation of phosphorylation of PKC-η protein (Figure 4B) (144). By doing so, the uORF-2 encoded peptide impairs breast cancer phenotypes such as cell proliferation and migration (144).
Interestingly, uORFs translated from tumor suppressor genes may also provide an additional mechanism of tumor inhibition independent of the adjacent protein coding CDS. Work in glioblastoma mouse models demonstrated a microprotein from a specific PTEN isoform, termed MP31, that also impaired tumorigenesis via a PTEN-independent mechanism that centered on the ability for MP31 to restrain lactate metabolism (Figure 4C) (145).
With increasing efforts to employ functional genomics to decipher uORFs (146), it is likely that the number of uORFs with microproteins involved in cancer cell biology will continue to increase in the coming years.
uORFs in cancer therapy
uORFs represent a non-traditional source for potential cancer therapies; yet they may also inspire unique views on target discovery efforts. As nucleotide sequences, uORFs may be amenable to RNA-directed therapeutics. For example, uORF-specific antisense oligonucleotides may block translation of the uORF to promote main CDS translation, which may ameliorate disease phenotypes. This approach has already been exploited in neurological diseases such as spinal muscular atrophy (147).
While uORF-encoded microproteins may provide additional cancer target genes, their typically short length is likely to make them difficult to target directly with small molecule inhibitors that require sufficient binding sites in a stably folded protein. Alternatively, uORFs are perhaps more attractive as a possible approach to develop so-called ‘miniprotein’ therapeutics (148). Since miniproteins represent a broad class of small protein scaffolds, one could use these short amino acid sequences to identify peptides with unique biological features for specific target engagement via direct protein binding (149–151). The recent investigation of the uORF-derived MP31 microprotein as an anti-tumor biologic therapy in glioblastoma suggests the potential feasibility of this approach (Figure 5A) (145). Additionally, in principle, miniproteins either derived from uORFs or inspired by uORFs may inform miniprotein therapeutic design, or uORF-derived miniproteins may act as molecular glue in therapeutic uses (Figure 5B). A similar approach has recently been employed to develop a miniprotein therapeutic, termed Omomyc, for the direct targeting of cMYC, which is now in clinical trials (NCT04808362) (152–154).
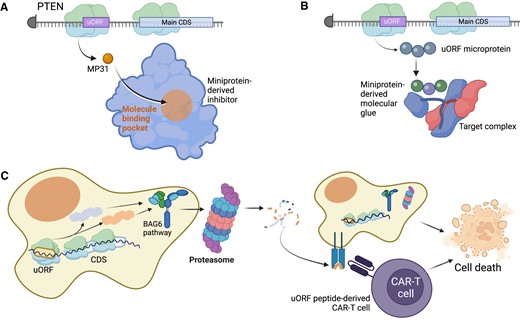
Potential therapeutics derived from uORFs in cancer. (A) In some cases, such as the MP31 microprotein from an upstream open reading frame in the PTEN tumor suppressor genes, direct administration of a uORF-derived miniprotein therapeutic could be clinically beneficial. With MP31, a synthetic MP31 miniprotein would inhibit tumor cell metabolism. (B) Some microproteins may have the capacity to engage in novel protein complex structures, leading to transient binding endogenously. A miniprotein therapeutic developed by modifying the uORF microprotein may have the ability to strengthen those interactions to be a toxic molecular glue for cancer cells. (C) uORF-derived microproteins are often unstable and processed for degradation using the BAG6 pathway, which directs the microprotein to the proteasome for cleavage. Ultimately, the proteasome-degraded peptides are presented on the cell surface through the human leukocyte antigen (HLA) system, which can be targeted through the design of custom CAR-T cells that recognize the uORF-derived peptide. Figure was created with Biorender.com.
However, the most tangible path for uORFs into the clinic is currently their potential application in immuno-oncology. Peptides and proteins derived from non-canonical open reading frames, including uORFs, are highly enriched on human leukocyte antigen-I (HLA-I) alleles as part of the major histocompatibility complex (MHC-I) (34,155–158). By one estimate, these peptides account for approximately 7.5% of the immunopeptidome (159). Since few uORF-derived microproteins are typically observed in mass spectrometry data (160), their enrichment on HLA-I alleles may reflect their intrinsic instability as amino acid sequences (160), or potentially unique cellular processing machinery such as Bcl-2-associated athanogen-6 (BAG6) (161), which may coordinate essential mechanisms of mitophagy for cancer cells (Figure 5C) (162–164).
Regardless of their stability as proteins, the HLA-I peptides derived from uORFs – and other non-canonical ORFs – may elicit strong immune responses, suggesting their use as potential agents in cancer vaccine or chimeric antigen receptor T cell (CAR-T) therapies (Figure 5C) (34,80,165,166). While specific pre-clinical therapeutics have yet to emerge for uORFs, their potential suitability as therapeutic antigens for immnuno-oncology is now well established and may be pursued in the coming years.
Deconstructing mechanisms of uORF translation in cancer
A second potentially clinically-relevant aspect of uORFs in cancer is that they may point toward novel aspects of cancer biology that could be exploited for cancer care. Already, non-canonical ORFs have placed a spotlight on mechanisms of cellular degradation of peptides, such as the BAG6 system which is critical for peptide quality-control (161).
Additionally, the upstream factors that govern uORF translation in a particular cancer may be unique to that cancer (167). For example, numerous eukaryotic initiation factors are dysregulated in cancer, and significant effort has been directed toward understanding the relationship between oncogenes, eIFs, and potential small molecule inhibitors of eIFs (167–170). While most eIFs and other cap-dependent translation factors are not known to have selective functions for uORFs, recent interest in Death-associated protein 5 (DAP5, or eukaryotic initiation factor 4G2 (eIF4G2)) has emerged.
DAP5 is a non-canonical initiation factor necessary for the translation initiation on certain transcripts. It is critical for IRES-mediated translation, initiates solely from a GUG start codon in human and mouse cells (171–175). Recently studies using ribosome profiling, luciferase-based reporters, and mutational analysis have shown that DAP5-mediated translation primarily takes place on mRNAs with lengthy, structure-prone 5′ leader sequences and pervasive uORF translation (176). Indeed, the presence of a uORF is thought to determine the dependence of mRNA translation by DAP5 (177), and thus, in one analysis using Ribo-Seq data to define translated uORFs, 34% of DAP5 target mRNAs possessed a translated uORF compared to 6% of mRNAs lacking a translated uORF (178). Rather than promoting uORF translation, DAP5 encourages preferential translation of the main CDS rather than the uORF, and DAP5 is implicated in the regulation of numerous cancer-associated genes such as kinases, proto-oncogenes and genes implicated in cell migration, adhesion and differentiation (176). As such, DAP5 is crucial for epithelial-to-mesenchymal transition and metastatic phenotypes in breast cancer cells (179). DAP5 expression is selectively upregulated in only certain cancer types, suggesting that the upstream mechanisms governing ribosome translation of uORFs will be heterogenous across cancers. Whether this enables new anti-cancer therapeutic opportunities that focus on modulation of uORF usage through DAP5-directed small molecules may be an emerging area of research (Figure 6A). Already, related therapeutic strategies targeting IRES-mediated translation or the eIF4E/eIF4G complex, which is implicated in this process, are garnering substantial attention (71,180–183).
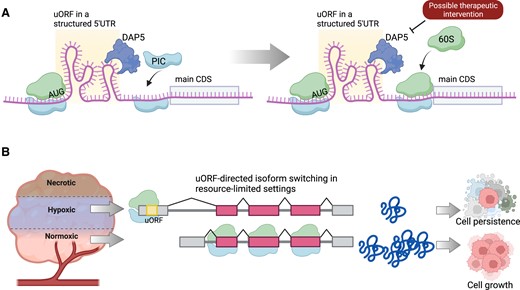
Cancer therapies to target mechanisms of selective uORF translation. (A) uORFs are frequently accompanied by structure 5′UTR sequences that are inhibitory to the translation of the main coding sequence (CDS). DAP5 is a eukaryotic initiation factor that may bypass these uORF 5′UTR structures to recruit the preinitiation complex (PIC) for CDS translation. DAP5-directed therapeutics may be able to shift the efficiency of this process and evaluate uORF translation. (B) Cancer microenvironments, such as hypoxia, may shift transcript isoform usage to include or exclude a uORF, for the long-term benefit of cancer cell survival in different conditions. Therapeutics that modulate this process may lead to a treatment strategy that regulates uORFs in cancer. Figure was created with Biorender.com.
Isoform switching of transcripts reflects another process that may selectively control uORF translation in cancer. Because 5′UTRs strongly influence mRNA translation efficiency, cancer cells are well-known to switch from one isoform to another to enhance translation of key cancer genes (184–186). While at first glance, this process may seem primed to exclude uORFs from isoforms upregulated in cancer – indeed, cancer cells presumably would want to increase translation of oncogenes and avoid repressive uORFs – the full picture is likely more nuanced. Cancer cells must withstand many environmental changes in response to hypoxia, treatment effects, and other situations that result in nutrient stress. In these situations, cancer cells may rapidly include or exclude 5′UTRs to optimize their survival during changing microenvironmental conditions (Figure 6B) (185).
Beyond uORFs: other non-canonical ORFs in cancer
In addition to uORFs, cancer cells translate multiple other types of non-canonical open reading frames (13,140,155,157). These cases may harbor similarities, yet also exhibit significant differences compared to uORF biology. High-throughput CRISPR/Cas9 screening suggests that non-canonical open reading frames of various kinds may serve as genetic dependencies in cancer (13,140). For example, other ORFs that exist on mRNA transcripts, such as downstream ORFs (dORFs), may share a potential for generating immunopeptides for cancer cell surveillance but lack the potentially repressive nature of uORFs on main CDS translation (155). Although more research is needed in this area, dORF translation may enhance main CDS translation (187), although dORF translation is, in general, very rare, likely occurring on <5% of mRNA transcripts (8,160). ORFs found in long non-coding RNAs (lncRNAs) are also likely to produce targetable cancer antigens (156,158), but these ORFs also are better described as a source for novel cancer microproteins with biological roles in disease pathophysiology (140,188).
Conclusions and future directions
Although uORFs have been observed in the human genome for over 30 years, it is only in the past several years that their critical roles in cancer biology have been appreciated. With the dual advent of transcriptome-wide RNA translation profiling and high-throughput functional genomics, uORF translation may now be readily monitored in cancer cells and also experimentally probed in a highly efficient manner. The summation of these efforts is that uORFs have gained initial recognition as potentially key mediators of mRNA regulation in cancer as well as a source of microproteins which may themselves be bioactive or be significant as immunopeptides (Table 1). Moreover, the widespread recognition of uORFs as gene elements now suggests that their presence should be considered during analyses of cancer genomes, as 5′UTR variants have the potential both to inactivate as well as activate uORF-mediated mechanisms of mRNA regulation.
Data availability
No original data was generated for this article.
uORF gene name . | Cancer type . | Observation in cancer . | Proposed cancer function . | Reference . |
---|---|---|---|---|
EPHB1 | Breast cancer | Cancer variant | Not known | 87 |
MAP2K6 | Colon cancer | Cancer variant | Not known | 87 |
BLK | Colon cancer | Cancer variant | Not known | 87 |
JAK2 | Chronic lymphocytic leukemia | Cancer variant | Not known | 87 |
CHD1L | Colon cancer | Cancer variant | Not known | 87 |
PTEN | Glioblastoma | Encodes overexpressed microprotein | Facilitates lactate metabolism in cancer | 136 |
ASNSD1 | Medulloblastoma | Encodes overexpressed microprotein | Proteome regulation with the prefoldin-like complex | 122 |
PKC-η | Breast cancer | Encodes tumor suppressor microprotein | Downregulated in breast cancer | 135 |
C/EBPα & C/EBPβ | Leukemia and breast | Overexpressed in disease | Regulates gene translation | 74 |
MYCN | Neuroblastoma | Overexpressed in disease | Regulates gene translation | 77 |
ER | Breast cancer | Overexpressed in disease | Regulates gene translation | 78 |
HER2 | Breast, gastric, bladder, others | Overexpressed in disease | Supports HER2 translation | 71–73 |
CDKN1B | Pancreas and pituitary gland | uORF-creating cancer variant | Downregulation of CDKN1B tumor suppressor | 51 |
CDKN2A | Melanoma | uORF-creating cancer variant | Downregulation of CDKN2A tumor suppressor | 99 |
NF2 | Neurofibromatosis | uORF-creating cancer variant | Downregulation of NF2 tumor suppressor | 95 |
ERCC5 | Ependymoma, neuroblastoma | uORF-creating cancer variant | Increase in ERCC5 translation | 103 |
uORF gene name . | Cancer type . | Observation in cancer . | Proposed cancer function . | Reference . |
---|---|---|---|---|
EPHB1 | Breast cancer | Cancer variant | Not known | 87 |
MAP2K6 | Colon cancer | Cancer variant | Not known | 87 |
BLK | Colon cancer | Cancer variant | Not known | 87 |
JAK2 | Chronic lymphocytic leukemia | Cancer variant | Not known | 87 |
CHD1L | Colon cancer | Cancer variant | Not known | 87 |
PTEN | Glioblastoma | Encodes overexpressed microprotein | Facilitates lactate metabolism in cancer | 136 |
ASNSD1 | Medulloblastoma | Encodes overexpressed microprotein | Proteome regulation with the prefoldin-like complex | 122 |
PKC-η | Breast cancer | Encodes tumor suppressor microprotein | Downregulated in breast cancer | 135 |
C/EBPα & C/EBPβ | Leukemia and breast | Overexpressed in disease | Regulates gene translation | 74 |
MYCN | Neuroblastoma | Overexpressed in disease | Regulates gene translation | 77 |
ER | Breast cancer | Overexpressed in disease | Regulates gene translation | 78 |
HER2 | Breast, gastric, bladder, others | Overexpressed in disease | Supports HER2 translation | 71–73 |
CDKN1B | Pancreas and pituitary gland | uORF-creating cancer variant | Downregulation of CDKN1B tumor suppressor | 51 |
CDKN2A | Melanoma | uORF-creating cancer variant | Downregulation of CDKN2A tumor suppressor | 99 |
NF2 | Neurofibromatosis | uORF-creating cancer variant | Downregulation of NF2 tumor suppressor | 95 |
ERCC5 | Ependymoma, neuroblastoma | uORF-creating cancer variant | Increase in ERCC5 translation | 103 |
uORF gene name . | Cancer type . | Observation in cancer . | Proposed cancer function . | Reference . |
---|---|---|---|---|
EPHB1 | Breast cancer | Cancer variant | Not known | 87 |
MAP2K6 | Colon cancer | Cancer variant | Not known | 87 |
BLK | Colon cancer | Cancer variant | Not known | 87 |
JAK2 | Chronic lymphocytic leukemia | Cancer variant | Not known | 87 |
CHD1L | Colon cancer | Cancer variant | Not known | 87 |
PTEN | Glioblastoma | Encodes overexpressed microprotein | Facilitates lactate metabolism in cancer | 136 |
ASNSD1 | Medulloblastoma | Encodes overexpressed microprotein | Proteome regulation with the prefoldin-like complex | 122 |
PKC-η | Breast cancer | Encodes tumor suppressor microprotein | Downregulated in breast cancer | 135 |
C/EBPα & C/EBPβ | Leukemia and breast | Overexpressed in disease | Regulates gene translation | 74 |
MYCN | Neuroblastoma | Overexpressed in disease | Regulates gene translation | 77 |
ER | Breast cancer | Overexpressed in disease | Regulates gene translation | 78 |
HER2 | Breast, gastric, bladder, others | Overexpressed in disease | Supports HER2 translation | 71–73 |
CDKN1B | Pancreas and pituitary gland | uORF-creating cancer variant | Downregulation of CDKN1B tumor suppressor | 51 |
CDKN2A | Melanoma | uORF-creating cancer variant | Downregulation of CDKN2A tumor suppressor | 99 |
NF2 | Neurofibromatosis | uORF-creating cancer variant | Downregulation of NF2 tumor suppressor | 95 |
ERCC5 | Ependymoma, neuroblastoma | uORF-creating cancer variant | Increase in ERCC5 translation | 103 |
uORF gene name . | Cancer type . | Observation in cancer . | Proposed cancer function . | Reference . |
---|---|---|---|---|
EPHB1 | Breast cancer | Cancer variant | Not known | 87 |
MAP2K6 | Colon cancer | Cancer variant | Not known | 87 |
BLK | Colon cancer | Cancer variant | Not known | 87 |
JAK2 | Chronic lymphocytic leukemia | Cancer variant | Not known | 87 |
CHD1L | Colon cancer | Cancer variant | Not known | 87 |
PTEN | Glioblastoma | Encodes overexpressed microprotein | Facilitates lactate metabolism in cancer | 136 |
ASNSD1 | Medulloblastoma | Encodes overexpressed microprotein | Proteome regulation with the prefoldin-like complex | 122 |
PKC-η | Breast cancer | Encodes tumor suppressor microprotein | Downregulated in breast cancer | 135 |
C/EBPα & C/EBPβ | Leukemia and breast | Overexpressed in disease | Regulates gene translation | 74 |
MYCN | Neuroblastoma | Overexpressed in disease | Regulates gene translation | 77 |
ER | Breast cancer | Overexpressed in disease | Regulates gene translation | 78 |
HER2 | Breast, gastric, bladder, others | Overexpressed in disease | Supports HER2 translation | 71–73 |
CDKN1B | Pancreas and pituitary gland | uORF-creating cancer variant | Downregulation of CDKN1B tumor suppressor | 51 |
CDKN2A | Melanoma | uORF-creating cancer variant | Downregulation of CDKN2A tumor suppressor | 99 |
NF2 | Neurofibromatosis | uORF-creating cancer variant | Downregulation of NF2 tumor suppressor | 95 |
ERCC5 | Ependymoma, neuroblastoma | uORF-creating cancer variant | Increase in ERCC5 translation | 103 |
Going forward, the momentum on uORF research leads to several key questions for the research community now to grapple with: (i) how frequently do uORFs produce bioactive proteins in addition to, or rather than, regulation on mRNA translation?, (ii) what is the landscape of uORF biology in cancer? and (iii) will uORF-inspired therapeutics be tractable in the clinic? Solving these questions remains daunting, but with the rapid advances in high-throughput molecular techniques, it is likely that the next decade of research on uORFs will bring further distinction to these novel players in cancer biology.
Acknowledgements
We acknowledge the use of BioRender.com for generation of the graphical abstract and figures for this manuscript.
Funding
J.R.P. acknowledges funding from the National Institutes of Health/National Cancer Institute [K08-CA263552-01A1]; Alex's Lemonade Stand Foundation Young Investigator Award [21-23983]; St. Baldrick's Foundation Scholar Award [931638]; Hyundai Hope on Wheels Foundation, the Yuvaan Tiwari Foundation; DIPG/DMG Research Funding Alliance; Book for Hope Foundation; Curing Kids Cancer Foundation, and the Andrew McDonough B+ Foundation; Collaborative Pediatric Cancer Research Awards Program/Kids Join the Fight award [22FN23].
Conflict of interest statement. J.R.P. reports receiving honoraria from Novartis Biosciences. J.R.P. reports being a paid consultant for ProFound Therapeutics.
Comments