-
PDF
- Split View
-
Views
-
Cite
Cite
Samuel Ogden, Ibrahim Ahmed, Shen-Hsi Yang, Paul Fullwood, the OCCAMS consortium, Chiara Francavilla, Andrew D Sharrocks, Oncogenic ERRB2 signals through the AP-1 transcription factor to control mesenchymal-like properties of oesophageal adenocarcinoma, NAR Cancer, Volume 5, Issue 1, March 2023, zcad001, https://doi.org/10.1093/narcan/zcad001
- Share Icon Share
Abstract
Oesophageal adenocarcinoma (OAC) is a deadly disease with poor survival statistics and few targeted therapies available. One of the most common molecular aberrations in OAC is amplification or activation of the gene encoding the receptor tyrosine kinase ERBB2, and ERBB2 is targeted in the clinic for this subset of patients. However, the downstream consequences of these ERBB2 activating events are not well understood. Here we used a combination of phosphoproteomics, open chromatin profiling and transcriptome analysis on cell line models and patient-derived datasets to interrogate the molecular pathways operating downstream from ERBB2. Integrated analysis of these data sets converge on a model where dysregulated ERBB2 signalling is mediated at the transcriptional level by the transcription factor AP-1. AP-1 in turn controls cell behaviour by acting on cohorts of genes that regulate cell migration and adhesion, features often associated with EMT. Our study therefore provides a valuable resource for the cancer cell signalling community and reveals novel molecular determinants underlying the dysregulated behaviour of OAC cells.
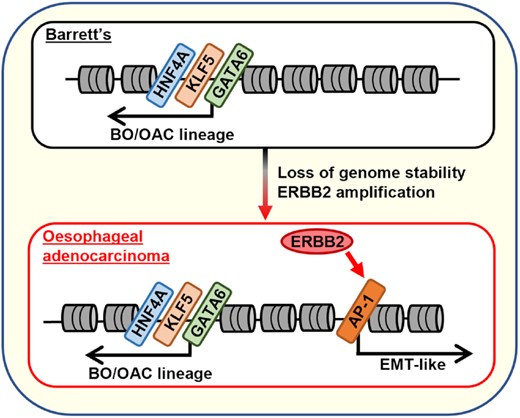
Oncogenic ERBB2 signalling drives the formation of oesophageal adenocarcinoma (OAC) from the underlying Barrett's oesophagus (BO) precancerous state through activating the AP-1 transcription factor which in turn activates an EMT-like gene expression programme.
INTRODUCTION
The RAS–ERK pathway and its upstream regulators is one of the most commonly disrupted pathways in cancer and its hyperactivation through oncogenic mutations drives the tumourigenic phenotype (1,2). Hyperactive RAS–ERK pathway signalling has major impacts in the nucleus on the activity of gene regulatory networks and the underlying regulatory chromatin landscape. Multiple transcription factors and chromatin remodelling proteins have been shown to be direct ERK targets (reviewed in (3)). In common with many other cancers, the RAS–ERK pathway is frequently activated in oesophageal adenocarcinoma (OAC) with multiple components being genomically activated in different patients (60–76%) (4,5). One of the most commonly mutated genes encodes the upstream receptor tyrosine kinase ERBB2, whose locus is amplified in up to 32% of cases (4,5). Patients harbouring these mutations can be treated with the ERBB2 targeting antibody trastuzumab, one of the few targeted therapies available for OAC patients (6; reviewed in 7). Indeed, due to usual late diagnosis and the lack of subsequent targeted therapies available, the current prognosis for OAC patients is poor with 5-year survival rates being only ∼20% (8). However, the molecular and cellular consequences of ERBB2 activation in OAC are poorly understood. Similarly, we know little about the molecular outcomes caused by the activation of alternative RTKs and commonly used downstream pathway components that are mutated in other OAC patients. To bridge this knowledge gap, we recently studied the effects of pharmacological ERBB2 inhibition on the gene regulatory pathways operational in OAC cells as they transition to a drug resistant state (9). In that study we focussed on the programmes that become newly operational following ERBB2 inhibition, and demonstrated a role for the transcription factor HNF4A and also the transcriptional co-activator PPARGC1A in rewiring the metabolic pathways to allow cells to survive and subsequently acquire a resistant state.
In this study, we extended our investigation of ERBB2 function and focussed on the immediate consequences of inhibitor treatment to identify the pathways and processes controlled by ERBB2 in OAC cells. By combining phosphoproteomics, chromatin accessibility mapping and transcriptomics, we uncovered the transcription factor AP-1 as a major mediator of ERBB2 activity in OAC. This is consistent with the finding that some of the major transcription factor targets of RTK signalling are in the AP-1 family. Individual subunits can be phosphorylated and activated (10–12) and/or their levels increased at the transcriptional level following pathway activation (reviewed in (13)). AP-1 itself controls multiple cellular processes (14), with its connections to CCND1 expression (15) linking it to cell cycle control and cell proliferation, one of the key cancer cell attributes (16). However, rather than an expected role in cell proliferation, AP-1 instead mediates ERBB2 signalling effects in OAC through controlling EMT-related properties such as actin cytoskeleton re-organisation and cell migration.
MATERIALS AND METHODS
Cell culture and treatments
OE19, and ESO26 cells were cultured in RPMI 1640 (ThermoFisher Scientific, 52400) supplemented with 10% foetal bovine serum (ThermoFisher Scientific, 10270) and 1% penicillin/streptomycin (ThermoFisher Scientific, 15140122). KYAE1 cells were cultured in 1:1 RPMI 1640:F12 (ThermoFisher, 11765054) supplemented with 10% foetal bovine serum and 1% penicillin/streptomycin. The OAC organoid WTSI-OESO_009 was cultured as described previously (9). HEK293T cells were cultured in DMEM (ThermoFisher Scientific, 22320-022) supplemented with 10% foetal bovine serum. Cell lines were cultured at 37°C, 5% CO2 in a humidified incubator.
Cell growth assays
Crystal violet assays were performed as described previously (9).
Dominant negative FOS over-expression
pINDUCER20-GFP-AFOS (ADS5006) (17) was packaged into lentivirus and OE19, KYAE1 and ESO26 cells were then transduced with lentivirus as described previously (9). Polyclonal cells were selected for 2 weeks using 250 μg/ml G418 (ThermoFisher Scientific, 10131027). Transduced cells were then seeded at 2 × 104 cells/cm2 for subsequent experiments. Twenty-four h later, dominant negative FOS (acidic FOS, aFOS; 18) was induced by doxycycline treatment.
SILAC labelling
OE19 cells were labelled in SILAC RPMI (Life Technologies, 88365) supplemented with 10% dialysed fetal bovine serum (Sigma, F0392) and 1% penicillin/streptomycin for 20 days (at least 10 cell doublings) to ensure complete incorporation of amino acids. Media was supplemented with labelled amino acids: light condition [Lys0 (Sigma, L8662), Arg0 (Sigma, A6969)], medium condition [Lys4 (CIL, DLM-2640-PK), Arg6 (CIL, CLM-2265-H-PK), heavy condition [Lys8 (CIL, CNLM-291-H-PK), Arg10 (CIL, CNLM-539-H-PK)].
Mass spectrometry-based proteomics and phosphoproteomics
Sample preparation: SILAC-labelled OE19 cells were seeded at 1.92 × 104 cells/cm2. In total 2.4 × 107 cells were seeded for light and medium conditions, and 3.16 × 107 cells were seeded for the heavy condition to account for cell death caused by lapatinib treatment. Twenty-four h after seeding, the light condition was treated with DMSO for 2 h, medium condition was treated with 500 nM lapatinib (Selleckchem, S1028) for 2 h and the heavy condition was treated with 500 nM lapatinib for 24 h. Cells were then washed with PBS and lysed at 4°C in ice-cold 1% triton lysis buffer supplemented with protease inhibitors (ThermoFisher Scientific, A32963) and phosphatase inhibitors (5 nM Na3VO4, 5 nM NaF, 5 mM β-glycerophosphate). Samples were prepared as described (Smith et al., 2021). Briefly, a 4-fold excess of ice-cold acetone was added and proteins were precipitated overnight at −20°C. Precipitated protein was solubilised in denaturation buffer (10 mM HEPES, pH 8.0, 6 M urea, 2 M thiourea) and proteins were quantified using a Bradford assay (Pierce, 23200). Three mg of each light, medium and heavy replicate were combined, resulting in 9 mg of total proteins. Cysteines were reduced using 1 mM dithiothreitol (DTT) and alkylated with 5.5 M chloroacetamide (CAA). For proteome analysis alkylated proteins were resolved by SDS-PAGE (8–12%, Invitrogen) and then fixed in gel and visualized with Colloidal Blue staining (Invitrogen, LC6025). Gel lanes were separated into eight slices, minced and destained with 50% ethanol in acetonitrile. Proteins were digested with sequencing grade, modified trypsin (Sigma, TRYPSEQM-RO) followed by quenching with 1% trifluoroacetic acid. For the phosphoproteome, alkylated proteins were digested using Lysyl Endopeptidase® (FUJIFILM Wako Pure Chemical Corporation, 125-05061) and sequencing grade modified trypsin (Sigma, TRYPSEQM-RO) followed by quenching with 1% trifluoroacetic acid. Peptides were purified using reversed-phase Sep-Pak C18 cartridges (Waters, USA) and eluted with 50% acetonitrile. 6 ml of 12% trifluoroacetic acid was added to the eluted peptides and subsequently enriched with TiO2 beads (5 μm, GL Sciences Inc., Tokyo, Japan). The beads were suspended in 20 mg/ml 2,5-dihydroxybenzoic acid, 80% acetonitrile, 5% trifluoroacetic acid and the samples were incubated in a sample to bead ratio of 1:2 (w/w) in batch mode for 15 min with rotation. After 5 min centrifugation the supernatant was collected and incubated a second time with a two-fold dilution of the previous bead suspension. Beads were washed with 10% acetonitrile, 6% trifluoroacetic acid followed by 40% acetonitrile, 6% trifluoroacetic acid and collected on C8 STAGE-tips (Waters, USA) and finally washed by 80% acetonitrile, 6% trifluoroacetic acid. Phosphorylated peptides were eluted using 20 μl 5% NH3 followed by 20 μl 10% NH3 in 25% acetonitrile. Phosphorylated peptides were evaporated to a final volume of 5 μl in a SpeedVac. Concentrated phosphorylated peptides were acidified with addition of 20 μl 0.1% trifluoroacetic acid, 5% acetonitrile and loaded on C18 STAGE-tips. Peptides were eluted from STAGE-tips with 20 μl of 40% acetonitrile followed by 10 μl 60% acetonitrile. Peptides were then reduced to a final, 5 μl volume by SpeedVac and 5 μl 0.1% formic acid, 5% acetonitrile was added. Samples were analysed by LC–MS/MS using a QE HF (ThermoFisher Scientific).
Mass Spectrometry: Purified peptides were analysed by LC–MS/MS using an UltiMate® 3000 Rapid Separation LC (RSLC, Dionex Corporation, Sunnyvale, CA) coupled to a QE-HF (Thermo Fisher Scientific, Waltham, MA) mass spectrometer (19). Mobile phase A was 0.1% FA in water and mobile phase B was 0.1% FA in ACN and the column was a 75 mm × 250 μm inner diameter 1.7 mM CSH C18, analytical column (Waters). Peptides were separated using a gradient that went from 7% to 18% B in 64 min, then from 18% to 27% B in 8 min and finally from 27% B to 60% B in 1 min. The column was washed at 60% B for 3 min and then re-equilibrated for a further 6.5 min. At 85 min, the flow was increased to 300 nl/min until the end of the run at 90 min. Mass spectrometry data was acquired in a data directed manner for 90 min in positive mode. Peptides were selected for fragmentation automatically by data dependent analysis on a basis of the top 8 (phosphoproteome analysis) or top 12 (proteome analysis) with m/z between 300 to 1750Th and a charge state of 2, 3 or 4 with a dynamic exclusion set at 15 s. The MS Resolution was set at 120 000 with an AGC target of 3e6 and a maximum fill time set at 20 ms. The MS2 Resolution was set to 60 000, with an AGC target of 2e5, and a maximum fill time of 110 ms for Top12 methods, and 30 000, with an AGC target of 2e5, and a maximum fill time of 45 ms for Top8 analysis. The isolation window was of 1.3Th and the collision energy was of 28.
Raw files analysis: Raw data were analysed by the MaxQuant software suite (https://www.maxquant.org; version 1.6.2.6) using the integrated Andromeda search engine (20). Proteins were identified by searching the HCD-MS/MS peak lists against a target/decoy version of the human Uniprot Knowledgebase database that consisted of the complete proteome sets and isoforms (v.2019; https://uniprot.org/proteomes/UP000005640_9606) supplemented with commonly observed contaminants such as porcine trypsin and bovine serum proteins. Tandem mass spectra were initially matched with a mass tolerance of 7 ppm on precursor masses and 0.02 Da or 20 ppm for fragment ions. Cysteine carbamidomethylation was searched as a fixed modification. Protein N-acetylation, N-pyro-glutamine, oxidized methionine and phosphorylation of serine, threonine, and tyrosine were searched as variable modifications for the phosphoproteomes. Protein N-acetylation, oxidized methionine and deamidation of asparagine and glutamine were searched as variable modifications for the proteome experiments. False discovery rate was set to 0.01 for peptides, proteins and modification sites. Minimal peptide length was six amino acids. Site localization probabilities were calculated by MaxQuant using the PTM scoring algorithm (21). The dataset were filtered by posterior error probability to achieve a false discovery rate below 1% for peptides, proteins and modification sites. Only peptides with Andromeda score >40 were included.
Data and statistical analysis: All statistical and bioinformatics analyses were done using the freely available software Perseus, version 1.6.2.1. (22), R framework and Bioconductor 23). We removed proteins or phosphorylated peptides that were not detected in all conditions or all biological replicates. We considered proteins or phosphorylated peptides up- or down-regulated if all three biological replicates were enriched by a 1.2 linear fold change (19). Metascape (24) was used for GO analysis. Kinase-substrate enrichment analysis (25) was used for kinase activity predictions.
RT-qPCR
RT-qPCR was performed as described previously (9). Primers used are listed in Supplementary Table S1. Where indicated, Ontarget plus (Horizon Discovery) siRNAs were used to reduce gene expression of transcription factors (FOS, L-003265-00-0005; FOSL1, L-004341-00-0005;) or control non-targeting pool (D-001810-10-05) was added prior to RT-qPCR. Double transfections were performed at day 1 and day 2 after seeding using RNAiMax (Life Technology) with 50 nM of each siRNA. RNA was harvested 2 days after second transfection.
Western blots and immunofluorescence
Western blots were performed as described previously (9). Antibodies used: anti-GFP (Santa Cruz, sc-8334, 1:2000), anti-ERK (Cell Signaling Technology, 4695S, 1:1000), phospho-ERK (20G11, Cell signalling Technology, 4376S, 1:000), alpha-tubulin (Sigma, T9026, 1:1000), anti-JUND (EPR17636, Abcam, ab181615, 1:1000), anti-FOSL2 (OTI1G1, Origene, TA809658, 1:2000).
For immunofluorescence, cells were grown on coverslips and fixed with 3.7% formaldehyde for 15 min at room temperature. Cells were permeabilised for 3 min in 0.5% Triton in PBS−/− (v/v), washed and then blocked in 1% BSA in PBS (v/v) for 1 h. Cells were stained with Alexa Fluor™ 568 Phalloidin (Invitrogen, A12380). Coverslips were mounted onto glass slides using ProLong® Gold and imaged using a snapshot fluorescence microscope (Zeiss) as previously described (26).
Cell migration and adhesion assays
Cell adhesion assays were performed as previously described (27). Briefly, OE19/KYAE-1-dnFOS cells were treated with 1 μg/ml doxycycline or DMSO control for 24 h to induce dnFOS. 200000 cells were then harvested and re-plated for 12 h at 37°C, 5% CO2 to allow adhesion. Plates were gently tapped and washed with PBS to remove floating cells. Adherent cells were fixed with 4% paraformaldehyde for 10 min and stained using 0.1% crystal violet (Sigma-Aldrich, HT90132) for 30 min at room temperature followed by extensive washing with water. Plates were allowed to dry and dye solubilised in 10% acetic acid for 10 min at room temperature with gentle shaking. Absorbance readings were taken at 570 nm on a SPECTROstar Nano Micoplate Reader (BMG LABTECH).
Cell migration assays were performed as previously described (28). Briefly, OE19/KYAE-1-dnFOS cells were treated with 1 μg/ml doxycycline or DMSO control for 24 h to induce dnFOS. 200 000 cells were harvested and plated into 8.0 μm transwell inserts (Corning, 353097) in 250 μl serum-free medium. Wells were filled with 750 μl medium containing 20% FBS, 1 μg/ml doxycycline and 5 ng/uL TGF-ꞵ. After 24 h migration at 37°C, 5% CO2, non-migrating cells were removed by wiping with a cotton swab. Migrated cells were fixed with 4% paraformaldehyde for 10 min and stained using 0.1% crystal violet. Transwell inserts were dried and imaged using a light microscope. Images were quantified using ImageJ software.
RNA-sequencing
dnFOS was induced in OE19-dnFOS (29) and KYAE1-dnFOS cells for 48 h before RNA was isolated and sequenced and analysed as described previously (9). Differentially expressed genes were determined using DESeq2 (30). Approximate FPKMs for plotting purposes were calculated from normalized FPMs output from DESeq2 by dividing the FPMs by the gene length in kilobases (output from featureCounts; 31). Differentially expressed genes with a mean log2(FPKM+1) <1 were excluded from the results.
ERBB2 positive OAC samples (ERBB2HIGH) were determined based on these samples having expression of ERBB2 greater than the median ERBB2 expression +2 SD. To identify genes differentially expressed in ERBB2 positive OAC these samples were compared to non-dysplastic Barrett's oesophagus samples (32).
Metascape (24) was used for gene ontology analysis of differentially expressed genes. Ingenuity Pathway Analysis (33) was used to predict upstream regulators. Gene set enrichment analysis (GSEA) was performed on differentially expressed genes using GSEA v4.0.3 (34), MSigDB Hallmarks v7.2 (35) with the permutation type set to gene set. OAC partial EMT (pEMT) signature genes were obtained from source data from Tyler and Tirosh, 2021 (36). Only genes more specifically expressed in cancer cells relative to the stromal compartment were used.
ATAC-seq analysis
Amplifications were called in OAC patients as described previously using a custom R script (37). T_007, TCGA-M9-A5M8 and TCGA-IC-A6RE were called as having ERBB2 amplifications. Amplifications of ERBB2 in the two TCGA samples were confirmed using cBioPortal (38,39) and visual inspection of the ERBB2 locus in a genome browser. Initial ATAC-seq data processing was performed as described previously (9). A union peakset was formed of all Barrett's oesophagus and OAC patients samples, using HOMER v4.9 mergePeaks.pl -d 250 (40) as described previously (41). Regions amplified in any OAC patient sample were removed using BEDtools v2.27.1 intersectBed (42). Differentially accessible peaks were then identified using featureCounts v1.6.2 and DESeq2 v1.14.1 (FDR < 0.05).
Distal regulatory regions (i.e. non-promoter regions) were defined as described previously (41). HOMER v4.9 38) was used for both de novo and ‘known’ transcription factor motif enrichment analysis. HOMER v4.9 annotatePeaks.pl or the basal extension model (GREAT; 43) were used to annotate peaks to genes. Genome browser data was visualized using IGV v2.7.2 (44). Heatmaps and tag density plots of epigenomic data were generated using deepTools (45). To visualise epigenomic data at peaks containing the AP-1 motif, HOMER v4.9 annotatePeaks.pl -size 200 -m was used.
Bioinformatics
Morpheus (https://software.broadinstitute.org/morpheus/) was used to generate heatmaps. The prcomp function in R v3.6.0 was used for principal component analysis (PCA). Eulerr or ggvenn packages in R v3.6.0 were used for generating Venn diagrams.
Datasets
All data was obtained from ArrayExpress, unless stated otherwise. Human tissue RNA-seq data was obtained from: E-MTAB-4054 (31) and the OCCAMS consortium (European Genome-Phenome Archive, EGAD00001007496). Human tissue ATAC-seq data was obtained from: E-MTAB-5169 (17), E-MTAB-6751 (41), E-MTAB-8447 (37) and The Cancer Genome Atlas OAC ATAC-seq data were obtained from the GDC data portal (portal.gdc.cancer.gov; (46)).
OE19 HNF4A and H3K27ac ChIP-seq was obtained from: E-MTAB-10319. OE19 lapatinib ATAC and RNA-seq was obtained from E-MTAB-10302, E-MTAB-10304. Lapatinib treatment of WTSI-OESO_009, ESO26, KYAE1 and NCI-N87 cells ATAC-seq was obtained from: E-MTAB-10306, E-MTAB-10307, E-MTAB-10310, E-MTAB-10313 (9). OE19 siERBB2 ATAC-seq and RNA-seq was obtained from: E-MTAB-8576, E-MTAB-8579. KLF5 ChIP-seq data was obtained from E-MTAB-8568 (37).
Data access
OE19 (28) and KYAE1 dnFOS RNA-seq have been deposited at ArrayExpress (E-MTAB-10334).
The mass spectrometry proteomics data in Thermo Scientific's *.raw format have been deposited to the ProteomeXchange Consortium via the PRIDE (47) partner repository with the following dataset identifiers: Project Name: ERBB2 signalling and functions in oesophageal cancer. Project accession: PXD029180.
RESULTS
Phosphoproteomic analysis of ERBB2 signalling in OAC cells
To uncover the signalling pathways and downstream targets controlled by oncogenic ERBB2, we treated OE19 OAC cells (which contain ERBB2 locus amplification; 48) with the ERBB2 inhibitor lapatinib for 2 h (to study immediate effects) and 24 h (to study subsequent/longer term effects) and analysed changes in the phosphoproteome by SILAC-based quantitative mass spectrometry (49) (Figure 1A). Changes in protein abundance and phosphorylation levels were determined by calculating the ratios of medium (2 h lapatinib) and heavy (24 h lapatinib) cell culture conditions to the light condition (control cells). We detected a total of 3366 phosphorylated sites and 2318 proteins, combining to produce 1595 phosphorylated proteins (Supplementary Figure S1A; Tables S2 and S3). The majority of the detected phosphorylated sites (median Andromeda score: 91.2; Supplementary Figure S1B) were on serine residues (88%) and only 13% of phosphopeptides contained more than one phosphorylated amino acid (Supplementary Figure S1C), consistent with previous work (19). The replicates from each of the experiments clustered together although one of the 24 hour lapatinib treated samples showed lower reproducibility in the proteome (Supplementary Figure S1D). At the total protein level, roughly equal numbers of proteins were up- and down-regulated (at a >1.2 fold threshold) at each time point of lapatinib treatment (Supplementary Figure S1E). However, as might be expected, reductions in phosphorylation events were more common than increases following lapatinib treatment, with 367 (after 2 h) and 467 (after 24 h) proteins showing decreased phosphorylation (corresponding to 510 and 689 phosphorylated sites respectively) (Supplementary Figure S1E). Changes in protein level expression after 24 h generally correlated well with the changes we observed at the mRNA level in RNA-seq analysis after 24 h (Supplementary Figure S1F, left). Such correlation was lacking if protein expression at 2 h was compared to the 24 h RNA expression changes (Supplementary Figure S1F, right). Overall, these results reveal extensive rewiring of the phosphoproteome following ERBB2 inhibition.
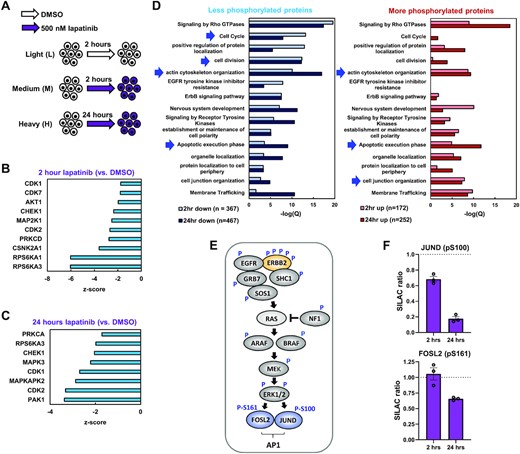
(A) Schematic of mass spectrometry experimental outline. SILAC labelled OE19 cells were treated with DMSO for 2 h, or 500 nM lapatinib for 2 and 24 h. (B and C) Kinase set enrichment analysis predicted changes in kinase activity in OE19 cells treated with 500 nM lapatinib for (B) 2 h or (C) 24 h, relative to DMSO vehicle control. (D) GO analysis of genes encoding proteins that were differentially phosphorylated in OE19 cells following 2 or 24 h lapatinib treatment relative to DMSO control. Arrows highlight terms discussed in the text. (E) Summary of phosphorylation site (P) changes after 2 and/or 24 h in proteins downstream from ERBB2 in the RAS–ERK pathway. (F) SILAC ratios of the peptides containing the indicated phosphorylated residues in OE19 cells treated with 500 nM lapatinib for the indicated timepoints, relative to DMSO vehicle control.
To gain insights into the impact of ERRB2-mediated phosphorylation events we analysed the local sequence context of the phosphorylated sites to determine the likely upstream kinases affected. Downregulation of the activity of multiple predicted kinases was revealed from the enriched motifs showing reduced phosphorylation, including elements of the signalling pathway through ERK, with MAP2K1 (MEK1), RPSP6KA1 (RSK1) and RPS6KA3 (RSK2) down after 2 h (Figure 1B) and MAPK3 (ERK1) down at 24 h (Figure 1C). Cyclin-dependent kinase activity including CDK1 and 2 were downregulated at both time points (Figure 1B and C), consistent with the loss of cell proliferation observed following lapatinib treatment of OE19 cells (9). We also examined the molecular and cellular processes that are likely affected, by performing Gene Ontology (GO) term analysis of proteins showing decreased or increased phosphorylation following ERBB2 inhibition (Figure 1D). Decreased phosphorylation was associated with cell cycle regulation and actin cytoskeleton organisation at both time points. Actin filament based processes were associated with increased phosphorylation as was cell junction organisation. Terms associated with apoptosis were associated with both hyper and hypophosphorylated proteins, which is consistent with the cell death observed upon lapatinib treatment of OE19 cells (9).
Subsequent analysis of the phosphorylation events in the canonical pathway downstream from ERBB2, revealed multiple changes to pathway components including phosphorylation sites on ERBB2 itself (Figure 1E; Supplementary Figure S1G; Supplementary Table S3). Several of the sites lost following ERBB2 inhibition are known to be important for controlling ERBB2 activity either positively (Y847; referred to as Y882 in (50)) or negatively (T671; referred to as T677 in (51)). Interestingly, several downstream transcriptional regulatory proteins show decreased phosphorylation including FOXK1/2, HMGA1, ZNF609 and the AP-1 components JUND and FOSL2 (Figure 1E and F; Supplementary Table S3). Importantly, little change in total levels of JUND and FOSL2 was observed (Supplementary Figure S1H) confirming this change was at the post-translational modification level. In the case of JUND, the downregulated site (S100) is known to be important for ERK-mediated activation of its activity (52). These targets provide potential links from ERBB2 to downstream changes in gene expression.
Inhibition of ERBB2 activity is therefore associated with large changes in the cellular phosphoproteome, including multiple downstream components of the RAS–ERK pathway. Phosphorylation of several transcriptional regulators, including AP-1, is disrupted and multiple protein targets of ERBB2 signalling are involved in the key cellular processes of cell division, actin cytoskeleton organisation and apoptosis. Thus, we generated a picture of the molecular events and associated cellular processes controlled by ERBB2 in OAC.
ERBB2 signalling converges on AP-1 transcription factors in ERBB2-positive OAC cells
Having established the signalling pathway changes elicited by ERBB2 inhibition, we next interrogated the nuclear consequences of ERBB2 inhibition by examining its influence on the accessible chromatin landscape. Previously, we performed ATAC-seq analysis to uncover accessible chromatin regions, which revealed the regulators and processes induced following ERBB2 inhibition that are important for drug resistance (9). Here we analyse the consequences of ERBB2 inhibition in terms of downregulated regulatory activities by focussing on chromatin regions that close following ERBB2 inhibition. We identified 1481 regions opening and 824 regions closing (>2-fold linear fold change, FDR < 0.05) following 24 h of lapatinib treatment (9; Figure 2A). Importantly, there was a large overlap with chromatin accessibility changes elicited by genetic depletion of ERBB2, which is particularly noticeable in the closing regions following more extended lapatinib treatment for 7 days (Supplementary Figure S2A–C). These chromatin accessibility changes following ERBB2 downregulation or inhibition correlated with changes in histone H3K27ac levels, indicating that chromatin closing is associated with the loss of this activating mark and vice versa for chromatin opening (Figure 2A; Supplementary Figure S2D). KLF transcription factor binding motifs are enriched in both opening and closing regions (Figure 2B and C; Supplementary Table S4A and B), consistent with the observation that we can detect KLF5 binding in both sets of regions (Figure 2A). Regions exhibiting increased opening, showed enrichment for motifs recognised by a set of transcription factors such as HNF4A and GATA4 that are usually expressed in gastrointestinal tissues (Figure 2B; Supplementary Table S4B). These motifs are also observed as cells acquire inhibitor resistance at later treatment time points (9). Indeed, these regions also exhibit increased HNF4A binding following lapatinib treatment (Figure 2A). In contrast, the motif recognised by AP-1 transcription factors (TGAC/GTCA) is the dominant sequence found in regions that close following ERBB2 inhibition (∼55% of regions; Figure 2C; Supplementary Table S4A), with motifs for ETS transcription factors being the next most common occurrence (∼25%; Figure 2C; Supplementary Table S4A). HNF4A motifs are lacking, consistent with the low levels of HNF4A binding observed in the closing regions (Figure 2A). Similar results were obtained when ERBB2 was depleted using siRNA (Supplementary Figure S2D–F). Both AP-1 and ETS transcription factors are established transcriptional and direct post-transcriptional targets of ERK, one of the key downstream kinases activated by ERBB2 signalling (53). Importantly, the AP-1 motif was also identified as the top scoring motif in chromatin regions that closed in response to lapatinib treatment in a range of additional gastro-oesophageal adenocarcinoma cells lines and an OAC organoid which harbour ERBB2 amplifications (Figure 2D). All these OAC-derived cell systems have previously been shown to be lapatinib sensitive (9).
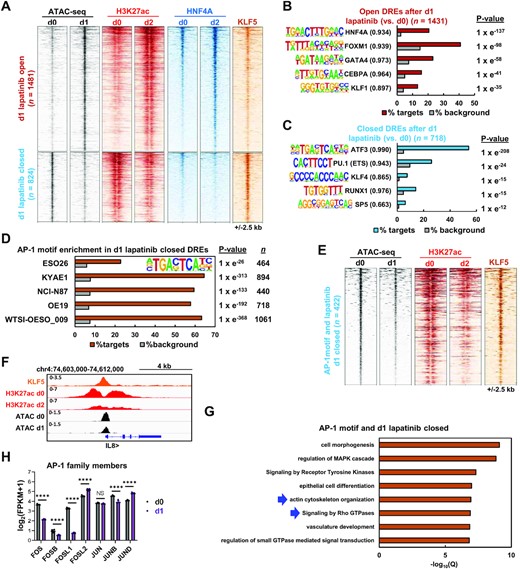
(A) Heatmap of differentially open or closed ATAC-seq peaks (FDR <0.05 and 2 fold change) in OE19 cells treated with 500 nM lapatinib for 1 day. ATAC-seq, HNF4A ChIP-seq and H3K27ac ChIP-seq data is shown for OE19 cells treated with lapatinib for the indicated timepoints (d0-d2). KLF5 ChIP-seq data from untreated OE19 cells is also shown. (B and C) De novo transcription factor motif enrichment at differentially (B) open or (C) closed distal regulatory elements (DREs) in OE19 cells treated with 500 nM lapatinib for 1 day. Motif match score to called transcription factor is shown in brackets. (D) AP-1 transcription factor motif enrichment in differentially closed DREs in ERBB2 positive gastro-oesophageal cell lines or organoid (WTSI-OESO_009) treated with 500 nM lapatinib for 1 day. (E) Heatmap of differentially closed chromatin regions containing the AP-1 motif in OE19 cells following 500 nM lapatinib treatment for 1 day (d1). ATAC-seq, H3K27ac ChIP-seq data is shown for OE19 cells treated with lapatinib for the indicated timepoints (d0-d2). Parental OE19 KLF5 ChIP-seq data is also shown. (F) Genome browser view of ATAC-seq data and ChIP-seq data (KLF5 and H3K27ac) at the indicated times of lapatinib treatment (d0, d1 or d2) highlighting the IL8 locus. (G) GO analysis of genes annotated to 1 day lapatinib differentially closed peaks that contain the AP-1 motif. Peaks were annotated to genes by the basal extension model using GREAT, and annotated genes were then analysed using Metascape. (H) mRNA expression from RNA-seq data of OE19 cells treated with lapatinib. d0 – DMSO control, d1 – 1 day lapatinib **** P < 0.0001.
Given the dominance of the AP-1 motif, we focussed on the closing regions containing AP-1 motifs. These regions show decreased H3K27ac following lapatinib treatment and are generally co-bound by KLF5 (Figure 2E), a factor we previously implicated in OAC (37). The IL8 locus is one such example (Figure 2F). Again, similar findings were made in AP-1 motif containing regions identified from ERBB2 depleted cells (Supplementary Figure S2G). These regions are associated with genes enriched in GO terms related to actin cytoskeleton organisation and signalling by Rho GTPases (Figure 2G). These terms are consistent with the processes affected at the post-translational level following ERBB2 inhibition (Figure 1D) and highlight a potential role for AP-1 in this context. Importantly, many AP-1 family members including FOS, FOSL1 and JUNB show downregulation in OE19 cells at the RNA level following either ERBB2 inhibition by 24 h of lapatinib treatment (Figure 2H) or ERBB2 depletion (Supplementary Figure S2H). This phenomenon is also observed in OAC organoids where FOS and FOSL1 expression is vastly reduced upon lapatinib treatment (Supplementary Figure S2I).
Collectively, these data demonstrate that pharmacological or genetic inhibition of ERBB2 signalling leads to substantive chromatin closing and opening. The regions that lose accessibility are associated with AP-1 motifs, implying a loss of AP-1 activity. Indeed, reductions in AP-1 transcription factor expression following ERBB2 inhibition is consistent with this conclusion, coupled with the reductions in AP-1 component activating phosphorylation events we observe.
AP-1 activity is elevated in ERBB2 positive OAC patients
Given the links we uncovered between ERBB2 signalling and AP-1 in our cell line models, we sought evidence for elevated AP-1 activity in OAC patients with tumours containing ERBB2 amplifications. Our previous work based on cell line models and a limited number of patient OAC samples, used ATAC-seq to implicate AP-1 in OAC (17,19). To more precisely study the potential role of ERBB2 in signalling through AP-1 in OAC, we compared the accessible chromatin landscape of three ERBB2 amplified OAC tumours to Barrett's oesophageal samples (the precursor to OAC) and nine OAC samples lacking such amplifications (Supplementary Figure S3A). Using the three ERBB2 amplified OAC samples, we called differentially open peaks compared to Barrett's oesophagus (2X linear fold change, FDR < 0.05) and identified 1253 more accessible and 679 less accessible regions (Figure 3A; Supplementary Table S5C and D). The more accessible regions showed increased accessibility when compared to other OAC samples, suggesting an influence of ERBB2 amplification (Figure 3A; Supplementary Figure S3B). Motif enrichment analysis identified the AP-1 motif (represented by FRA1) as the top scoring motif in the regions showing increased accessibility in ERBB2 amplified OAC with ∼30% of regions containing this motif (Figure 3B; Supplementary Table S5F). Conversely, the closing regions showed an enrichment for CTCF as the top scoring motif (Figure 3C; Supplementary Table S5F). Furthermore, similarities between motifs identified in differentially accessible regions in ERBB2-positive patients with those identified in differentially accessible regions following ERBB2 inhibition/depletion in vitro were uncovered (Figure 3D). Clustering of the enriched motifs revealed the close concordance of AP-1 motif association with more accessible regions in ERBB2-positive OAC cancer samples and regions that closed in OE19 cells after ERBB2 inhibition (Figure 3D; Supplementary Table S5G). Conversely, FOX and GATA factors showed reciprocal behaviour and were more enriched in regions more open in Barrett's oesophagus and which opened following ERBB2 inhibition in OE19 cells (Figure 3D; Supplementary Table S5G). GO term analysis revealed that genes associated with opening regions were enriched for a variety of biological processes related to epithelial cell biology, but also ‘signalling by receptor tyrosine kinases’ and ‘actin cytoskeleton organization’ (Figure 3E). The former would be expected due to the influence of amplified ERBB2, while the latter process is in line with the changes observed in the phosphoproteome (Figure 1D).
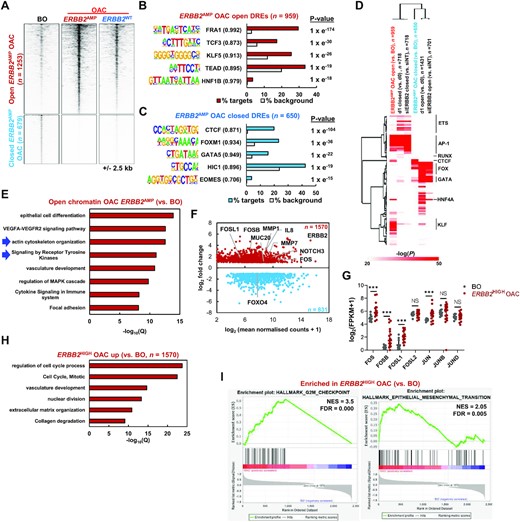
(A) Heatmap showing ATAC-seq signal at differentially open or closed (FDR < 0.05 and 2 fold change) chromatin regions in OAC tumours harbouring ERBB2 amplifications (ERBB2AMP OAC) relative to Barrett's oesophagus (BO) tissue. Data for OAC tumours without ERBB2 amplifications (ERBB2WT OAC) is also shown. (B and C) De novo transcription factor motif enrichment at differentially (B) open or (C) closed distal regulatory elements. Motif match score to called transcription factor is shown in brackets. (D) Heatmap of Homer ‘known’ transcription factor motif enrichment (log10P > 20 in one condition) in chromatin regions closing or opening in OE19 cells with lapatinib (d1) or siERBB2 treatment or in patients harbouring ERBB2 amplifications (ERBB2AMP) compared to Barrett's oesophagus (BO) patients. (E) GO analysis (GREAT) of genes annotated (basal extension model) to differentially open chromatin regions in ERBB2AMP OAC relative to BO. (F) MA plot of differentially expressed genes (DEGs) in ERBB2HIGH OAC patient samples relative to Barrett's oesophagus tissue. Red – genes up-regulated in ERBB2HIGH OAC; blue – genes down-regulated in ERBB2HIGH OAC. DEGs were defined by FDR < 0.05, 2× fold change and FPKM > 1. (G) mRNA expression of AP-1 family members in Barrett's oesophagus (BO) and ERBB2HIGH OAC patient tissue. (H) GO analysis (Metascape) of genes up-regulated in ERBB2HIGH OAC relative to BO tissue. (I) Enrichment plots from GSEA on differentially expressed up- and down-regulated genes in ERBB2HIGH OAC relative to BO. NES – normalized enrichment score.
To further examine the molecular changes in OAC samples containing high levels of ERBB2 expression (defined as >2SD above the median level), we compared their RNA-seq profiles with those found in Barrett's oesophagus patients. PCA analysis clustered each type of sample together (Supplementary Figure S3C) and we uncovered 1570 upregulated and 831 downregulated genes (Figure 3F; Supplementary Table S5A and B). Among the upregulated genes we identified the AP-1 family genes FOS, FOSB and FOSL1 (Figure 3F and G). GO term analysis revealed an increase in cell cycle-associated genes as might be expected for cancer cells (Figure 3H) and decreases in genes associated with various metabolic processes (Supplementary Figure S3D). However, we also identified ‘collagen degradation’ as an enriched term associated with up regulated genes, and further analysis of our ATAC-seq data revealed prominent peaks in the intragenic region associated with the MMP7 and MMP20 genes encoding matrix degrading enzymes (Supplementary Figure S3E). RNA-seq analysis revealed upregulation of genes encoding several MMPs in these OAC samples including MMP7 (Figure 3F; Supplementary Figure S3F). Finally, we looked for Gene Set enrichments in our data and again identified various cell cycle terms associated with the upregulated genes, but also ‘epithelial to mesenchymal transition (EMT)’ (Figure 3I; Supplementary Figure S3G). Metabolic processes were again associated with downregulated genes (Supplementary Figure S3G).
Collectively, these data therefore support a potential functional role for AP-1 in OAC patient samples exhibiting high level ERBB2 expression, both from upregulation of its constituent subunits and the appearance of its binding motif in opening regions of chromatin. Furthermore, genes associated with biological processes focussed around cell cycle, actin cytoskeletal rearrangement, ECM degradation and EMT are prominent in these OAC samples, suggesting a potential link between these molecular and gene expression events.
AP-1 regulates processes associated with EMT in ERBB2 positive OAC cells
Our ATAC-seq and RNA-seq data from OAC patients and cell lines and accompanying phosphoproteomic data, all point to a potentially important connection between AP-1 and the OAC cell phenotype driven by ERBB2. To determine how AP-1 affects OAC function, we inhibited AP-1 function by inducibly overexpressing a dominant-negative form of FOS (dnFOS; Figure 4A) (18) in OE19 cells. dnFOS expression gave the expected downregulation of the AP-1 target gene MMP1 (Supplementary Figure S4A). However, unexpectedly, given previous links between AP-1 and cell cycle control (15; reviewed in 14), there was little effect on cell growth even 6 days after dnFOS induction (Figure 4B). Similarly, dnFOS barely affected the growth of two other ERBB2 positive OAC lines (ESO26 and KYAE1; Supplementary Figure S4B). Indeed, there was little effect on the expression of a panel of genes related to proliferation and cell cycle control although the control AP-1 target gene MMP1 was downregulated (Supplementary Figure S4C). To provide further insights into the function of AP-1 in OAC, we therefore performed RNA-seq analysis on OE19 (29) and KYAE1 cells after expression of dnFOS for 2 days. RNA-seq replicates showed good correlation (Supplementary Figure S4D and E). We next identified genes that were differentially expressed in the presence of dnFOS and identified 168 upregulated and 340 downregulated genes in OE19 cells and 395 upregulated and 448 downregulated genes in KYAE1 cells (Figure 4C; Supplementary Table S6). Importantly there was a significant large overlap in down regulated targets with 171 genes (>50%) showing downregulation in both cell lines (Figure 4D) compared to only 49 genes (<30%) showing consistent upregulation (Supplementary Figure S4F). We verified that AP-1 subunits are involved in regulating the same genes as dnFOS by co-depleting FOS and FOSL1 (the two FOS subfamily members most affected by lapatinib treatment) and testing a panel of dnFOS-regulated genes. The majority of these genes were down regulated following knockdown of the AP-1 transcription factor subunits (Supplementary Figure S4G). Ingenuity Pathway Analysis of RNA-seq data revealed the expected inhibition of activity of the AP-1 family members FOS, FOSL1 and JUN following dnFOS expression in both OE19 and KYAE1 cell lines (Figure 4E). The dnFOS-mediated downregulated genes were associated with a number of GO terms related to EMT and cell migration such as ‘response to wounding’, ‘regulation of cell adhesion’ and ‘actin cytoskeleton organisation’ (Figure 4F). Example genes related to these GO terms include MMP7, ITGB4, IL8 and SMAD3 (Supplementary Figure S4H). In contrast, no changes were observed in CCND gene expression, consistent with a lack of a connection to cell cycle and proliferation categories (Supplementary Figure S4I). No GO terms were identified at the same levels of significance for the upregulated genes (Supplementary Figure S4J). Gene set enrichment analysis (GSEA) revealed EMT as a highly scoring category for genes downregulated in both cell lines (Figure 4G; Supplementary Figure S4K).
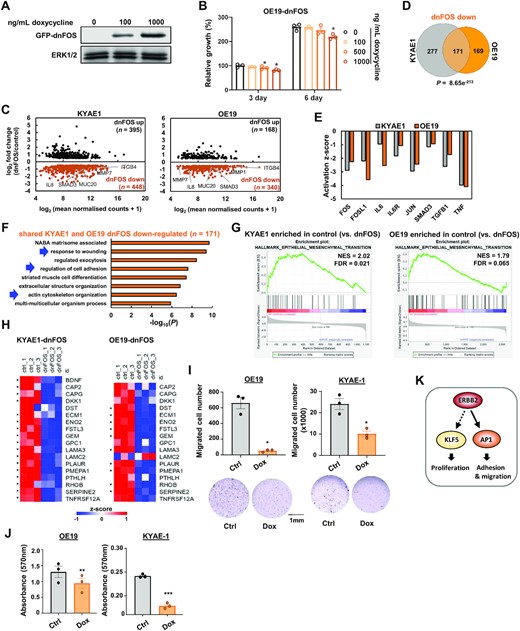
(A) Western blot of GFP-dnFOS protein levels in OE19-dnFOS cells. GFP-dnFOS expression was induced by treatment with the indicated doses of doxycycline for 48 h. (B) Crystal violet growth assay of OE19-dnFOS cells following dnFOS induction. * P < 0.05, paired T-test (n = 3). (C) MA plot showing DEGs (0.5X log2 fold change, FDR < 0.05, FPKM > 1) in KYAE1-dnFOS and OE19-dnFOS cells following GFP-dnFOS induction. Cells were untreated (control) or dnFOS expression was induced by 1000 ng/ml doxycycline treatment for 48 h (dnFOS). (D) Overlap of genes down-regulated (log2 fold change > 0.5, FDR < 0.05, FPKM > 1) by dnFOS induction in KYAE1-dnFOS and OE19-dnFOS cells (i.e. shared AP-1 target genes). (E) Ingenuity Pathway Analysis of proteins whose activity is predicted to be inhibited in KYAE1-dnFOS and OE19-dnFOS cells following dnFOS induction. (F) GO analysis (Metascape) of shared AP-1 target genes. (G) Enrichment plot of epithelial–mesenchymal transition hallmark from GSEA on differentially expressed up- and down- regulated genes in OE19 cells following dnFOS induction. NES – normalized enrichment score. (H) Z-scored mRNA expression of partial EMT (pEMT) genes (34) down-regulated in either KYAE1-dnFOS or OE19-dnFOS cells following dnFOS induction. * Denotes statistically significant changes (0.5 log2-fold change, FDR < 0.05). Note that BDNF expression was only detected in KYAE1 cells. (I) Cell migration assay of OE19-dnFOS or KYAE1-dnFOS cells treated with DMSO (ctrl) or doxycycline (Dox). ** P < 0.01, paired T-test (n = 3). (J) Adhesion assay of OE19-dnFOS or KYAE1-dnFOS cells treated with DMSO (ctrl) or doxycycline (Dox). ** P < 0.01, *** P <0.001, paired T-test (n = 3). (K) Model for ERBB2 signalling through AP-1 (this study) and KLF5 (36) to promote distinct biological processes. Dotted arrow indicates that direct molecular connections to KLF5 have not been shown (36).
Recently, a partial EMT-like state (pEMT) was identified as intrinsic to epithelial-derived cancer cells rather than the stromal compartment (34). We therefore asked whether expression of any of the 79 genes in the OAC pEMT signature are affected by AP-1 inhibition. Seventeen of the pEMT genes were significantly down regulated in either KYAE1 or OE19-dnFOS cells, with the majority of these showing downregulation in both cell lines (Figure 4H) with only one gene going in the opposite direction in both cases. This finding is substantiated by GSEA using a custom dataset created from the 79 pEMT genes (Supplementary Figure S4L). From the 17 pEMT genes responsive to AP-1 inhibition, 8 of these show upregulation in ERBB2HIGH OAC patient samples, while none showed lower expression (Supplementary Figure S4M). This is consistent with notion that this AP-1-regulated pEMT signature is active in ERBB2-driven OAC patients. To investigate further links to ERBB2 signalling, we also tested overlaps between the AP-1 target genes and those regulated by ERBB2 inhibition by genetic depletion or pharmacological inhibition. In both cases, a significant overlap was seen between downregulated genes when a stringent 2-fold cut off was applied (Supplementary Figure S4N) or when we considered the directionality of response (Supplementary Figure S4O). These results are therefore consistent with a link between ERBB2 signalling and AP-1 activity.
Finally, we studied the functional consequences of AP-1 inhibition based on the predictions from enriched GO terms and gene sets. First, we tested cell migration following dnFOS treatment of OE19 and KYAE1 cells and found a significant decrease in both cell lines (Figure 4I). Next, we tested cell adhesion and found it to be severely disrupted in both lines following AP1 inhibition (Figure 4J). We also assessed the status of the actin microtubule network in OE19 cells and found it to be altered after dnFOS expression with large foci of actin found in wild-type cells being disrupted following treatment (Supplementary Figure S4P).
Collectively these data therefore support a role for AP-1 in mediating the outputs from oncogenic ERBB2 signalling through affecting gene expression and the cellular functions associated with EMT-like processes.
DISCUSSION
Signalling through the RAS–ERK pathway is a hallmark of OAC and activating genetic disruptions to this pathway are observed in a large proportion of patients, indicating that is likely a pivotal event in the tumourigenic process (4,5). The most frequent aberrations observed are amplifications of the gene encoding the upstream RTK, ERBB2 and here we focussed on patients and cell lines harbouring this amplification event to gain mechanistic insights into the pathways through which ERBB2 controls OAC cell behaviour. Through combined analysis of phosphoproteomic, open chromatin and transcriptomic datasets we converged on a model whereby ERBB2 functions through activating the transcription factor AP-1 and its downstream target gene network to promote mesenchymal-like behaviour through changes to cell adhesion and migration (Figure 4K). Although we focussed on ERBB2, it is likely that AP-1 activity is elevated in OAC cells in which other components of the RTK signalling pathways are hyperactivated suggesting a broader impact on our understanding of OAC.
Functionally, we link AP-1 activity in OAC cells to control of EMT-related processes such as actin cytoskeletal structures, cell migration and cell adhesion. This is consistent with the functions of AP-1 in other cancer subtypes such as the recently uncovered link between AP-1 and mesenchymal transformation in glioblastomas (54) and more broadly links between AP-1 and mesenchymal-like behaviour across multiple cancer types (55). More generally, ERBB2 is also involved in these processes as exemplified from our phosphoproteomics and open chromatin profiling experiments following genetic or chemical inhibition of ERBB2 in OAC cells. Thus, the molecular links we make are supported by the functional similarities we uncover. Our data are consistent with earlier studies that linked AP-1 to RTK signalling in other cancer types where similar biological processes are affected (56). However, we were unable to uncover any links to CCND expression or more generally cell cycle and proliferation control, suggesting that ERBB2 controls this aspect of cell behaviour through alternative regulatory proteins. The transcription factor KLF5 is one such candidate, which we recently linked to cell cycle control in OAC (Figure 4K; (37)).
Our phosphoproteomic studies show that a multitude of phosphorylation changes are initiated shortly after ERBB2 inhibition and that this rewiring is maintained or further modified after 24 h. While we have focussed on the RAS–ERK pathway and the functional downstream consequences from this, many other kinase activities are altered. Central among these are the cyclin-dependent kinases (CDKs) whose activities are downregulated and likely contributes to the proliferation defects caused by ERBB2 inhibition. However, AKT1 and PRKCD (PKCδ) are among the immediately affected kinases and PAK1 features prominently after 24 hrs (Figure 1B and C). The latter kinase is of interest as it lies downstream of Cdc42/Rac signalling and transmits signals to the actin cytoskeleton reorganisation and subsequent cell motility. It is also defined as a ‘helper gene’ and is amplified in ∼4% of OAC cases (57). Thus, ERBB2 may impact both directly through modifying signalling cascade activity and indirectly through altering the cellular transcriptome and proteome, leading to rewiring of the signalling networks in the cell and subsequent phenotypic effects. Further work is required to understand the full impact of ERBB2 regulated pathways on the cellular behaviour of OAC cells.
While we have shown that AP-1 is the dominant transcriptional regulator downstream of ERBB2 signalling, it is likely that additional proteins are involved controlling ERBB2-mediated gene expression programmes. In addition to AP-1, our data implicate several other potential transcriptional regulators in ERBB2 functionality in OAC. For example, the changes in phosphorylation status of FOXK1/2, HMGA1 and ZNF609 suggest likely downstream gene regulatory consequences. Furthermore, open chromatin profiling reveals loss of accessibility in sites enriched for the binding of ETS, KLF and RUNX transcription factors following ERBB2 inhibition. Members of the ETS family (17,58,59) and KLF5 (36,59) have previously been implicated as important regulators of OAC gene expression programmes. RUNX2 has been identified as a helper gene and is amplified at a low level in OAC (∼2%; 57). Additional transcriptional regulators may have been missed by our analyses either due to the sensitivity of the phosphoproteomic analysis or for proteins whose binding site accessibility does not greatly change (eg by transcription factor exchange) and/or have relatively few target loci. Again further work is required to gain a comprehensive view of the transcriptional regulatory proteins controlled by oncogenic ERBB2 signalling.
In summary, we demonstrate that oncogenic ERBB2 signalling controls cell behaviour through modifying the activity of the AP-1 transcription factor. AP-1 itself drives processes associated with cell migration and invasion which might ultimately contribute to the metastatic spread of the tumours. Importantly, our results have broader significance across a wider range of OAC patients, given the frequent activation of other RTKs and downstream pathway components (4,5), which likely also impact on AP-1 activity. ERBB2 inhibition has been shown to have limited clinical efficacy with only a few months gained in overall survival time (6,60). Instead, targeting components of the downstream AP-1 regulatory network might represent an alternative more effective therapeutic route through which to combat ERBB2-driven OAC.
DATA AVAILABILITY
OE19 and KYAE1 dnFOSRNA-seq have been deposited at ArrayExpress (E-MTAB-10334).
SUPPLEMENTARY DATA
Supplementary Data are available at NAR Cancer Online.
ACKNOWLEDGEMENTS
We thank Guanhua Yan for excellent technical assistance, staff in the Bioinformatics, Genomic Technologies and Bioimaging core facilities. We also thank Angeliki Malliri for critical appraisal of the manuscript. We thank Joseph Parsons and Dr David Knight (Bio-MS Facility) for help with the phosphoproteomics experiments.
Author contributions: S.O., I.A. and S.H.Y. performed the experiments and data analysis in this study; P.F. prepared samples for proteomics and phosphoproteomics analysis. C.F. led the proteomic studies, performed data analysis and supervised P.F. and S.O.; A.D.S. led the other experimental parts of the program. All authors contributed to manuscript preparation and/or critically appraised manuscript drafts.
FUNDING
Wellcome Trust [103857/Z/14/Z, 102171/Z/13/Z, 102171/B/13/Z to A.D.S.]; research in CF lab is supported by the Wellcome Trust [107636/Z/15/Z, 107636/Z/15/A].
Conflict of interest statement. None declared.
Comments