-
PDF
- Split View
-
Views
-
Cite
Cite
Jialian Liu, Xiaofeng Wang, Alexei V Filippenko, Thomas G Brink, Yi Yang, Weikang Zheng, Hanna Sai, Gaobo Xi, Shengyu Yan, Nancy Elias-Rosa, Wenxiong Li, Xiangyun Zeng, Abdusamatjan Iskandar, Implications for the explosion mechanism of Type Ia supernovae from their late-time spectra, Monthly Notices of the Royal Astronomical Society, Volume 526, Issue 1, November 2023, Pages 1268–1286, https://doi.org/10.1093/mnras/stad2851
- Share Icon Share
ABSTRACT
Late-time spectra of Type Ia supernovae (SNe Ia) are important in clarifying the physics of their explosions, as they provide key clues to the inner structure of the exploding white dwarfs. We examined late-time optical spectra of 36 SNe Ia, including five from our own project (SNe 2019np, 2019ein, 2021hpr, 2021wuf, and 2022hrs), with phase coverage of ∼200 to ∼400 d after maximum light. At this late phase, the outer ejecta have become transparent and the features of inner iron-group elements emerge in the spectra. Based on multicomponent Gaussian fits and reasonable choices for the pseudo-continuum around Ni and Fe emission features, we get reliable estimates of the Ni to Fe ratio, which is sensitive to the explosion models of SNe Ia. Our results show that the majority (about 67 per cent) of our SNe Ia are more consistent with the sub-Chandrasekhar-mass (i.e. double-detonation) model, although they could be affected by evolutionary or ionization effects. Moreover, we find that the Si ii λ6355 velocity measured around the time of maximum light tends to increase with the Ni to Fe ratio for the subsample with either redshifted or blueshifted nebular velocities, suggesting that progenitor metallicity might play an important role in accounting for the observed velocity diversity of SNe Ia.
1 INTRODUCTION
It is widely accepted that thermonuclear explosions of carbon–oxygen (CO) white dwarfs (WDs) with masses close to the Chandrasekhar limit (Nomoto, Iwamoto & Kishimoto 1997; Hillebrandt & Niemeyer 2000; Maoz, Mannucci & Nelemans 2014) produce Type Ia supernovae (SNe Ia; see, e.g. Filippenko 1997 for a review of supernova classification). However, the mechanism that triggers the explosion and drives the propagation of the burning front, together with the nature of the donor, still remain unclear. Different models (that probably yield multiple valid channels of the explosion) invoke for the mass donor a non-WD companion such as a red giant or a helium star (the ‘single-degenerate’ channel, Whelan & Iben 1973), or another WD (the ‘double-degenerate’ channel, Iben & Tutukov 1984; Webbink 1984). Also, increasing attention is being paid to sub-Chandrasekhar-mass (sub-MCh) models (Bildsten et al. 2007; Shen & Bildsten 2009). In addition to the vast parameter space for the progenitor systems, since the spectral features of SNe Ia exhibit both intermediate-mass elements (IMEs, i.e. from Si to Ca) and iron-group elements (IGEs), a transition of the nuclear burning front from subsonic to supersonic phases is expected to take place. Various models are mainly differentiated by the mechanism that triggers the detonation, as follows.
In WDs near MCh, owing to the compressional heating, an initial burning starts as a subsonic deflagration near its mass centre. As the burning front propagates outward, some parts of the front would be accelerated due to the Rayleigh–Taylor (RT) instabilities. Subsequent transitions from the subsonic deflagration to the supersonic detonation would take place at the plume structures that develop (Gamezo, Khokhlov & Oran 2005; Seitenzahl et al. 2013). Such a later-ignited ‘delayed-detonation’ (DDT) front will synthesize the remaining WD into IGE-dominated products.
In WDs below MCh (i.e. ≲ 1.2 M⊙), the detonation of the CO WD can be triggered by an initial instability-induced detonation of an accreted helium shell on top of the WD (the ‘double-detonation’, Sim et al. 2010; Shen et al. 2018; Townsley et al. 2019).
Based on the result of hydrodynamic simulations, dynamical procedures such as the merger or the head-on collision of a double WD binary would also be able to meet the criteria that detonate the merged WD (Raskin et al. 2009; Kushnir et al. 2013; Pakmor et al. 2013; Pakmor 2017).
Because WDs are mostly electron-degenerate matter consisting of CO, all models that blow up the WD are anchored to the same network of nuclear reactions, which dominate the electromagnetic signatures of SNe Ia around their peak luminosity. Such a commonality thus lead to universal chemical compositions and energetics regardless of the model details. This also roughly explains why the photometric and spectroscopic evolution of SNe Ia around the time of maximum light can be well reproduced by a broad range of models.
When WDs explode as SNe Ia, the time-scale of their photometric evolution is well correlated with its peak luminosity. Both quantities are determined by the content of the heavy elements synthesized during the explosion. The peak luminosities of SNe Ia, including the slowly declining, hot, luminous SN 1991T-like or SN 1999aa-like objects, the rapidly declining, cool, subluminous SN 1991bg-like objects, and the ‘Branch-normal’ objects (Branch, Fisher & Nugent 1993), show a prominent correlation with the post-peak decline rate Δm15(B), with brighter objects having smaller Δm15(B) (Phillips 1993). This correlation has been dubbed the ‘Phillips relation.’ It serves as the basic recipe for the cosmological use of SNe Ia, and it is believed to be governed mainly by the amount of 56Ni produced in the explosion (Hoeflich & Khokhlov 1996). Moreover, there is increasing evidence showing that SN Ia peak luminosity is not parametrized only by the decline rate. The inclusion of a colour parameter helps tighten the dispersion of normalized peak luminosity (Tripp 1998; Wang et al. 2005) and improves distance estimates from SNe Ia (e.g. Guy et al. 2007; Betoule et al. 2014).
However, despite the first-order simplicity and commonality of SNe Ia, diversity among a range of subtypes has also been explored by various studies. For example, Benetti et al. (2005) found that SNe Ia exhibit large scatter in the velocity evolution of the ejecta, and the velocity gradient measured from Si ii λ6355 absorption is not correlated with Δm15(B) for Branch-normal SNe Ia. Wang et al. (2009b) divided Branch-normal SNe Ia into two groups based on Si ii λ6355 velocities measured around their time of B-band maximum light, with the high-velocity (HV) group having Si velocities |$\gtrsim 12\, 000$||$\rm km\ s^{-1}$| and the normal-velocity (NV) group having Si velocities |$\lesssim 12\, 000$||$\rm km\ s^{-1}$|. Such velocity diversity is independent of Δm15(B), and the origin of the spectral differences was interpreted as a geometric viewing-angle effect (Maeda et al. 2010a; Silverman, Ganeshalingam & Filippenko 2013). However, Wang et al. (2013) studied the birthplace environments of SNe Ia and found that HV SNe Ia tend to occur in inner, brighter regions of more-massive galaxies compared with the NV counterparts, suggesting that these two subclasses may have different progenitor properties. For example, HV SNe Ia are likely associated with metal-rich progenitors (Pan et al. 2015a; Pan 2020), and they have more circumstellar matter (CSM) than NV SNe Ia (Wang et al. 2019). Thus, the idea that all SNe Ia originate from one family or one explosion mechanism is challenged even if we do not consider peculiar subclasses of SNe Ia such as those defined by subluminous SN 1991bg-like (Filippenko et al. 1992), overluminous SN 1991T-like (Phillips et al. 1992), SN 2002es-like (Ganeshalingam et al. 2012), and SN 2009dc-like (Silverman et al. 2011) SNe Ia.
To further explore the explosion mechanism and progenitor physics of SNe Ia, we need to inspect the inner regions of their ejecta. At early times, the outer ejecta are opaque, and the deeper regions are hidden. At a phase of over 200 d after peak brightness, the ejecta have expanded substantially and become transparent to the radiation from the inner core, which is dominated by emission from the Fe-group elements. At such nebular phases, the shape of the spectral profile over the wavelength range of 6800–7800 Å, dominated by [Fe ii] and [Ni ii] features (Maguire et al. 2018; Flörs et al. 2020), delivers critical constraints on the structure and abundance ratio of the Fe-group elements. For instance, the non-zero velocity shifts of [Fe ii] and [Ni ii] features indicate an asymmetric explosion (Maeda et al. 2010b). In addition, the iron is mainly contributed by the end product of the radioactive decay of 56Ni, and the stable nickel was synthesized by the explosion. Thus, the Ni/Fe ratio in the nebular phase reflects the ratio of stable to radioactive isotopes of Fe-group elements produced in the explosion, which is sensitive to the central density of the exploding WD. Maguire et al. (2018) used a multicomponent Gaussian fit to measure the Ni/Fe ratio in the 7300 Å region in optical spectra to distinguish MCh and sub-MCh models since the central densities of WDs are quite different for these two explosion models. Flörs et al. (2020) and Graham et al. (2022) also measured the Ni/Fe ratio using different methods, but their results were generally lower than those given by Maguire et al. (2018). Flörs et al. (2020) mentioned that the differences are mainly due to the placement of the pseudo-continuum across the 7300 Å region. In this work, we generally follow the fitting method of Maguire et al. (2018), but adopt the pseudo-continuum in a different way.
Using nebular-phase spectra of SNe Ia published in the literature as well as data collected through our own program, we attempt to provide more-accurate measurements of the Ni/Fe ratio to put tighter constraints on the explosion mechanism of SNe Ia. Since the Ni/Fe ratio can be affected by the progenitor metallicity (Timmes, Brown & Truran 2003; Shen et al. 2018; Shingles et al. 2020), we also examine correlations of this ratio with SN Ia observables measured at early times, such as the Si ii velocity and Δm15(B).
The paper is structured as follows. We outline the sample used in our analysis in Section 2, and Section 3 shows the fitting methods. Our results are presented in Section 4 and discussed in Section 5. Section 6 summarizes our conclusions.
2 DATA SOURCES
In order to examine the distribution of the Ni/Fe ratio, we collect a sample of 58 late-time spectra taken at t ≈ +200–400 d after maximum light for 36 SNe Ia. Those showing peculiar properties such as SN 1991T-like and SN 1991bg-like SNe Ia are also included except when their spectra exhibit strong calcium features or have a flat profile in the 7300 Å region. The publicly available data were retrieved using the Open Supernova Catalog (OSC, Guillochon et al. 2017), the Weizmann Interactive Supernova data REPository (WISeREP, Yaron & Gal-Yam 2012), and the Supernovae Database (SNDB, Silverman et al. 2012a; Shivvers et al. 2019). The t ≈ +384 d spectrum of SN 2017fgc comes from Zeng et al. (2021). Information on the spectra collected by our own project is presented in Section 2.1. An overview of the observations for all the SNe Ia in this work is listed in Table A1. References for all the late-time spectra can be found in Table A2.
To study the connections between early- and late-time properties, we used the spectrum around the time of maximum light (i.e. within about 3 d from the B-band peak, except for SN 2012hr at +5 d and ASASSN-14jg at + 6 d) to measure the Si ii λ6355 velocity from the absorption minimum for each object of our sample. The Si velocities and the phase of the spectra used in the measurements are presented in Table A1. We collected the post-peak decline rate Δm15(B) and the date of B-band maximum from the literature when available. For SNe 2003kf, 2012hr, and 2013cs, these two parameters are estimated by applying the SALT2 (Guy et al. 2007) fit to their light curves. All spectra used in the analysis have been corrected for host-galaxy redshift from the NASA/IPAC Extragalactic Database (NED) and extinction due to the Milky Way (Schlafly & Finkbeiner 2011) and host galaxy whenever possible (for details, see Table A1). As we only focus on the 7300 Å region in the spectra, the results should suffer little from uncertainties in extinction corrections.
2.1 Late-time spectra from our project
Our program aims to collect some late-time spectra for SNe Ia that were well observed at early phases. Thus far, we have obtained late-time spectra of SN 2019np (Sai et al. 2022), SN 2019ein (Xi et al. 2022), SN 2021hpr (Iskandar et al., in preparation), SN 2021wuf (Zeng et al., in preparation), and SN 2022hrs (Liu et al., in preparation). The nebular spectra of these last four SNe Ia were taken with the Low Resolution Imaging Spectrometer (LRIS; Oke et al. 1995) mounted on the 10 m Keck-I telescope and the DEep Imaging Multi-Object Spectrograph (DEIMOS; Faber et al. 2003) on the 10 m Keck-II telescope on Maunakea, and the Kast double spectrograph (Miller & Stone 1993) mounted on the Shane 3 m telescope at Lick Observatory. The late-time spectra of SN 2019np were obtained with the Optical System for Imaging and low-Intermediate-Resolution Integrated Spectroscopy (OSIRIS) mounted on the 10.4 m Gran Telescopio CANARIAS (GTC) at the Roque de Los Muchachos Observatory (Spain). To minimize slit losses caused by atmospheric dispersion (Filippenko 1982), the slit was oriented at or near the parallactic angle. The Keck I/LRIS spectra were reduced using the LPipe pipeline (Perley 2019). The GTC/OSIRIS, Keck II/DEIMOS, and Shane/Kast observations were reduced using standard IRAF1 routines for CCD processing (e.g. Silverman et al. 2012a) and optimal spectrum extraction (Horne 1986). The spectra were flux calibrated using observations of appropriate spectrophotometric standard stars observed on the same night, at similar airmasses, and with an identical instrument configuration. We corrected for the atmospheric extinction using the extinction curves of local observatories. A journal of observations is given in Table 1 and the corresponding spectra are shown in Fig. 1.
![Late-time spectra of SNe 2019ein, 2019np, 2021hpr, 2021wuf, and 2022hrs. Flux densities (fλ) are all normalized to the [Fe iii] 4700 Å feature and smoothed with a Savitsky–Golay filter of width ∼50 Å and order 1. The rest-frame wavelengths of [Fe iii] 4659 Å, [Fe ii] 7155 Å, and [Ni ii] 7378 Å are marked by dashed lines.](https://oup.silverchair-cdn.com/oup/backfile/Content_public/Journal/mnras/526/1/10.1093_mnras_stad2851/1/m_stad2851fig1.jpeg?Expires=1750230203&Signature=Xy1CMoBmVlsTQA8yRdJyYLReAq1S7~cW4UgTes~8dRiSuTVYXya1hGwNi7y6P4hcSSYwpIhRJSmG3x81JTOvexNPI9~GIjB3KpIhpxqC0oSrS6Zb~P8fZiPytqWOh5T4UWP2HZ44PYOwfLsSX0ZSxI8vYTuhOydYllyuQpP7JM-x4FOQ7Xj3aJtQgBy9PheCZJAGzt82NvhsLlsv11PEkVpGI~nQn0vA38QPFsDzU4Ys2gOW5httfQ5YxKUpqcQlxRaTH1umuAs~x9nmlYxji-e9q4itMlrl5mo1KLbSWLHCooVrtvqtCDxpg4V8E-Y2VD7tSJ5-nec0~Wxp0ZbRiQ__&Key-Pair-Id=APKAIE5G5CRDK6RD3PGA)
Late-time spectra of SNe 2019ein, 2019np, 2021hpr, 2021wuf, and 2022hrs. Flux densities (fλ) are all normalized to the [Fe iii] 4700 Å feature and smoothed with a Savitsky–Golay filter of width ∼50 Å and order 1. The rest-frame wavelengths of [Fe iii] 4659 Å, [Fe ii] 7155 Å, and [Ni ii] 7378 Å are marked by dashed lines.
Name . | Observation . | Observation . | Phase . | Range . | Instrument . | Telescope . | Exposure . |
---|---|---|---|---|---|---|---|
. | MJD . | date (UTC) . | (d) . | (Å) . | . | . | (s) . |
SN 2019ein | 58 932 | 2020 Mar 24 | + 313 | 3147–10 278 | LRIS | Keck I | 1077.5 |
SN 2019np | 58 813 | 2019 Nov 26 | + 303 | 3812–7830 | OSIRIS | GTC | 1380 |
SN 2019np | 58 878 | 2020 Jan 30 | + 368 | 3832–7831 | OSIRIS | GTC | 1380 |
SN 2021hpr | 59 585 | 2022 Jan 6 | + 263 | 3622–10 380 | Kast | Shane | 3600 |
SN 2021hpr | 59 610 | 2022 Jan 31 | + 288 | 3166–10 275 | LRIS | Keck I | 1200 |
SN 2021wuf | 59 670 | 2022 Apr 1 | + 208 | 3142–10 272 | LRIS | Keck I | 1200 |
SN 2021wuf | 59 761 | 2022 July 1 | + 299 | 3138–10 259 | LRIS | Keck I | 2400 |
SN 2022hrs | 59 995 | 2023 Feb 20 | + 297 | 3634–10 752 | Kast | Shane | 3660 |
SN 2022hrs | 60 025 | 2023 Mar 22 | + 327 | 4420–9626 | DEIMOS | Keck II | 1500 |
Name . | Observation . | Observation . | Phase . | Range . | Instrument . | Telescope . | Exposure . |
---|---|---|---|---|---|---|---|
. | MJD . | date (UTC) . | (d) . | (Å) . | . | . | (s) . |
SN 2019ein | 58 932 | 2020 Mar 24 | + 313 | 3147–10 278 | LRIS | Keck I | 1077.5 |
SN 2019np | 58 813 | 2019 Nov 26 | + 303 | 3812–7830 | OSIRIS | GTC | 1380 |
SN 2019np | 58 878 | 2020 Jan 30 | + 368 | 3832–7831 | OSIRIS | GTC | 1380 |
SN 2021hpr | 59 585 | 2022 Jan 6 | + 263 | 3622–10 380 | Kast | Shane | 3600 |
SN 2021hpr | 59 610 | 2022 Jan 31 | + 288 | 3166–10 275 | LRIS | Keck I | 1200 |
SN 2021wuf | 59 670 | 2022 Apr 1 | + 208 | 3142–10 272 | LRIS | Keck I | 1200 |
SN 2021wuf | 59 761 | 2022 July 1 | + 299 | 3138–10 259 | LRIS | Keck I | 2400 |
SN 2022hrs | 59 995 | 2023 Feb 20 | + 297 | 3634–10 752 | Kast | Shane | 3660 |
SN 2022hrs | 60 025 | 2023 Mar 22 | + 327 | 4420–9626 | DEIMOS | Keck II | 1500 |
Name . | Observation . | Observation . | Phase . | Range . | Instrument . | Telescope . | Exposure . |
---|---|---|---|---|---|---|---|
. | MJD . | date (UTC) . | (d) . | (Å) . | . | . | (s) . |
SN 2019ein | 58 932 | 2020 Mar 24 | + 313 | 3147–10 278 | LRIS | Keck I | 1077.5 |
SN 2019np | 58 813 | 2019 Nov 26 | + 303 | 3812–7830 | OSIRIS | GTC | 1380 |
SN 2019np | 58 878 | 2020 Jan 30 | + 368 | 3832–7831 | OSIRIS | GTC | 1380 |
SN 2021hpr | 59 585 | 2022 Jan 6 | + 263 | 3622–10 380 | Kast | Shane | 3600 |
SN 2021hpr | 59 610 | 2022 Jan 31 | + 288 | 3166–10 275 | LRIS | Keck I | 1200 |
SN 2021wuf | 59 670 | 2022 Apr 1 | + 208 | 3142–10 272 | LRIS | Keck I | 1200 |
SN 2021wuf | 59 761 | 2022 July 1 | + 299 | 3138–10 259 | LRIS | Keck I | 2400 |
SN 2022hrs | 59 995 | 2023 Feb 20 | + 297 | 3634–10 752 | Kast | Shane | 3660 |
SN 2022hrs | 60 025 | 2023 Mar 22 | + 327 | 4420–9626 | DEIMOS | Keck II | 1500 |
Name . | Observation . | Observation . | Phase . | Range . | Instrument . | Telescope . | Exposure . |
---|---|---|---|---|---|---|---|
. | MJD . | date (UTC) . | (d) . | (Å) . | . | . | (s) . |
SN 2019ein | 58 932 | 2020 Mar 24 | + 313 | 3147–10 278 | LRIS | Keck I | 1077.5 |
SN 2019np | 58 813 | 2019 Nov 26 | + 303 | 3812–7830 | OSIRIS | GTC | 1380 |
SN 2019np | 58 878 | 2020 Jan 30 | + 368 | 3832–7831 | OSIRIS | GTC | 1380 |
SN 2021hpr | 59 585 | 2022 Jan 6 | + 263 | 3622–10 380 | Kast | Shane | 3600 |
SN 2021hpr | 59 610 | 2022 Jan 31 | + 288 | 3166–10 275 | LRIS | Keck I | 1200 |
SN 2021wuf | 59 670 | 2022 Apr 1 | + 208 | 3142–10 272 | LRIS | Keck I | 1200 |
SN 2021wuf | 59 761 | 2022 July 1 | + 299 | 3138–10 259 | LRIS | Keck I | 2400 |
SN 2022hrs | 59 995 | 2023 Feb 20 | + 297 | 3634–10 752 | Kast | Shane | 3660 |
SN 2022hrs | 60 025 | 2023 Mar 22 | + 327 | 4420–9626 | DEIMOS | Keck II | 1500 |
3 FITTING METHOD
3.1 Multicomponent Gaussian fit
To derive the Ni/Fe ratio in an SN Ia explosion, we focus on the 7300 Å region in the nebular-phase spectra, where the emission features are dominated by [Fe ii] (7155, 7172, 7388, and 7453 Å) and [Ni ii] (7378 and 7412 Å) (Maguire et al. 2018). The [Fe ii] features mainly come from 56Fe which results from the decay of 56Co, while the [Ni ii] features are mostly contributed by stable 58Ni, since radioactive 56Ni has a half-life of only 6.1 d and its contribution is negligible at late times.
To measure accurately the velocity shift, width, and strength of the above features, a multicomponent Gaussian function is used to fit the line profiles following Maguire et al. (2018),
where Ai is the strength of the emission feature, |$\lambda _i^{\rm centre}$| is the central wavelength, and σi represents the width. The subscript i denotes different components. With these parameters, we can calculate the velocity shift (vi), full width at half-maximum intensity (|$\rm FWHM_i$|), and flux (Fi) for different emission components:
where |$\lambda _i^{\rm rest}$| is the rest-frame wavelength of the corresponding emission component and c is the speed of light, and we use the relativistic Doppler formula to convert wavelengths to velocities. The Ni/Fe ratio is inferred from the flux ratio of [Ni ii] 7378 Å and [Fe ii] 7155 Å, F7378/F7155; more details are presented in Section 4.2.
Before fitting the Ni and Fe emission features, each spectrum is smoothed with a Savitsky–Golay filter of width ∼20–50 Å and order 1 using the scipy package’s signal.savgol_filter function. The pseudo-continuum is defined as a straight line connecting the interactively chosen points to the red and blue of the features in the 7300 Å region. We notice that the red side of the features near 7600 Å were often affected by telluric absorption lines, so we choose a region longward of this (i.e. at ∼7700 Å) as the red continuum point when such absorption appears in the spectrum, and we mask the absorption before fitting. After subtracting the pseudo-continuum, we apply a multicomponent Gaussian function to fit the observed features. Following Maguire et al. (2018), line widths and velocity shifts of the same species are set to the same values, and the relative strengths of the same species are fixed as well. Following Jerkstrand et al. (2015), the strengths of the [Fe ii] 7172, 7388, and 7453 Å features relative to [Fe ii] 7155 Å are set to 0.24, 0.19, and 0.31, respectively, while the [Ni ii] 7412 Å feature has a relative strength of 0.31 compared to [Ni ii] 7378 Å. Thus, there are only six free parameters for the multicomponent Gaussian function: width, velocity shift, and strength of each of [Fe ii] 7155 Å and [Ni ii] 7378 Å. Similar to Graham et al. (2022), we also place the boundary of the nickel width to be |$\lesssim 13\, 000$| km s−1.
It should be noted that possible [Ca ii] emission at 7291.5 and 7323.9 Å could contaminate the late-time spectra. We try to add two additional Gaussian components to represent the weak [Ca ii] emission lines in the fit, where the velocity shifts of the [Fe ii] and [Ni ii] are set to the same values; otherwise the [Ca ii] could dominate the blue or red peak. We find that the influence of the [Ca ii] emission on our fitting is very limited for most objects except the SN 1991bg-like SNe Ia such as SN 1986G and SN 2003gs, which is consistent with previous work (e.g. Graham et al. 2017; Maguire et al. 2018; Flörs et al. 2020; Tucker et al. 2022). In fact, the spectra of SN 1986G and SN 2003gs cannot be fit well by only [Fe ii] and [Ni ii], so we fit them by adding the [Ca ii] emission lines.
To estimate the uncertainties, the endpoints of the continuum vary within 10–50 Å (smaller variation ranges for lower-quality spectra to avoid improperly determining of the continuum; if some regions are masked, their edges are varied within 10 Å) and the emission features at 7100–7400 Å are refit 1000 times through the Monte Carlo method. A fit is rejected when the FWHM of nickel emission features is >12 500 km s−1. The standard deviation in the measurement is taken as one part of the uncertainty of the Gaussian parameters. Additional uncertainty comes from smoothing the spectrum, which is taken as the difference between the results given by the smoothed spectrum and the observed one. Following Maguire et al. (2018), an uncertainty of 200 |$\rm km\ s^{-1}$| is added to the velocity-shift measurement to account for the peculiar velocities of host galaxies.
Graham et al. (2022) also adopted the analysis method of Maguire et al. (2018) to fit the 7300 Å region, finding that the nickel line profile can be unusually broad. Thus, they proposed a two-stage minimum-Ni fit to further suppress the nickel contribution, which led to a lower Ni/Fe ratio. Flörs et al. (2020) used a non-local-thermodynamic-equilibrium (NLTE) level population model of the first and second ionization stages of iron, nickel, and cobalt to fit the full late-time spectra for their SN Ia sample. The Ni/Fe ratios derived by them are lower than those obtained by Maguire et al. (2018) for the same objects and the deviations were attributed to the placement of the pseudo-continuum. Although in this work we mainly follow the fitting method of Maguire et al. (2018), we try to give a more reasonable placement of the pseudo-continuum to make the Ni/Fe ratio more accurate; see Section 3.2.
3.2 Selection of pseudo-continuum
We notice that there exists a small bump at 6800–7000 Å in most spectra of our sample, which tends to disappear at later phases and might be contributed by Co lines such as [Co iii] λ6855 (Flörs et al. 2020). In the analysis by Maguire et al. (2018), the blue end of the continuum for the emission features near 7100–7400 Å was chosen as the point around 6900 Å (called point A′), which would induce a steeper pseudo-continuum. In our analysis, we prefer to choose the minimum in the blue wing of the bump as the blue end of the continuum (called point A) to place a flatter pseudo-continuum. Fig. 2 shows a comparison of these two placements for spectra of SN 2011fe and SN 2012fr, two well-observed nearby SNe Ia (e.g. Childress et al. 2015; Zhang et al. 2016). The pseudo-continuum defined with point A′ is steeper than that defined with point A, significantly affecting the fitting results. In the following analysis, we call these two cases of choosing the blue side of the continuum as cases A and A′, respectively.
![Evolution of the 7300 Å region shown for late-time spectra of SN 2011fe and SN 2012fr. All spectra have been smoothed with a Savitsky–Golay filter of width ∼50 Å and order 1. Upper panel: evolution of unscaled spectra. Middle panel: evolution of spectra scaled to the 7155 Å peak. The pseudo-continuum is defined as a straight line connecting the red and blue endpoints of the fitting region. The pseudo-continuum with point A′ or point A as the blue endpoint are shown with red and blue dashed lines, respectively. The bump near 6900 Å affecting the definition of the pseudo-continuum and the region near 7900 Å which might be contaminated by [Ni iii] are labelled by the shaded region. Bottom panel: evolution of spectra from which the pseudo-continuum defined in the middle panel has been subtracted. The spectra obtained by subtracting the pseudo-continuum defined by A or A′ are shown as solid and dashed, respectively.](https://oup.silverchair-cdn.com/oup/backfile/Content_public/Journal/mnras/526/1/10.1093_mnras_stad2851/1/m_stad2851fig2.jpeg?Expires=1750230203&Signature=2f-vVEzYAIaH9cz2wn~HORr07a7qtEFEeuEhRBmxtE2t8CWOC3Rzym2DWOCehb5Nc~4f4mEoW278XKweyxseiZkyVYv7wb5NfasP8h~L777pm0CWNo2MaGAQPmTXnro9nNp3tok7GNNkx60chsIZlZrjXPyL5GriPMmrR0exvGUldLcKCUhid7hKnkE9FLXaXBodzQD~g7GmC~0twCo6oWjkqIS5FBaJladTTGSivN80my~VlOR6w3IUHezqYa5uXvid393uodhnC~gAJui-Ra0H1TKZGRBaTbbwGOqNXwr52sV1LlYicMs9EV-N799VapGgp6Tz1mtrpgdowa99OQ__&Key-Pair-Id=APKAIE5G5CRDK6RD3PGA)
Evolution of the 7300 Å region shown for late-time spectra of SN 2011fe and SN 2012fr. All spectra have been smoothed with a Savitsky–Golay filter of width ∼50 Å and order 1. Upper panel: evolution of unscaled spectra. Middle panel: evolution of spectra scaled to the 7155 Å peak. The pseudo-continuum is defined as a straight line connecting the red and blue endpoints of the fitting region. The pseudo-continuum with point A′ or point A as the blue endpoint are shown with red and blue dashed lines, respectively. The bump near 6900 Å affecting the definition of the pseudo-continuum and the region near 7900 Å which might be contaminated by [Ni iii] are labelled by the shaded region. Bottom panel: evolution of spectra from which the pseudo-continuum defined in the middle panel has been subtracted. The spectra obtained by subtracting the pseudo-continuum defined by A or A′ are shown as solid and dashed, respectively.
We mask the bump feature at 6800–7000 Å before fitting. However, this bump may be blended with iron emission [Fe ii] 7155 Å. To roughly estimate the influence on the fitting, we try to use another Gaussian component to fit the bump feature near 6900 Å instead of masking it. We find that the F7378/F7155 ratio derived by these two methods can differ by about 15 per cent when using the t ≈ +222 d spectrum of SN 2012fr. Such a discrepancy becomes less significant with time, and it nearly disappears in the t ≈ +357 d spectrum of SN 2012fr. Assuming that the bump feature near 6900 Å is indeed mainly contributed by [Co iii] 6855 Å, it has a limited effect on the iron feature unless the [Co iii] emission has an unreasonably broad width.
An important reason for us to choose case A is that it gives a smaller systematic error for the estimation of feature velocities. Assuming that velocities of [Fe ii] 7155 Å and [Ni ii] 7378 Å are similar (Maeda et al. 2010b), the velocity difference of these two features should be close to zero or at least has a symmetric distribution with respect to zero for our sample. Fig. 3 compares the velocity fitting results between cases A and A′. We find that the velocity difference of case A′ with a mean value of ∼−900 km s−1 tends to be bluer – that is, the nickel velocity tends to be bluer than the iron velocity. This bias is more serious for the spectra taken at t < +300 d. Meanwhile, the velocity difference distribution of case A is consistent with our expectations, with a mean value of ∼−200 km s−1, close to zero.
![Comparisons of the velocity difference of [Ni ii] 7378 Å and [Fe ii] 7155 Å between cases A and A′. The velocity difference inferred from the spectra taken at t < +300 d are shown with green dots, while those taken at t > +300 d are shown with purple dots.](https://oup.silverchair-cdn.com/oup/backfile/Content_public/Journal/mnras/526/1/10.1093_mnras_stad2851/1/m_stad2851fig3.jpeg?Expires=1750230203&Signature=YIMOrjbnsimn2vNp6fvBy1e0Zdmo41tGO34aWLMpXweZHhEXFAkjlJZAjDIHOk9CtBIa4On3dIT7VckMj9HOoLhD2wTt4LIRwHaVkScAIFKM-2fT0VOI~Ezx~ZD6jusOB-JOeniI8YvTGDxdvZEJgY8RXZdZXQbrJyli1wqEwCNMLDD5P4Rbq8MuBreeF6-69E4qkgG0UZ5UoX60k03vsnA6wWtAt5zGLnFJEIi-36mzzMG3ggmt30SQNp2JMusY~z3dNk~pbuszMeVdAutyLVwNEQ24jKvC2zd6xq27W2I9PisElF0~7M-1vVBfOSaBGbVmpjSFxU871Lsm-pX7Gg__&Key-Pair-Id=APKAIE5G5CRDK6RD3PGA)
Comparisons of the velocity difference of [Ni ii] 7378 Å and [Fe ii] 7155 Å between cases A and A′. The velocity difference inferred from the spectra taken at t < +300 d are shown with green dots, while those taken at t > +300 d are shown with purple dots.
Another problem of case A′ is the overly broad nickel component in some fitting cases, which is also mentioned by Graham et al. (2022). We find that, in most cases, the unreasonably broad nickel component can be avoided by using point A to set the pseudo-continuum and adjusting the masking regions. When the adjustment does not work (only for the spectrum of SN 2017cbv at +317 d in our sample), we assume that the velocity shifts of [Fe ii] and [Ni ii] are the same, which can also avoid the overly broad nickel.
Fig. 4 shows the best fit to the 7300 Å region for the late-time spectra of SNe 2017fgc, 2019ein, 2019np, 2021hpr, 2021wuf, and 2022hrs.
![Best fits to the 7300 Å region dominated by [Fe ii] and [Ni ii] features. The reddening-corrected spectra are shown in grey, while the smoothed spectra are in black. The overall fits are shown with a red line, while the fitting regions are indicated by blue lines. The [Fe ii] and [Ni ii] features are represented by purple and green dashed lines, respectively.](https://oup.silverchair-cdn.com/oup/backfile/Content_public/Journal/mnras/526/1/10.1093_mnras_stad2851/1/m_stad2851fig4.jpeg?Expires=1750230203&Signature=a6ZfUoKwpSWnYDep-tBw2hHah0AALP0n09E9X2APiP8AgbtFysUwpW4Hnpe-DlkGN80W8JBQYA1u57FOJRgzfJ6mF3wD3yxhewqDssCO1cRasvTIIw9Z3Dz11pzBNr0CFpqw7jvq-vvePp6RwAuGmPFTR~QkYgoy9MJPag1PU3kf7-E5N0HSoPVyaoKsuu0WlRykOQMOkyo2DN9zJj8LHxwZTuLOW9DVnJDhsd8lCk7Gcb5vKlzfjJsdN5bdoaSvyOAWr~LDj1aI9CDlf6likmkjnLr46WvNVBT1S39s5pcxTGLGuas~fD9-KfAXRSJixVNbgDd9jqO5lScnrBHWJA__&Key-Pair-Id=APKAIE5G5CRDK6RD3PGA)
Best fits to the 7300 Å region dominated by [Fe ii] and [Ni ii] features. The reddening-corrected spectra are shown in grey, while the smoothed spectra are in black. The overall fits are shown with a red line, while the fitting regions are indicated by blue lines. The [Fe ii] and [Ni ii] features are represented by purple and green dashed lines, respectively.
4 RESULTS
The velocity shifts and line widths (i.e. FWHM) of the [Fe ii] 7155 Å and [Ni ii] 7378 Å features as well as their flux ratios are listed in Table A2. Significant differences are found to exist in the velocity shifts and FWHM between the [Fe ii] 7155 Å and [Ni ii] 7378 Å features for some objects, as discussed in Section 4.1. We use the velocity shifts of [Fe ii] 7155 Å and [Ni ii] 7378 Å to identify whether the nebular velocities are redshifted or blueshifted, which might have a connection with asymmetric explosion models (Maeda et al. 2010a). We then use the flux ratio of [Fe ii] 7155 Å and [Ni ii] 7378 Å to roughly estimate the Ni/Fe ratio and constrain the explosion models. The fits for all the spectra used in this work are shown in Fig. A1. The inferred nebular velocities and Ni/Fe ratios are also listed in Table A2. Some potential correlations can be found between nebular velocities, Ni/Fe ratios, Si ii velocities at maximum light, and post-maximum decline rates Δm15(B).
4.1 Velocities and widths of [Fe ii] and [Ni ii] features
Nebular velocities were often estimated from the mean velocity shift inferred from the [Fe ii] 7155 Å and [Ni ii] 7378 Å emission features (e.g. Maeda et al. 2010a; Silverman et al. 2013). However, we note that the velocities inferred from [Fe ii] and [Ni ii] lines tend to show noticeable differences (e.g. over 1000 km s−1) when their emission peaks are not of comparable strength. The velocity inferred from the weaker feature usually has a larger uncertainty relative to the stronger one. To quantify the difference between these two velocities, we apply a simple linear fit and find that |$v_{\rm Ni} = 1.24\, v_{\rm Fe}-170$| km s−1. For an absolute mean velocity of vFe = 1260 km s−1, the difference in vNi and vFe is within about 500 km s−1, which is not significant. As the [Fe ii] 7155 Å feature is usually stronger and has smaller measurement uncertainties in our sample, we adopt the [Fe ii] velocity to represent the nebular velocity except when the [Ni ii] 7378 Å feature is stronger. In these cases, we use the [Ni ii] velocity to represent the nebular velocity (e.g. for SN 2003hv).
Fig. 5 shows the nebular velocities inferred from the [Fe ii] 7155 Å or [Ni ii] 7378 Å features in spectra taken at different phases. As can be seen, the nebular velocity does not show significant evolution in a given object. When the nebular velocity is close to zero within the quoted uncertainty, we consider its nebular velocity as zero instead of identifying it as a redshifted or blueshifted subclass. Among the 36 SNe Ia collected in our study, we find that 14 can be classified as having redshifted nebular velocities and 18 as having blueshifted ones. The numbers of redshifted and blueshifted objects are comparable, and the nebular velocities have a roughly symmetric distribution in the approximate range −3000 to 3000 km s−1.
![Left panel: nebular velocities inferred from the [Fe ii] 7155 Å or [Ni ii] 7378 Å features as a function of time. Right panel: the corresponding histogram for average nebular velocity measured for our sample.](https://oup.silverchair-cdn.com/oup/backfile/Content_public/Journal/mnras/526/1/10.1093_mnras_stad2851/1/m_stad2851fig5.jpeg?Expires=1750230204&Signature=uK3yFqktOwovo9DsrWmYjF1VVmNIgD2iBj3IaOhOSoGoH-7Gl7aDAwNu6vfUfQiv3DQI0o0fV0Qd2MNN3flQztNrcUIqBNFA6zyq1uH5XLRluEgbyGEKD7zc39MIWIb0-XbgnrlkcCq948u4koWDDXikvZP~DCvkKMdrieaLbTPHIV3gb3CtUhXbVSD5WyweoRJCLoYZikacDDaFmomuGJvTpRUOkQ64MIEuYx66CQtzjOepXiyARdFZeyPR8tCdUx1GS0bQtdGukDNs-6PZ1POyZAiTe7kCXr9xo6daHNG1ETSpYCVz1jQPzMQnfrht1j0j-f5aCyLyqRPRoSmQJQ__&Key-Pair-Id=APKAIE5G5CRDK6RD3PGA)
Left panel: nebular velocities inferred from the [Fe ii] 7155 Å or [Ni ii] 7378 Å features as a function of time. Right panel: the corresponding histogram for average nebular velocity measured for our sample.
For the line widths, there is considerable scatter between the FWHM of [Fe ii] and [Ni ii] features. The [Fe ii] FWHM is usually larger than the [Ni ii] FWHM, with mean values of 8429 ± 961 and 6078 ± 1445 km s−1 (respectively) – approximately consistent with the DDT models of Seitenzahl et al. (2013) where 56Ni usually has a slightly wider distribution than 58Ni (see their fig. 8). But in some extreme cases, the [Fe ii] FWHM is much larger than the [Ni ii] FWHM (e.g. more than a factor of 2), not expected from the models. Note that the difference between [Fe ii] and [Ni ii] FWHM evolves with phase, with a tendency of being larger at earlier nebular phases (e.g. ASASSN-14jg), which can partially explain the large FWHM differences as revealed in Table A2. Nevertheless, inspecting the measurements for those spectra taken at similar phases (i.e. t ≈ 250 d), we still find large FWHM scatter between [Ni ii] and [Fe ii] lines, suggesting that this scatter might be genuine and its origin should be further explored.
4.2 Estimation of Ni/Fe ratio
The Ni/Fe ratio is estimated following the method of Maguire et al. (2018),
where |$d_{C_{\rm Fe~II}}/d_{C_{\rm Ni~II}}$| denotes the ratio of departure coefficients, k is the Boltzmann constant in units of eV per K, and T is the temperature. L7378/L7155 represents the luminosity ratio and is estimated as the measured flux ratio of [Fe ii] 7155 Å and [Ni ii] 7378 Å. Note that Maguire et al. (2018) used the ratio of pseudo-equivalent width of these two lines.
To obtain a rough estimate of the Ni/Fe ratio, Maguire et al. (2018) adopted the value of the ratio of departure coefficients, temperature, and ionization balance from a day 330 W7 model of Fransson & Jerkstrand (2015). The ratio |$d_{C_{\rm Fe~II}}/d_{C_{\rm Ni~II}}$| has a range of 1.2–2.4 over the phase of interest, with smaller coefficients at earlier times when the conditions are slightly closer to LTE. The temperature is assumed to range from 3000 to 8000 K, while the Ni/Fe ratio nNi/nFe is considered to be equal to nNi II/nFe II. We also use these estimates to infer the Ni/Fe ratio. Then the mass ratio of Ni and Fe is estimated using 56Fe and 58Ni. The relative uncertainty of the Ni/Fe ratio obtained in this way is about 40 per cent (Maguire et al. 2018), and it is combined with that of the measured flux ratio to give a total uncertainty.
Considering the decay of 56Co, the abundance of 56Fe will increase with time while the resulting Ni/Fe ratio will decrease accordingly. To avoid such an effect of temporal evolution, we scale the Ni/Fe ratio to t → ∞ under the assumption that all of the 56Fe comes from the final decay product of 56Ni; the contribution from other stable irons is negligible.
Fig. 6 shows the estimated mass ratio of Ni and Fe as a function of phase since maximum light. We notice that the Ni/Fe ratio inferred from nebular-phase spectra might increase with time, as seen in SN 2011fe, which is also discussed in Section 5.1. For those SNe Ia with multiple late-time spectra, we usually adopt the Ni/Fe ratios derived from the latest ones. The final results shown in Fig. 6 indicate that the inferred Ni/Fe ratios for about |$70 \pm 6~{{\ \rm per\ cent}}$| of our SN Ia sample favour the double-detonation sub-MCh model. Here, the proportion uncertainty is simply estimated by using a Monte Carlo method to vary the Ni/Fe ratios according to their uncertainty. This proportion is higher than that derived by Maguire et al. (2018), mainly because of the different pseudo-continuum adoption in the fitting. The remaining objects are in better agreement with DDT models (Seitenzahl et al. 2013) or have a critical Ni/Fe ratio lying between the above two models, except for SN 2015F and SN 2002bo which have too high Ni/Fe ratios. Tiwari et al. (2022) use late-time light curves to constrain the mass of SN Ia progenitors and find that SN 2015F is only consistent with the sub-MCh model, which suggests that its Ni/Fe ratio should be small. In addition, we note that the shapes of the 7300 Å feature are peculiar and cannot be well fit, so we exclude SN 2015F from the following analysis. For SN 2002bo, the red half of the 7300 Å feature seems much stronger than the blue half, compared with other objects, which leads to an Ni/Fe ratio that is much higher than the upper limit given by the models involved in our analysis. Thus, we also exclude SN 2002bo from our analysis.
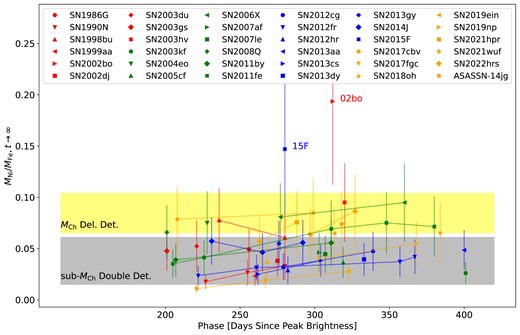
The inferred mass ratio of Ni and Fe as a function of phase after maximum light for SNe Ia collected in this work. The large error bars are mainly due to the ∼40 per cent relative uncertainty of the estimate of Maguire et al. (2018) that we adopt. Following Flörs et al. (2020), the Ni/Fe ratio range predicted for the DDT models (Seitenzahl et al. 2013) is shown by the yellow band and that of the sub-MCh models (Shen et al. 2018) is represented by the grey band. Both the Ni/Fe ratios measured from the observed spectra and those predicted by the explosion models are scaled to t → ∞ by assuming a rise time of ∼18 d (Ganeshalingam, Li & Filippenko 2011).
4.3 Connection between Ni/Fe ratios and Si velocities
To find some potential correlations between Ni/Fe ratios and early-time observed properties, we use the t ≈ 300 d spectra whenever possible, since the parameters that we adopt to infer the Ni/Fe ratio come from the W7 model at t ≈ 330 d (Fransson & Jerkstrand 2015). Note that t ≈ +300 d is the median of the phase range (∼+200–400 d) of our sample.
The distribution of the inferred Ni/Fe mass ratios and the Si ii λ6355 velocities measured around the time of maximum light is shown in Fig. 7, where one can see that the MNi/MFe ratio tends to increase with Si ii velocity. Moreover, the subsamples with redshifted or blueshifted nebular velocities seem to follow distinct MNi/MFe–vSi II relations. To test the robustness of the above correlation, we further use the scipy package’s stats.pearsonr function to calculate the Pearson coefficient. The correlations are found to be prominent for both the blueshifted and redshifted subsamples when not considering the error bars, with the r-values being 0.53 and 0.61, and the p-values being 0.03 and 0.03, respectively. When combining the whole sample, the correlation seems to be less prominent, with the r-value being only 0.32, and the p-value being 0.07. For the case including error bars, we assume a Gaussian distribution for the quoted uncertainties and repeat the calculation 20 001 times with a Monte Carlo method. If selecting the middle number of the Monte Carlo results, the significance of the correlations becomes more ambiguous, with the r-values being 0.32 and 0.38, and the p-values being 0.21 and 0.19, for the blueshifted and redshifted subsamples, respectively. Nevertheless, the connection is in agreement with some previous work and will be further discussed in Section 5.2.
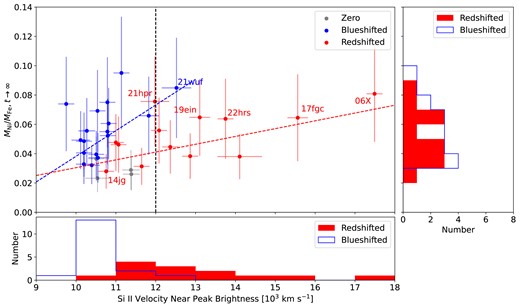
Inferred Ni/Fe mass ratio versus the Si ii 6355 Å velocity measured around the time of maximum light. SNe Ia that have redshifted or blueshifted nebular velocities are shown by red and blue circles, respectively, while those with nebular velocities close to zero are represented by grey dots. The red and blue dashed lines represent the best linear fits to the data points with redshifted and blueshifted nebular velocities, respectively. The horizontal and vertical histograms represent the distributions of Si velocities and Ni/Fe ratios.
From the histograms shown in Fig. 7, we can see that all SNe Ia with blueshifted Ni/Fe velocities in the nebular phase have Si velocities |$\lt 12\, 000$| km s−1 except for SN 2021wuf, while those with redshifted nebular velocities have Si velocities ranging from 10 000 to |$\sim 17\, 000$| km s−1. The range of the Ni/Fe ratio is similar for these two subgroups. We also notice that when the Si velocity is near 10 000 km s−1, the two groups with blueshifted and redshifted Ni/Fe velocities tend to mix.
4.4 Connection between the Ni/Fe ratio and Δm15(B)
Fig. 8 shows the relation between the Ni/Fe mass ratio and Δm15(B). Note that SNe Ia with blueshifted and redshifted nebular velocities tend to mix in the plot, in contrast to the distinct distribution seen in Fig. 7. The Ni/Fe mass ratio seems to increase with Δm15(B), with fast decliners having larger Ni/Fe mass ratio. However, this correlation reverses for SNe Ia with larger post-peak decline rates – that is, the SNe Ia with Δm15(B) > 1.7 mag have small Ni/Fe mass ratio. This discrepancy is likely related to the intrinsic difference existing between the SN 1991bg-like (SN 1986G and SN 2003gs in Fig. 8) and other SNe Ia. In addition, we caution about the low Ni/Fe ratio inferred for the overluminous SN 1991T-like SN 1999aa, which favours a sub-MCh explosion. Note that the sub-MCh detonation model of Shen et al. (2018) of a relatively massive WD (1.1 M⊙) shows lower luminosity than the overluminous SN Ia; thus, it seems to be hard for the sub-MCh model to produce the high luminosity.
We calculate the Pearson’s r-values for all of the sample shown in Fig. 8, except SN 1986G and SN 2003gs. We obtain an r-value of 0.68 and a p-value of 2.18 × 10−5 without considering the error bars, and an r-value of 0.43 and a p-value of 0.01 when including the error bars with the Monte Carlo method adopted in Section 4.3. These results strongly suggest that the inferred Ni/Fe mass ratio has a positive correlation with Δm15(B).
5 DISCUSSION
In this paper, we applied multicomponent Gaussian fits to the spectral features near 7300 Å for 36 SNe Ia. Nebular velocities and Ni/Fe mass ratios are inferred from the velocity shifts and flux ratios, respectively. In this section, we further discuss the evolution of the inferred Ni/Fe ratio, and we explore possible connections between inferred late- and early-time parameters and their implications for explosion mechanisms.
5.1 Evolution of the 7300 Å region
For the well-observed object SN 2011fe, we notice that the inferred Ni/Fe ratio shows temporal evolution, moving from the regions favouring the sub-MCh double-detonation model to that favouring the MCh delayed detonation model. The increasing trend is also found for other events having at least three spectra except for SN 2014J. Considering the uncertainties using a Monte Carlo method, the mean Pearson r-value derived for SNe 1990N, 2011fe, 2012fr, and ASASSN-14jg is 0.73 ± 0.11. Note that the variation inferred for the Ni/Fe ratio with phase only reflects the evolution of the flux ratio of [Ni ii] 7378 Å and [Fe ii] 7155 Å, since we use the same temperature and departure coefficients to infer the Ni/Fe ratio in all phases as Maguire et al. (2018) did. It should be noted that the errors associated with the Ni/Fe ratio shown in Fig. 6 are dominated by uncertainties in temperature and departure coefficients ratio, which might be overestimated when analysing the evolution for the same object. This is due to the fact that the temperature usually decreases with time while the departure coefficients increase with time for the same object, and the net evolution effect of these two parameters on the inferred Ni/Fe ratio is thus limited.
Note that we use the same temperature and ratio of departure coefficients to infer the Ni/Fe ratio, hence the evolution of the Ni/Fe ratio is due to the evolution of the flux ratio of [Ni ii] 7378 Å and [Fe ii] 7155 Å. This evolution is clearly indicated in Fig. 2, where the [Ni ii] 7378 Å feature tends to become increasingly prominent compared with the [Fe ii] 7155 Å feature, which might be due to the suppression of the [Ni ii] lines as a result of higher ionization of nickel in the inner ejecta (Blondin et al. 2022). As the inner ejecta cool gradually, the flux ratio of [Ni ii] to [Ni iii] increases with time, leading to the increase of the inferred Ni/Fe ratio. In fact, we also notice that the scaled flux of the 7900 Å region decreases with time and this region could have a contribution from [Ni iii] 7890 Å. Thus, the spectral evolution might indicate the recombination of [Ni iii], since the flux-density ratio of [Ni ii] 7378 Å and the 7900 Å region increases with time. Moreover, the high-quality spectra of SN 2011fe show that there exists a weak feature in the 7900 Å region, which nearly disappears at later phases.
Another possible reason for the increase of the flux ratio of [Ni ii] 7378 Å and [Fe ii] 7155 Å is that the [Fe ii] 7155 Å feature is contributed by the bump that gets weaker with time. Fig. 2 also shows the evolution of the spectra subtracting the pseudo-continuums defined by point A′, where the bump (likely related to some additional features that only contribute at early times) is subtracted. In this case, the trend that the flux ratio of [Ni ii] 7378 Å and [Fe ii] 7155 Å increase with time is still visible. But if the bump contributes little to the [Ni ii] 7378 Å feature, the strength of the [Ni ii] 7378 Å feature will be underestimated when subtracting the pseudo-continuums defined by point A′. A more careful fitting that includes some new emission lines related to the bump is needed to confirm the evolution, which is more complicated and beyond the scope of this paper.
Flörs et al. (2020) used an NLTE level population model to fit the full late-time spectra for their SN Ia sample and found no evident evolution for Ni/Fe ratios for a given object. In Fig. 9, we compare our inferred Ni/Fe ratios with those predicted by Flörs et al. (2020) for the same spectra. We also show the comparison of the Ni/Fe ratios between cases A and A′. The results from Flörs et al. (2020) and ours are in good agreement at phases t ≲ +300 d. When approaching t ≳ +300 d, our fits tend to give systematically larger Ni/Fe ratios. A possible interpretation is that the evolution of the Ni/Fe ratio disappears when more accurate temperatures, departure coefficients, and flux ratios are obtained through the NLTE calculations and full spectral fitting. But we notice that the 7900 Å region is not fit well by Flörs et al. (2020), which might also lead to the different results. For cases A and A′, the Ni/Fe mass ratios inferred from the spectra taken at t > +300 d are more similar, since the small bump at 6800–7000 Å that leads to the different pseudo-continuums becomes weak at such phases.
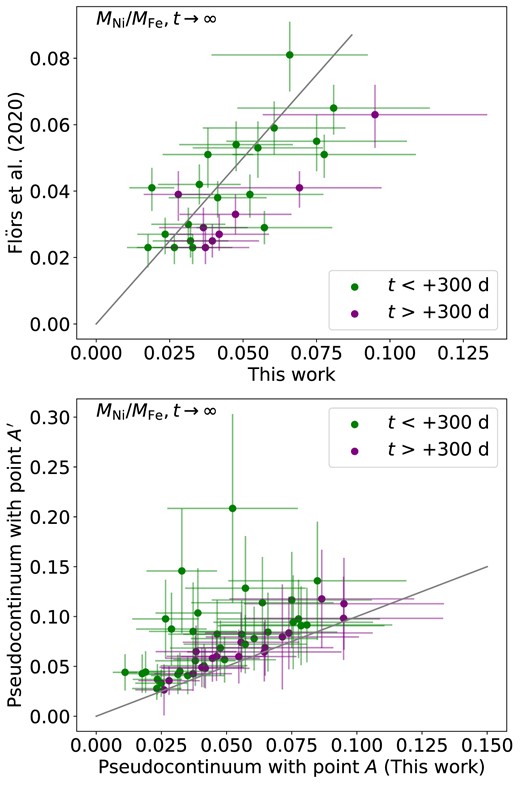
Comparisons of the Ni/Fe ratios given by different methods. The top panel compares our fits with those from the NLTE model of Flörs et al. (2020) for the same spectra. The labelled objects are those whose Ni/Fe ratios deviate by more than 1σ. The bottom panel compares the fits using point A, which defines a similar pseudo-continuum to that of Maguire et al. (2018), with the fits using point A′, which is adopted in this work. The Ni/Fe ratios inferred from the spectra taken at t < +300 d are shown with green dots, while those taken at t > +300 d are shown with purple dots.
It should be noted that the evolution of the inferred Ni/Fe ratio makes the distinction between MCh and sub-MCh models difficult. If the evolution is caused by suppression of [Ni ii], more-accurate estimates of the Ni/Fe ratio could be inferred from very late-phase spectra, at t ≳ +350 d when the minor emission on the left side of the ∼7300 Å feature disappears in the spectra. Considering such an evolutionary trend, a relatively lower Ni/Fe ratio inferred from earlier nebular-phase spectra (i.e. at t ≈ 250 d) should be used carefully to confine the explosion models. For our sample, 7 and 12 out of 19 SNe Ia are found to be more in favour of the MCh and sub-MCh models, respectively, if restricting the SN Ia sample to those with at least one spectrum at t > +300 d. The relative fractions (i.e. about 37 per cent for MCh and 63 per cent for sub-MCh) are consistent with the results derived for the whole sample. However, the proportion favouring an origin in sub-MCh explosions still could be overestimated because the inferred low Ni/Fe ratio might be affected by spectral evolution.
5.2 Implications for the variation of Si velocities
Maeda et al. (2010a) found that Si velocity gradients were correlated with nebular velocities, and they proposed an asymmetric and off-centre explosion toy model to interpret this correlation as a geometric effect. In particular, one will find low-velocity-gradient (LVG) SNe Ia when viewing from the direction of off-centre ignition and high-velocity-gradient (HVG) SNe Ia from the opposite direction. A similar correlation can be found between Si velocities at maximum light and nebular velocities (Silverman et al. 2013). Fig. 7 shows that almost all HV SNe Ia display a redshifted nebular velocity, in agreement with Silverman et al. (2013). Note that the HV subclass is not bound exactly to the HVG subclass; for example, SN 2021wuf can be put into the HV group, while its Si velocity gradient is relatively low (LVG). Nevertheless, to do the analysis we use the Si velocity around the time of maximum light instead of the Si velocity gradient, since the former parameter is more convenient to measure.
As indicated in Fig. 7, the two branches of SNe Ia characterized by redshifted or blueshifted nebular velocities show distinct distributions in the Ni/Fe ratio and Si ii velocity plane, especially at the larger velocity end where no blueshifted SNe Ia are found except for SN 2021wuf. The histogram distribution of the Ni/Fe ratio and Si ii velocity indicates that the separation between redshifted and blueshifted objects is caused mainly by Si velocity instead of the Ni/Fe ratio.
To examine whether the blueshifted and redshifted objects at lower velocities also come from different groups, we applied a 2D K-S test (Fasano & Franceschini 1987) and derived the p-value as 0.05. Such a small p-value suggests that the blueshifted and redshifted branches still show a significant difference. Nevertheless, we caution that this result may suffer from small-number statistics (especially for redshifted NV objects), and a larger sample is needed for further analysis. Note that the correlation between the Ni/Fe ratio and Si velocity is weak for the whole sample, with a Pearson r-value being 0.31 even when not considering the uncertainties. Thus, we focus on the subsamples with redshifted or blueshifted nebular velocities in the following analysis in this subsection.
In the study by Maeda et al. (2010a), the LVG SNe Ia displaying redshifted nebular velocities were not regarded as exceptions, and they suggested that SNe Ia of the LVG and HVG subclasses do not have intrinsic differences as long as the redshifted nebular velocity is not too large (i.e. ≲ 1000 km s−1). However, the possible LVG SN Ia ASASSN-14jg (Graham et al. 2017), with Si velocity ∼10 760 km s−1 at + 6 d, has an unusually large redshifted nebular velocity (∼1600 km s−1) relative to the expected upper limit of ∼1000 km s−1. This suggests that ASASSN-14jg could still be observed in the NV subclass when viewing from the opposite direction of off-centre ignition owing to its overall low Si velocity. Moreover, among the redshifted objects, ∼40 per cent are found to have normal Si velocity (i.e. |$\lesssim 12\, 000$| km s−1), which means that it is not rare that NV SNe Ia display redshifted nebular velocities. Thus, other factors are needed to interpret the variation of Si velocities.
On the other hand, Wang et al. (2013) found that HV SNe Ia tend to occur in inner regions of their host galaxies, arguing that the diversity of Si velocities in SNe Ia cannot be completely attributed to geometric effects and HV SNe Ia may be related to metal-rich stellar populations (Pan et al. 2015a; Pan 2020). This is consistent with the recent result that HV SNe Ia tend to have abundant circumstellar dust (Wang et al. 2019). The analysis of late-time spectral features presented in this paper also supports that HV SNe Ia may have metal-rich progenitors. Timmes et al. (2003) explored how the ratio of stable-to-radioactive nucleosynthetic products increases with the metallicity of SN progenitors. A higher ratio of stable-to-radioactive nucleosynthetic products means a higher late-time Ni/Fe ratio, since all of the radioactive 56Ni would decay to 56Co and finally 56Fe. This has also been discussed by Graham et al. (2022). If we only focus on the SNe Ia that have redshifted or blueshifted nebular velocities, the Ni/Fe ratio tends to increase with Si velocity, so the Si velocity has a positive correlation with metallicity. This correlation is also in agreement with the simulated result that the blueshift of Si ii absorption increases with the metallicity of the C + O layer of SN Ia progenitors (Lentz et al. 2000). Thus, the low Si velocity of ASASSN-14jg may be due to its low Ni/Fe ratio (low metallicity). However, we caution that whether the variation of metallicity can completely account for the observed variation of Si velocity is not clear, and more-detailed explosion simulations are needed in future work.
5.3 Sub-MCh models prefer smaller Δm15(B)?
As shown in Fig. 8, a positive correlation between the Ni/Fe ratio and Δm15(B) seems to exist for SNe Ia with Δm15 < 1.7 mag. A higher Ni/Fe ratio means a higher ratio of stable-to-radioactive nucleosynthetic products and hence a lower mass of synthetic radioactive 56Ni. The mass of radioactive 56Ni is a dominant parameter describing the explosion energy of SNe Ia, with larger 56Ni corresponding to a more energetic explosion and hence smaller Δm15(B). Thus, given a similar total mass of ejecta, a higher ratio of stable-to-radioactive nucleosynthetic products would naturally result in a fainter SN Ia with larger Δm15(B).
However, the positive correlation between the Ni/Fe ratio and Δm15(B) also implies that the sub-MCh models would tend to produce more-luminous SNe Ia than the MCh models, contradicting the previous understanding of explosion models. Considering the [Ni ii] suppression that we have discussed in Section 5.1, an alternative interpretation is that SNe Ia with smaller Δm15(B) might have more radioactive materials, which would ionize more nickel to Ni iii at late phases. This interpretation is supported by the finding of Blondin et al. (2022) that the strong [Ni ii] lines predicted by their MCh models can be completely suppressed when 56Ni is sufficiently mixed with the stable IGEs. Thus, the Ni/Fe ratio inferred from our methods would be underestimated for more-luminous SNe Ia. In other words, the positive correlation between the inferred Ni/Fe ratio and Δm15(B) is caused by different degrees of suppression of [Ni ii] features.
6 CONCLUSIONS
We have performed multicomponent Gaussian fits to the 7300 Å emission features (due to [Fe ii] and [Ni ii]) in the nebular spectra of 36 SNe Ia. This has allowed us to measure the velocity shifts and flux ratios of [Ni ii] and [Fe ii] features in this region, which can be used to infer nebular velocities and the late-time Ni/Fe ratio, respectively. The nebular velocity might be connected to the geometry of the explosion, while the Ni/Fe ratio can be used to distinguish sub-MCh and MCh models. Connecting these inferred late-time parameters with the early-time observations (Si ii λ6355 velocity at maximum light and Δm15(B)), we find some interesting correlations. Our main results are as follows.
The majority (about 67 per cent) of SNe Ia in this work favour sub-MCh models. However, this fraction could be overestimated owing to a potentially increasing trend of the inferred Ni/Fe ratio with SN age. Moreover, the increasing trend can be interpreted as the suppression of [Ni ii] lines as a result of higher ionization of nickel in the inner ejecta.
Although the whole sample do not show a prominent correlation between the inferred Ni/Fe ratio and Si velocity, a positive correlation tends to exist for subsamples with redshifted or blueshifted nebular velocities. This indicates that the progenitor metallicity should be at least partially responsible for the variation of Si velocity observed in SNe Ia.
The Ni/Fe ratio has a positive correlation with Δm15(B), except for the SN 1991bg-like SNe Ia. This correlation seems to suggest that sub-MCh explosions tend to produce more-luminous SNe Ia than MCh explosions, which violates common sense. However, this correlation could be caused by serious suppression of [Ni ii] (ionized to [Ni iii]) for luminous SNe Ia with smaller Δm15(B).
Late-time spectra reveal the inner region of SNe Ia and are important in studying the explosion properties. Connections between the inner region and the outer ejecta also have many implications for the study of explosion mechanisms. In future work, more high-quality late-time spectra are needed to confirm these connections and explore new correlations. Additional well-observed SNe Ia at late phases are also needed to study the evolution of late-time spectra and give more-accurate estimates of the Ni/Fe ratio to confine explosion models.
ACKNOWLEDGEMENTS
We thank the anonymous referee for his/her constructive comments which help improve the manuscript.
This work is supported by the National Science Foundation of China (NSFC grants 12288102, 12033003, 11633002, and 12090044), the Ma Huateng Foundation, the Scholar Program of Beijing Academy of Science and Technology (DZ:BS202002), and the Tencent Xplorer Prize. AVF’s team received support from the Christopher R. Redlich Fund, Gary and Cynthia Bengier, Clark and Sharon Winslow, Sanford Robertson, Alan Eustace, Briggs and Kathleen Wood, and many other donors. NER acknowledges partial support from MIUR, PRIN 2017 (grant 20179ZF5KS), PRIN-INAF 2022, the Spanish MICINN grant PID2019-108709GB-I00, and FEDER funds, and the program Unidad de Excelencia María de Maeztu CEX2020-001058-M.
We thank the staffs at the observatories where data were obtained. Some of the data presented herein were obtained at the W. M. Keck Observatory, which is operated as a scientific partnership among the California Institute of Technology, the University of California, and NASA; the observatory was made possible by the generous financial support of the W. M. Keck Foundation. A major upgrade of the Kast spectrograph on the Shane 3 m telescope at Lick Observatory, led by Brad Holden, was made possible through generous gifts from the Heising-Simons Foundation, William and Marina Kast, and the University of California Observatories. Research at Lick Observatory is partially supported by a generous gift from Google. Based in part on observations made with the GTC, installed in the Spanish Observatorio del Roque de los Muchachos of the Instituto de Astrofísica de Canarias, in the island of La Palma.
This work made use of the OSC (https://sne.space), SNDB (http://heracles.astro.berkeley.edu), and WISeREP (https://wiserep.weizmann.ac.il) databases. We have used the NED, which is funded by NASA and operated by the California Institute of Technology. This research utilized scipy (https://www.scipy.org), extinction (https://pypi.org/project/extinction), matplotlib (Hunter 2007), and numpy (https://numpy.org).
DATA AVAILABILITY
The data underlying this article are available in the article. Information on the spectra collected by our own project is presented in Table 1. Some basic information about the SNe Ia in this work is presented in Table A1. The fits for all spectra in this work are shown in Fig. A1, and the corresponding parameters are presented in Table A2.
Footnotes
IRAF is distributed by the National Optical Astronomy Observatories, which are operated by the Association of Universities for Research in Astronomy, Inc., under cooperative agreement with the National Science Foundation (NSF).
References
APPENDIX A: OVERVIEW OF SNE IA AND FITTING RESULTS
Best fits to the 7300 Å region dominated by [Fe ii] and [Ni ii] features. The reddening-corrected spectra are shown in grey, while the smoothed spectra are shown in black. The overall fits are shown in red, the [Fe ii] features are in purple dashed lines, and the [Ni ii] features are in green dashed lines. The [Ca ii] features for SN 1986G and SN 2003gs are in pink. The fitting regions are indicated with blue lines, and the pseudo-continuum is in yellow.
Name . | Typea . | Host galaxy . | Redshift . | |$A_V\, ^{b}$| . | Δm15(B) . | Si vel., Phase . | Date of max.c . | Ref.d . | Ref.e . | Ref. . |
---|---|---|---|---|---|---|---|---|---|---|
. | . | . | . | (mag) . | (mag) . | (1000 |$\rm km\ s^{-1}$|, d) . | UTC . | spec. . | LC . | AV . |
SN 2019ein | pec | NGC 5353 | 0.007755 | 0.033 | 1.35 ± 0.01 | 13.10 ± 0.26, + 0 | 20190516 | 1 | 2 | 3 |
SN 2019np | norm | NGC 3254 | 0.00452 | 0.054 | 1.04 ± 0.07 | 10.20 ± 0.20, + 0 | 20190127 | 4 | 4 | 3 |
SN 2021hpr | norm | NGC 3147 | 0.009346 | 0.065 | 1.0 ± 0.06 | 11.98 ± 0.36, + 1 | 20210418 | 5 | 5 | 3 |
SN 2021wuf | norm | NGC 6500/NGC 6501 | 0.01 | 0.241 | 1.11 ± 0.06 | 12.52 ± 0.37, + 1 | 20210905 | 6 | 6 | 3 |
SN 2022hrs | norm | NGC 4647 | 0.0047 | 0.744 | 1.11 ± 0.08 | 13.75 ± 0.20, + 0 | 20220429 | 7 | 7 | 7,3 |
SN 1986G | 91bg | NGC 5128 | 0.001825 | 2.79 | 1.73 ± 0.07 | 10.11 ± 0.23, + 0 | 19860511 | 8 | 9,10 | 9 |
SN 1990N | norm | NGC 4639 | 0.003369 | 0.069 | 1.03 ± 0.06 | 10.20 ± 0.20, + 2 | 19900710 | 11 | 12 | 3 |
SN 1998bu | norm | NGC 3368 | 0.002992 | 1.054 | 1.02 ± 0.04 | 10.82 ± 0.20, −1 | 19980519 | 13 | 14 | 14 |
SN 1999aa | 91T | NGC 2595 | 0.014907 | 0.106 | 0.75 ± 0.03 | 10.54 ± 0.31, −1 | 19990222 | 15 | 16 | 3 |
SN 2002bo | norm | NGC 3190 | 0.0043 | 1.333 | 1.13 ± 0.05 | 13.41 ± 0.31, + 0 | 20020323 | 17 | 17 | 17 |
SN 2002dj | norm | NGC 5018 | 0.009393 | 0.254 | 1.08 ± 0.05 | 14.11 ± 0.56, −3 | 20020624 | 18 | 18 | 3 |
SN 2003du | norm | NGC 9391 | 0.006408 | 0.027 | 1.04 ± 0.03 | 10.80 ± 0.20, + 0 | 20030506 | 19 | 20 | 3 |
SN 2003gs | 91bg | NGC 936 | 0.00477 | 0.094 | 1.83 ± 0.02 | 11.00 ± 0.20, + 2 | 20030728 | 21 | 22 | 3 |
SN 2003hv | norm | NGC 1201 | 0.005624 | 0.041 | 1.61 ± 0.02 | 11.14 ± 0.20, + 1 | 20030909 | 23 | 23 | 3 |
SN 2003kf | norm | MCG -02-16-002 | 0.0074 | 0.833 | 0.98 ± 0.07 | 11.39 ± 0.21, −3 | 20031207 | 24 | 25 | 3 |
SN 2004eo | norm | NGC 6928 | 0.015718 | 0.288 | 1.45 ± 0.04 | 10.79 ± 0.20, −3 | 20040930 | 26 | 26 | 3 |
SN 2005cf | norm | MCG -01-39-003 | 0.006461 | 0.261 | 1.05 ± 0.03 | 10.51 ± 0.20, + 0 | 20050612 | 27 | 28 | 3 |
SN 2006X | norm | NGC 4321 | 0.005294 | 2.172 | 1.17 ± 0.05 | 17.49 ± 0.20, + 0 | 20060219 | 29 | 29 | 29,3 |
SN 2007af | norm | NGC 5584 | 0.005464 | 0.104 | 1.2 ± 0.05 | 11.07 ± 0.20, + 0 | 20070314 | 24 | 30,25 | 3 |
SN 2007le | norm | NGC 7721 | 0.006721 | 0.71 | 1.02 ± 0.04 | 12.36 ± 0.20, + 3 | 20071025 | 31 | 32,33 | 32,3 |
SN 2008Q | norm | NGC 524 | 0.0081 | 0.221 | 1.39 ± 0.1 | 11.82 ± 0.26, + 0 | 20080209 | 24 | 34 | 3 |
SN 2011by | norm | NGC 3972 | 0.002843 | 0.037 | 1.14 ± 0.03 | 10.27 ± 0.21, + 1 | 20110509 | 33 | 33 | 3 |
SN 2011fe | norm | M 101 | 0.000804 | 0.024 | 1.18 ± 0.03 | 10.54 ± 0.20, + 0 | 20110910 | 35 | 36 | 3 |
SN 2012cg | 91T | NGC 4424 | 0.001458 | 0.62 | 0.86 ± 0.02 | 10.39 ± 0.20, + 3 | 20120603 | 37 | 37 | 38,3 |
SN 2012fr | norm | NGC 1365 | 0.004 | 0.054 | 0.85 ± 0.05 | 11.65 ± 0.20, + 0 | 20121112 | 39 | 40 | 3 |
SN 2012hr | norm | ESO 121-G026 | 0.008 | 0.121 | 1.1 ± 0.04 | 11.38 ± 0.22, + 5 | 20121228 | 41 | 42 | 3 |
SN 2013aa | norm | NGC 5643 | 0.003999 | 0.453 | 0.95 ± 0.01 | 10.20 ± 0.20, + 0 | 20130220 | 41 | 43 | 3 |
SN 2013cs | norm | ESO 576-G017 | 0.00924 | 0.248 | 1.07 ± 0.05 | 12.86 ± 0.20, + 1 | 20130525 | 44 | 45 | 3 |
SN 2013dy | norm | NGC 7250 | 0.00389 | 0.409 | 0.89 ± 0.01 | 10.51 ± 0.20, + 1 | 20130728 | 46 | 46 | 3 |
SN 2013gy | norm | NGC 1418 | 0.014023 | 0.154 | 1.23 ± 0.06 | 10.79 ± 0.20, −1 | 20131222 | 47 | 47 | 3 |
SN 2014J | norm | NGC 3034 | 0.000677 | 2.421 | 1.1 ± 0.02 | 12.08 ± 0.20, + 0 | 20140201 | 48 | 49 | 50,3 |
SN 2015F | norm | NGC 2442 | 0.0049 | 0.542 | 1.35 ± 0.03 | 10.44 ± 0.23, −3 | 20150325 | 51 | 52 | 3 |
SN 2017cbv | norm | NGC 5643 | 0.003999 | 0.45 | 0.99 ± 0.01 | 9.75 ± 0.20, −1 | 20170328 | 53 | 54 | 3 |
SN 2017fgc | norm | NGC 0474 | 0.008 | 0.091 | 1.05 ± 0.07 | 15.56 ± 0.26, + 1 | 20170725 | 55 | 56 | 3 |
SN 2018oh | norm | UGC 04780 | 0.012 | 0.12 | 0.96 ± 0.03 | 10.55 ± 0.24, + 0 | 20180213 | 57 | 57 | 3 |
ASASSN-14jg | norm | PGC 128348 | 0.0148 | 0.04 | 0.92 ± 0.01 | 10.76 ± 0.20, + 6 | 20141031 | 44 | 41 | 3 |
Name . | Typea . | Host galaxy . | Redshift . | |$A_V\, ^{b}$| . | Δm15(B) . | Si vel., Phase . | Date of max.c . | Ref.d . | Ref.e . | Ref. . |
---|---|---|---|---|---|---|---|---|---|---|
. | . | . | . | (mag) . | (mag) . | (1000 |$\rm km\ s^{-1}$|, d) . | UTC . | spec. . | LC . | AV . |
SN 2019ein | pec | NGC 5353 | 0.007755 | 0.033 | 1.35 ± 0.01 | 13.10 ± 0.26, + 0 | 20190516 | 1 | 2 | 3 |
SN 2019np | norm | NGC 3254 | 0.00452 | 0.054 | 1.04 ± 0.07 | 10.20 ± 0.20, + 0 | 20190127 | 4 | 4 | 3 |
SN 2021hpr | norm | NGC 3147 | 0.009346 | 0.065 | 1.0 ± 0.06 | 11.98 ± 0.36, + 1 | 20210418 | 5 | 5 | 3 |
SN 2021wuf | norm | NGC 6500/NGC 6501 | 0.01 | 0.241 | 1.11 ± 0.06 | 12.52 ± 0.37, + 1 | 20210905 | 6 | 6 | 3 |
SN 2022hrs | norm | NGC 4647 | 0.0047 | 0.744 | 1.11 ± 0.08 | 13.75 ± 0.20, + 0 | 20220429 | 7 | 7 | 7,3 |
SN 1986G | 91bg | NGC 5128 | 0.001825 | 2.79 | 1.73 ± 0.07 | 10.11 ± 0.23, + 0 | 19860511 | 8 | 9,10 | 9 |
SN 1990N | norm | NGC 4639 | 0.003369 | 0.069 | 1.03 ± 0.06 | 10.20 ± 0.20, + 2 | 19900710 | 11 | 12 | 3 |
SN 1998bu | norm | NGC 3368 | 0.002992 | 1.054 | 1.02 ± 0.04 | 10.82 ± 0.20, −1 | 19980519 | 13 | 14 | 14 |
SN 1999aa | 91T | NGC 2595 | 0.014907 | 0.106 | 0.75 ± 0.03 | 10.54 ± 0.31, −1 | 19990222 | 15 | 16 | 3 |
SN 2002bo | norm | NGC 3190 | 0.0043 | 1.333 | 1.13 ± 0.05 | 13.41 ± 0.31, + 0 | 20020323 | 17 | 17 | 17 |
SN 2002dj | norm | NGC 5018 | 0.009393 | 0.254 | 1.08 ± 0.05 | 14.11 ± 0.56, −3 | 20020624 | 18 | 18 | 3 |
SN 2003du | norm | NGC 9391 | 0.006408 | 0.027 | 1.04 ± 0.03 | 10.80 ± 0.20, + 0 | 20030506 | 19 | 20 | 3 |
SN 2003gs | 91bg | NGC 936 | 0.00477 | 0.094 | 1.83 ± 0.02 | 11.00 ± 0.20, + 2 | 20030728 | 21 | 22 | 3 |
SN 2003hv | norm | NGC 1201 | 0.005624 | 0.041 | 1.61 ± 0.02 | 11.14 ± 0.20, + 1 | 20030909 | 23 | 23 | 3 |
SN 2003kf | norm | MCG -02-16-002 | 0.0074 | 0.833 | 0.98 ± 0.07 | 11.39 ± 0.21, −3 | 20031207 | 24 | 25 | 3 |
SN 2004eo | norm | NGC 6928 | 0.015718 | 0.288 | 1.45 ± 0.04 | 10.79 ± 0.20, −3 | 20040930 | 26 | 26 | 3 |
SN 2005cf | norm | MCG -01-39-003 | 0.006461 | 0.261 | 1.05 ± 0.03 | 10.51 ± 0.20, + 0 | 20050612 | 27 | 28 | 3 |
SN 2006X | norm | NGC 4321 | 0.005294 | 2.172 | 1.17 ± 0.05 | 17.49 ± 0.20, + 0 | 20060219 | 29 | 29 | 29,3 |
SN 2007af | norm | NGC 5584 | 0.005464 | 0.104 | 1.2 ± 0.05 | 11.07 ± 0.20, + 0 | 20070314 | 24 | 30,25 | 3 |
SN 2007le | norm | NGC 7721 | 0.006721 | 0.71 | 1.02 ± 0.04 | 12.36 ± 0.20, + 3 | 20071025 | 31 | 32,33 | 32,3 |
SN 2008Q | norm | NGC 524 | 0.0081 | 0.221 | 1.39 ± 0.1 | 11.82 ± 0.26, + 0 | 20080209 | 24 | 34 | 3 |
SN 2011by | norm | NGC 3972 | 0.002843 | 0.037 | 1.14 ± 0.03 | 10.27 ± 0.21, + 1 | 20110509 | 33 | 33 | 3 |
SN 2011fe | norm | M 101 | 0.000804 | 0.024 | 1.18 ± 0.03 | 10.54 ± 0.20, + 0 | 20110910 | 35 | 36 | 3 |
SN 2012cg | 91T | NGC 4424 | 0.001458 | 0.62 | 0.86 ± 0.02 | 10.39 ± 0.20, + 3 | 20120603 | 37 | 37 | 38,3 |
SN 2012fr | norm | NGC 1365 | 0.004 | 0.054 | 0.85 ± 0.05 | 11.65 ± 0.20, + 0 | 20121112 | 39 | 40 | 3 |
SN 2012hr | norm | ESO 121-G026 | 0.008 | 0.121 | 1.1 ± 0.04 | 11.38 ± 0.22, + 5 | 20121228 | 41 | 42 | 3 |
SN 2013aa | norm | NGC 5643 | 0.003999 | 0.453 | 0.95 ± 0.01 | 10.20 ± 0.20, + 0 | 20130220 | 41 | 43 | 3 |
SN 2013cs | norm | ESO 576-G017 | 0.00924 | 0.248 | 1.07 ± 0.05 | 12.86 ± 0.20, + 1 | 20130525 | 44 | 45 | 3 |
SN 2013dy | norm | NGC 7250 | 0.00389 | 0.409 | 0.89 ± 0.01 | 10.51 ± 0.20, + 1 | 20130728 | 46 | 46 | 3 |
SN 2013gy | norm | NGC 1418 | 0.014023 | 0.154 | 1.23 ± 0.06 | 10.79 ± 0.20, −1 | 20131222 | 47 | 47 | 3 |
SN 2014J | norm | NGC 3034 | 0.000677 | 2.421 | 1.1 ± 0.02 | 12.08 ± 0.20, + 0 | 20140201 | 48 | 49 | 50,3 |
SN 2015F | norm | NGC 2442 | 0.0049 | 0.542 | 1.35 ± 0.03 | 10.44 ± 0.23, −3 | 20150325 | 51 | 52 | 3 |
SN 2017cbv | norm | NGC 5643 | 0.003999 | 0.45 | 0.99 ± 0.01 | 9.75 ± 0.20, −1 | 20170328 | 53 | 54 | 3 |
SN 2017fgc | norm | NGC 0474 | 0.008 | 0.091 | 1.05 ± 0.07 | 15.56 ± 0.26, + 1 | 20170725 | 55 | 56 | 3 |
SN 2018oh | norm | UGC 04780 | 0.012 | 0.12 | 0.96 ± 0.03 | 10.55 ± 0.24, + 0 | 20180213 | 57 | 57 | 3 |
ASASSN-14jg | norm | PGC 128348 | 0.0148 | 0.04 | 0.92 ± 0.01 | 10.76 ± 0.20, + 6 | 20141031 | 44 | 41 | 3 |
Notes. References: (1) Pellegrino et al. (2020); (2) Xi et al. (2022); (3) Schlafly & Finkbeiner (2011); (4) Sai et al. (2022); (5) Iskandar et al. (in preparation); (6) Zeng et al. (in preparation); (7) Liu et al. (in preparation); (8) Cristiani et al. (1992); (9) Phillips et al. (1987); (10) Phillips et al. (1999); (11) Mazzali et al. (1993); (12) Lira et al. (1998); (13) Matheson et al. (2008); (14) Jha et al. (1999); (15) Silverman et al. (2012a); (16) Krisciunas et al. (2000); (17) Benetti et al. (2004); (18) Pignata et al. (2008); (19) Gerardy (2005); (20) Anupama, Sahu & Jose (2005); (21) Evans et al. (2003); (22) Krisciunas et al. (2009); (23) Leloudas et al. (2009); (24) Blondin et al. (2012); (25) Hicken et al. (2009); (26) Pastorello et al. (2007); (27) Garavini et al. (2007); (28) Wang et al. (2009a); (29) Wang et al. (2008); (30) Simon et al. (2007); (31) Folatelli et al. (2013); (32) Simon et al. (2009); (33) Silverman et al. (2013); (34) Milne et al. (2010); (35) Pereira et al. (2013); (36) Zhang et al. (2016); (37) Marion et al. (2016); (38) Silverman et al. (2012b); (39) Childress et al. (2013); (40) Zhang et al. (2014); (41) Graham et al. (2017); (42) Brown et al. (2014); (43) Burns et al. (2020); (44) Childress et al. (2016); (45) Walker et al. (2015); (46) Pan et al. (2015b); (47) Holmbo et al. (2019); (48) Srivastav et al. (2016); (49) Li et al. (2019b); (50) Foley et al. (2014); (51) Foley et al. (2016); (52) Cartier et al. (2017); (53) Hosseinzadeh et al. (2017); (54) Wang et al. (2020); (55) Stahl et al. (2020); (56) Zeng et al. (2021); and (57) Li et al. (2019a). The sample from our own project are put at the top of the table and separated from the public sample by a line.
aThe type is estimated with SNID (Blondin & Tonry 2007) using the spectrum around the maximum light except for SN 2003gs, SN 2013aa, and SN 2019ein, whose classifications are taken from Krisciunas et al. (2009), Parker et al. (2013), and Burke et al. (2019), respectively.
bWe assume host RV = 3.1 except for SN 2006X, SN 2007le, and SN 2014J, whose host RV values are 1.48, 2.56, and 1.6, respectively.
cThe date of B-band maximum light used in this work.
dSources for the spectra used to calculate Si ii λ6355 velocities around the time of B-band maximum and identify the type. The Si ii λ6355 velocities of SN 2003gs and SN 2013aa are taken from Evans et al. (2003) and Graham et al. (2017), respectively.
eReferences for the date of B-band maximum and Δm15(B). For SN 2003kf, SN 2012hr, and SN 2013cs, these two parameters are measured in this work with SALT2 using the light curves from the references.
Name . | Typea . | Host galaxy . | Redshift . | |$A_V\, ^{b}$| . | Δm15(B) . | Si vel., Phase . | Date of max.c . | Ref.d . | Ref.e . | Ref. . |
---|---|---|---|---|---|---|---|---|---|---|
. | . | . | . | (mag) . | (mag) . | (1000 |$\rm km\ s^{-1}$|, d) . | UTC . | spec. . | LC . | AV . |
SN 2019ein | pec | NGC 5353 | 0.007755 | 0.033 | 1.35 ± 0.01 | 13.10 ± 0.26, + 0 | 20190516 | 1 | 2 | 3 |
SN 2019np | norm | NGC 3254 | 0.00452 | 0.054 | 1.04 ± 0.07 | 10.20 ± 0.20, + 0 | 20190127 | 4 | 4 | 3 |
SN 2021hpr | norm | NGC 3147 | 0.009346 | 0.065 | 1.0 ± 0.06 | 11.98 ± 0.36, + 1 | 20210418 | 5 | 5 | 3 |
SN 2021wuf | norm | NGC 6500/NGC 6501 | 0.01 | 0.241 | 1.11 ± 0.06 | 12.52 ± 0.37, + 1 | 20210905 | 6 | 6 | 3 |
SN 2022hrs | norm | NGC 4647 | 0.0047 | 0.744 | 1.11 ± 0.08 | 13.75 ± 0.20, + 0 | 20220429 | 7 | 7 | 7,3 |
SN 1986G | 91bg | NGC 5128 | 0.001825 | 2.79 | 1.73 ± 0.07 | 10.11 ± 0.23, + 0 | 19860511 | 8 | 9,10 | 9 |
SN 1990N | norm | NGC 4639 | 0.003369 | 0.069 | 1.03 ± 0.06 | 10.20 ± 0.20, + 2 | 19900710 | 11 | 12 | 3 |
SN 1998bu | norm | NGC 3368 | 0.002992 | 1.054 | 1.02 ± 0.04 | 10.82 ± 0.20, −1 | 19980519 | 13 | 14 | 14 |
SN 1999aa | 91T | NGC 2595 | 0.014907 | 0.106 | 0.75 ± 0.03 | 10.54 ± 0.31, −1 | 19990222 | 15 | 16 | 3 |
SN 2002bo | norm | NGC 3190 | 0.0043 | 1.333 | 1.13 ± 0.05 | 13.41 ± 0.31, + 0 | 20020323 | 17 | 17 | 17 |
SN 2002dj | norm | NGC 5018 | 0.009393 | 0.254 | 1.08 ± 0.05 | 14.11 ± 0.56, −3 | 20020624 | 18 | 18 | 3 |
SN 2003du | norm | NGC 9391 | 0.006408 | 0.027 | 1.04 ± 0.03 | 10.80 ± 0.20, + 0 | 20030506 | 19 | 20 | 3 |
SN 2003gs | 91bg | NGC 936 | 0.00477 | 0.094 | 1.83 ± 0.02 | 11.00 ± 0.20, + 2 | 20030728 | 21 | 22 | 3 |
SN 2003hv | norm | NGC 1201 | 0.005624 | 0.041 | 1.61 ± 0.02 | 11.14 ± 0.20, + 1 | 20030909 | 23 | 23 | 3 |
SN 2003kf | norm | MCG -02-16-002 | 0.0074 | 0.833 | 0.98 ± 0.07 | 11.39 ± 0.21, −3 | 20031207 | 24 | 25 | 3 |
SN 2004eo | norm | NGC 6928 | 0.015718 | 0.288 | 1.45 ± 0.04 | 10.79 ± 0.20, −3 | 20040930 | 26 | 26 | 3 |
SN 2005cf | norm | MCG -01-39-003 | 0.006461 | 0.261 | 1.05 ± 0.03 | 10.51 ± 0.20, + 0 | 20050612 | 27 | 28 | 3 |
SN 2006X | norm | NGC 4321 | 0.005294 | 2.172 | 1.17 ± 0.05 | 17.49 ± 0.20, + 0 | 20060219 | 29 | 29 | 29,3 |
SN 2007af | norm | NGC 5584 | 0.005464 | 0.104 | 1.2 ± 0.05 | 11.07 ± 0.20, + 0 | 20070314 | 24 | 30,25 | 3 |
SN 2007le | norm | NGC 7721 | 0.006721 | 0.71 | 1.02 ± 0.04 | 12.36 ± 0.20, + 3 | 20071025 | 31 | 32,33 | 32,3 |
SN 2008Q | norm | NGC 524 | 0.0081 | 0.221 | 1.39 ± 0.1 | 11.82 ± 0.26, + 0 | 20080209 | 24 | 34 | 3 |
SN 2011by | norm | NGC 3972 | 0.002843 | 0.037 | 1.14 ± 0.03 | 10.27 ± 0.21, + 1 | 20110509 | 33 | 33 | 3 |
SN 2011fe | norm | M 101 | 0.000804 | 0.024 | 1.18 ± 0.03 | 10.54 ± 0.20, + 0 | 20110910 | 35 | 36 | 3 |
SN 2012cg | 91T | NGC 4424 | 0.001458 | 0.62 | 0.86 ± 0.02 | 10.39 ± 0.20, + 3 | 20120603 | 37 | 37 | 38,3 |
SN 2012fr | norm | NGC 1365 | 0.004 | 0.054 | 0.85 ± 0.05 | 11.65 ± 0.20, + 0 | 20121112 | 39 | 40 | 3 |
SN 2012hr | norm | ESO 121-G026 | 0.008 | 0.121 | 1.1 ± 0.04 | 11.38 ± 0.22, + 5 | 20121228 | 41 | 42 | 3 |
SN 2013aa | norm | NGC 5643 | 0.003999 | 0.453 | 0.95 ± 0.01 | 10.20 ± 0.20, + 0 | 20130220 | 41 | 43 | 3 |
SN 2013cs | norm | ESO 576-G017 | 0.00924 | 0.248 | 1.07 ± 0.05 | 12.86 ± 0.20, + 1 | 20130525 | 44 | 45 | 3 |
SN 2013dy | norm | NGC 7250 | 0.00389 | 0.409 | 0.89 ± 0.01 | 10.51 ± 0.20, + 1 | 20130728 | 46 | 46 | 3 |
SN 2013gy | norm | NGC 1418 | 0.014023 | 0.154 | 1.23 ± 0.06 | 10.79 ± 0.20, −1 | 20131222 | 47 | 47 | 3 |
SN 2014J | norm | NGC 3034 | 0.000677 | 2.421 | 1.1 ± 0.02 | 12.08 ± 0.20, + 0 | 20140201 | 48 | 49 | 50,3 |
SN 2015F | norm | NGC 2442 | 0.0049 | 0.542 | 1.35 ± 0.03 | 10.44 ± 0.23, −3 | 20150325 | 51 | 52 | 3 |
SN 2017cbv | norm | NGC 5643 | 0.003999 | 0.45 | 0.99 ± 0.01 | 9.75 ± 0.20, −1 | 20170328 | 53 | 54 | 3 |
SN 2017fgc | norm | NGC 0474 | 0.008 | 0.091 | 1.05 ± 0.07 | 15.56 ± 0.26, + 1 | 20170725 | 55 | 56 | 3 |
SN 2018oh | norm | UGC 04780 | 0.012 | 0.12 | 0.96 ± 0.03 | 10.55 ± 0.24, + 0 | 20180213 | 57 | 57 | 3 |
ASASSN-14jg | norm | PGC 128348 | 0.0148 | 0.04 | 0.92 ± 0.01 | 10.76 ± 0.20, + 6 | 20141031 | 44 | 41 | 3 |
Name . | Typea . | Host galaxy . | Redshift . | |$A_V\, ^{b}$| . | Δm15(B) . | Si vel., Phase . | Date of max.c . | Ref.d . | Ref.e . | Ref. . |
---|---|---|---|---|---|---|---|---|---|---|
. | . | . | . | (mag) . | (mag) . | (1000 |$\rm km\ s^{-1}$|, d) . | UTC . | spec. . | LC . | AV . |
SN 2019ein | pec | NGC 5353 | 0.007755 | 0.033 | 1.35 ± 0.01 | 13.10 ± 0.26, + 0 | 20190516 | 1 | 2 | 3 |
SN 2019np | norm | NGC 3254 | 0.00452 | 0.054 | 1.04 ± 0.07 | 10.20 ± 0.20, + 0 | 20190127 | 4 | 4 | 3 |
SN 2021hpr | norm | NGC 3147 | 0.009346 | 0.065 | 1.0 ± 0.06 | 11.98 ± 0.36, + 1 | 20210418 | 5 | 5 | 3 |
SN 2021wuf | norm | NGC 6500/NGC 6501 | 0.01 | 0.241 | 1.11 ± 0.06 | 12.52 ± 0.37, + 1 | 20210905 | 6 | 6 | 3 |
SN 2022hrs | norm | NGC 4647 | 0.0047 | 0.744 | 1.11 ± 0.08 | 13.75 ± 0.20, + 0 | 20220429 | 7 | 7 | 7,3 |
SN 1986G | 91bg | NGC 5128 | 0.001825 | 2.79 | 1.73 ± 0.07 | 10.11 ± 0.23, + 0 | 19860511 | 8 | 9,10 | 9 |
SN 1990N | norm | NGC 4639 | 0.003369 | 0.069 | 1.03 ± 0.06 | 10.20 ± 0.20, + 2 | 19900710 | 11 | 12 | 3 |
SN 1998bu | norm | NGC 3368 | 0.002992 | 1.054 | 1.02 ± 0.04 | 10.82 ± 0.20, −1 | 19980519 | 13 | 14 | 14 |
SN 1999aa | 91T | NGC 2595 | 0.014907 | 0.106 | 0.75 ± 0.03 | 10.54 ± 0.31, −1 | 19990222 | 15 | 16 | 3 |
SN 2002bo | norm | NGC 3190 | 0.0043 | 1.333 | 1.13 ± 0.05 | 13.41 ± 0.31, + 0 | 20020323 | 17 | 17 | 17 |
SN 2002dj | norm | NGC 5018 | 0.009393 | 0.254 | 1.08 ± 0.05 | 14.11 ± 0.56, −3 | 20020624 | 18 | 18 | 3 |
SN 2003du | norm | NGC 9391 | 0.006408 | 0.027 | 1.04 ± 0.03 | 10.80 ± 0.20, + 0 | 20030506 | 19 | 20 | 3 |
SN 2003gs | 91bg | NGC 936 | 0.00477 | 0.094 | 1.83 ± 0.02 | 11.00 ± 0.20, + 2 | 20030728 | 21 | 22 | 3 |
SN 2003hv | norm | NGC 1201 | 0.005624 | 0.041 | 1.61 ± 0.02 | 11.14 ± 0.20, + 1 | 20030909 | 23 | 23 | 3 |
SN 2003kf | norm | MCG -02-16-002 | 0.0074 | 0.833 | 0.98 ± 0.07 | 11.39 ± 0.21, −3 | 20031207 | 24 | 25 | 3 |
SN 2004eo | norm | NGC 6928 | 0.015718 | 0.288 | 1.45 ± 0.04 | 10.79 ± 0.20, −3 | 20040930 | 26 | 26 | 3 |
SN 2005cf | norm | MCG -01-39-003 | 0.006461 | 0.261 | 1.05 ± 0.03 | 10.51 ± 0.20, + 0 | 20050612 | 27 | 28 | 3 |
SN 2006X | norm | NGC 4321 | 0.005294 | 2.172 | 1.17 ± 0.05 | 17.49 ± 0.20, + 0 | 20060219 | 29 | 29 | 29,3 |
SN 2007af | norm | NGC 5584 | 0.005464 | 0.104 | 1.2 ± 0.05 | 11.07 ± 0.20, + 0 | 20070314 | 24 | 30,25 | 3 |
SN 2007le | norm | NGC 7721 | 0.006721 | 0.71 | 1.02 ± 0.04 | 12.36 ± 0.20, + 3 | 20071025 | 31 | 32,33 | 32,3 |
SN 2008Q | norm | NGC 524 | 0.0081 | 0.221 | 1.39 ± 0.1 | 11.82 ± 0.26, + 0 | 20080209 | 24 | 34 | 3 |
SN 2011by | norm | NGC 3972 | 0.002843 | 0.037 | 1.14 ± 0.03 | 10.27 ± 0.21, + 1 | 20110509 | 33 | 33 | 3 |
SN 2011fe | norm | M 101 | 0.000804 | 0.024 | 1.18 ± 0.03 | 10.54 ± 0.20, + 0 | 20110910 | 35 | 36 | 3 |
SN 2012cg | 91T | NGC 4424 | 0.001458 | 0.62 | 0.86 ± 0.02 | 10.39 ± 0.20, + 3 | 20120603 | 37 | 37 | 38,3 |
SN 2012fr | norm | NGC 1365 | 0.004 | 0.054 | 0.85 ± 0.05 | 11.65 ± 0.20, + 0 | 20121112 | 39 | 40 | 3 |
SN 2012hr | norm | ESO 121-G026 | 0.008 | 0.121 | 1.1 ± 0.04 | 11.38 ± 0.22, + 5 | 20121228 | 41 | 42 | 3 |
SN 2013aa | norm | NGC 5643 | 0.003999 | 0.453 | 0.95 ± 0.01 | 10.20 ± 0.20, + 0 | 20130220 | 41 | 43 | 3 |
SN 2013cs | norm | ESO 576-G017 | 0.00924 | 0.248 | 1.07 ± 0.05 | 12.86 ± 0.20, + 1 | 20130525 | 44 | 45 | 3 |
SN 2013dy | norm | NGC 7250 | 0.00389 | 0.409 | 0.89 ± 0.01 | 10.51 ± 0.20, + 1 | 20130728 | 46 | 46 | 3 |
SN 2013gy | norm | NGC 1418 | 0.014023 | 0.154 | 1.23 ± 0.06 | 10.79 ± 0.20, −1 | 20131222 | 47 | 47 | 3 |
SN 2014J | norm | NGC 3034 | 0.000677 | 2.421 | 1.1 ± 0.02 | 12.08 ± 0.20, + 0 | 20140201 | 48 | 49 | 50,3 |
SN 2015F | norm | NGC 2442 | 0.0049 | 0.542 | 1.35 ± 0.03 | 10.44 ± 0.23, −3 | 20150325 | 51 | 52 | 3 |
SN 2017cbv | norm | NGC 5643 | 0.003999 | 0.45 | 0.99 ± 0.01 | 9.75 ± 0.20, −1 | 20170328 | 53 | 54 | 3 |
SN 2017fgc | norm | NGC 0474 | 0.008 | 0.091 | 1.05 ± 0.07 | 15.56 ± 0.26, + 1 | 20170725 | 55 | 56 | 3 |
SN 2018oh | norm | UGC 04780 | 0.012 | 0.12 | 0.96 ± 0.03 | 10.55 ± 0.24, + 0 | 20180213 | 57 | 57 | 3 |
ASASSN-14jg | norm | PGC 128348 | 0.0148 | 0.04 | 0.92 ± 0.01 | 10.76 ± 0.20, + 6 | 20141031 | 44 | 41 | 3 |
Notes. References: (1) Pellegrino et al. (2020); (2) Xi et al. (2022); (3) Schlafly & Finkbeiner (2011); (4) Sai et al. (2022); (5) Iskandar et al. (in preparation); (6) Zeng et al. (in preparation); (7) Liu et al. (in preparation); (8) Cristiani et al. (1992); (9) Phillips et al. (1987); (10) Phillips et al. (1999); (11) Mazzali et al. (1993); (12) Lira et al. (1998); (13) Matheson et al. (2008); (14) Jha et al. (1999); (15) Silverman et al. (2012a); (16) Krisciunas et al. (2000); (17) Benetti et al. (2004); (18) Pignata et al. (2008); (19) Gerardy (2005); (20) Anupama, Sahu & Jose (2005); (21) Evans et al. (2003); (22) Krisciunas et al. (2009); (23) Leloudas et al. (2009); (24) Blondin et al. (2012); (25) Hicken et al. (2009); (26) Pastorello et al. (2007); (27) Garavini et al. (2007); (28) Wang et al. (2009a); (29) Wang et al. (2008); (30) Simon et al. (2007); (31) Folatelli et al. (2013); (32) Simon et al. (2009); (33) Silverman et al. (2013); (34) Milne et al. (2010); (35) Pereira et al. (2013); (36) Zhang et al. (2016); (37) Marion et al. (2016); (38) Silverman et al. (2012b); (39) Childress et al. (2013); (40) Zhang et al. (2014); (41) Graham et al. (2017); (42) Brown et al. (2014); (43) Burns et al. (2020); (44) Childress et al. (2016); (45) Walker et al. (2015); (46) Pan et al. (2015b); (47) Holmbo et al. (2019); (48) Srivastav et al. (2016); (49) Li et al. (2019b); (50) Foley et al. (2014); (51) Foley et al. (2016); (52) Cartier et al. (2017); (53) Hosseinzadeh et al. (2017); (54) Wang et al. (2020); (55) Stahl et al. (2020); (56) Zeng et al. (2021); and (57) Li et al. (2019a). The sample from our own project are put at the top of the table and separated from the public sample by a line.
aThe type is estimated with SNID (Blondin & Tonry 2007) using the spectrum around the maximum light except for SN 2003gs, SN 2013aa, and SN 2019ein, whose classifications are taken from Krisciunas et al. (2009), Parker et al. (2013), and Burke et al. (2019), respectively.
bWe assume host RV = 3.1 except for SN 2006X, SN 2007le, and SN 2014J, whose host RV values are 1.48, 2.56, and 1.6, respectively.
cThe date of B-band maximum light used in this work.
dSources for the spectra used to calculate Si ii λ6355 velocities around the time of B-band maximum and identify the type. The Si ii λ6355 velocities of SN 2003gs and SN 2013aa are taken from Evans et al. (2003) and Graham et al. (2017), respectively.
eReferences for the date of B-band maximum and Δm15(B). For SN 2003kf, SN 2012hr, and SN 2013cs, these two parameters are measured in this work with SALT2 using the light curves from the references.
Multicomponent Gaussian-fit parameters of nebular-phase emission lines, and implications.
Name . | Phase . | [Fe ii] Vel. . | [Ni ii] Vel. . | [Fe ii] FWHM . | [Ni ii] FWHM . | Flux ratio . | Nebular vel. . | MNi/MFe . | Ref. . |
---|---|---|---|---|---|---|---|---|---|
. | (d) . | (km s−1) . | (km s−1) . | (km s−1) . | (km s−1) . | λ7155/λ7378 . | (km s−1) . | t → ∞ . | spec. . |
SN 2019ein | + 313 | −11 ± 623 | 2436 ± 326 | 12531 ± 434 | 4368 ± 379 | 0.370 ± 0.023 | 2436 ± 326 | 0.065 ± 0.026 | 1 |
SN 2019np | + 303 | −1700 ± 227 | −3258 ± 412 | 6980 ± 80 | 5520 ± 240 | 0.233 ± 0.008 | −1700 ± 227 | 0.041 ± 0.016 | 2 |
SN 2019np | + 368 | −1526 ± 318 | −3057 ± 404 | 7128 ± 331 | 5639 ± 79 | 0.305 ± 0.018 | −1526 ± 318 | 0.055 ± 0.022 | 2 |
SN 2021hpr | + 263 | 790 ± 249 | 125 ± 325 | 7453 ± 626 | 5366 ± 236 | 0.338 ± 0.036 | 790 ± 249 | 0.057 ± 0.024 | 3 |
SN 2021hpr | + 288 | 623 ± 231 | 676 ± 295 | 8363 ± 222 | 5998 ± 82 | 0.438 ± 0.028 | 623 ± 231 | 0.076 ± 0.031 | 3 |
SN 2021wuf | + 208 | −3226 ± 351 | −2776 ± 242 | 7691 ± 162 | 4597 ± 207 | 0.494 ± 0.038 | −2776 ± 242 | 0.079 ± 0.032 | 4 |
SN 2021wuf | + 299 | −3040 ± 467 | −2503 ± 362 | 9977 ± 144 | 4880 ± 266 | 0.488 ± 0.022 | −2503 ± 362 | 0.085 ± 0.034 | 4 |
SN 2022hrs | + 297 | 628 ± 554 | 777 ± 403 | 9064 ± 213 | 6097 ± 359 | 0.367 ± 0.057 | 628 ± 554 | 0.064 ± 0.027 | 5 |
SN 2022hrs | + 327 | 890 ± 498 | 739 ± 657 | 8212 ± 515 | 6510 ± 357 | 0.491 ± 0.044 | 890 ± 498 | 0.087 ± 0.035 | 5 |
SN 1986Ga | + 256 | −965 ± 237 | −965 ± 237 | 8109 ± 163 | 3569 ± 393 | 0.293 ± 0.005 | −965 ± 237 | 0.049 ± 0.020 | 6 |
SN 1990N | + 227 | −1696 ± 236 | −3606 ± 323 | 8875 ± 192 | 6472 ± 103 | 0.108 ± 0.007 | −1696 ± 236 | 0.018 ± 0.007 | 7 |
SN 1990N | + 255 | −1354 ± 274 | −1827 ± 569 | 9268 ± 272 | 6655 ± 806 | 0.158 ± 0.042 | −1354 ± 274 | 0.027 ± 0.013 | 7 |
SN 1990N | + 280 | −1315 ± 229 | −1968 ± 418 | 9017 ± 114 | 8165 ± 358 | 0.191 ± 0.018 | −1315 ± 229 | 0.033 ± 0.014 | 7 |
SN 1998bu | + 236 | −1322 ± 227 | −1604 ± 272 | 8198 ± 68 | 5533 ± 319 | 0.471 ± 0.020 | −1322 ± 227 | 0.078 ± 0.031 | 8 |
SN 1998bu | + 280 | −1429 ± 265 | −1686 ± 289 | 8393 ± 437 | 4824 ± 303 | 0.353 ± 0.009 | −1429 ± 265 | 0.061 ± 0.024 | 8 |
SN 1999aa | + 260 | 114 ± 263 | −209 ± 277 | 8030 ± 100 | 3392 ± 211 | 0.138 ± 0.009 | 114 ± 263 | 0.023 ± 0.009 | 8 |
SN 2002bo | + 312 | 1251 ± 278 | 1859 ± 324 | 7505 ± 579 | 8452 ± 668 | 1.105 ± 0.143 | 1251 ± 278 | 0.194 ± 0.081 | 9 |
SN 2002dj | + 275 | 1224 ± 276 | 2202 ± 334 | 9535 ± 210 | 5445 ± 208 | 0.222 ± 0.015 | 1224 ± 276 | 0.038 ± 0.015 | 10 |
SN 2003du | + 221 | −2001 ± 292 | −3024 ± 741 | 8112 ± 355 | 8075 ± 803 | 0.323 ± 0.085 | −2001 ± 292 | 0.052 ± 0.025 | 11 |
SN 2003gsa | + 201 | 807 ± 258 | 807 ± 258 | 9687 ± 212 | 4932 ± 87 | 0.303 ± 0.021 | 807 ± 258 | 0.048 ± 0.019 | 8 |
SN 2003hv | + 320 | −2575 ± 218 | −3688 ± 257 | 8525 ± 225 | 4984 ± 107 | 0.541 ± 0.030 | −3688 ± 257 | 0.095 ± 0.038 | 12 |
SN 2003kf | + 401 | 302 ± 396 | 115 ± 728 | 10651 ± 212 | 4944 ± 1585 | 0.144 ± 0.021 | 302 ± 396 | 0.026 ± 0.011 | 9 |
SN 2004eo | + 228 | −994 ± 249 | −2266 ± 352 | 8329 ± 149 | 8556 ± 325 | 0.459 ± 0.040 | −994 ± 249 | 0.075 ± 0.031 | 13 |
SN 2005cf | + 319 | 67 ± 237 | −84 ± 1647 | 8574 ± 1106 | 6790 ± 461 | 0.208 ± 0.021 | 67 ± 237 | 0.036 ± 0.015 | 14 |
SN 2006X | + 277 | 2298 ± 277 | 2248 ± 237 | 8052 ± 114 | 5434 ± 117 | 0.472 ± 0.031 | 2298 ± 277 | 0.081 ± 0.033 | 15 |
SN 2006X | + 360 | 2731 ± 235 | 2488 ± 244 | 9182 ± 212 | 5272 ± 72 | 0.531 ± 0.024 | 2731 ± 235 | 0.095 ± 0.038 | 8 |
SN 2007af | + 303 | 421 ± 226 | 367 ± 349 | 7744 ± 367 | 5775 ± 223 | 0.265 ± 0.029 | 421 ± 226 | 0.046 ± 0.019 | 9 |
SN 2007le | + 307 | 1497 ± 246 | 1614 ± 293 | 8809 ± 287 | 5238 ± 77 | 0.255 ± 0.019 | 1497 ± 246 | 0.045 ± 0.018 | 8 |
SN 2008Q | + 201 | −2239 ± 318 | −1485 ± 228 | 11131 ± 225 | 4765 ± 29 | 0.419 ± 0.024 | −1485 ± 228 | 0.066 ± 0.027 | 8 |
SN 2011by | + 207 | −1536 ± 277 | −2845 ± 488 | 7675 ± 278 | 5547 ± 435 | 0.246 ± 0.029 | −1536 ± 277 | 0.039 ± 0.016 | 16 |
SN 2011by | + 311 | −1066 ± 212 | −1716 ± 243 | 7970 ± 155 | 5080 ± 40 | 0.318 ± 0.013 | −1066 ± 212 | 0.056 ± 0.022 | 16 |
SN 2011fe | + 205 | −1143 ± 210 | −2217 ± 241 | 8423 ± 78 | 5606 ± 56 | 0.222 ± 0.007 | −1143 ± 210 | 0.035 ± 0.014 | 17 |
SN 2011fe | + 226 | −1027 ± 229 | −2077 ± 308 | 8148 ± 128 | 5709 ± 145 | 0.254 ± 0.012 | −1027 ± 229 | 0.041 ± 0.017 | 18 |
SN 2011fe | + 311 | −1068 ± 251 | −1077 ± 332 | 8329 ± 215 | 7072 ± 224 | 0.395 ± 0.022 | −1068 ± 251 | 0.069 ± 0.028 | 18 |
SN 2011fe | + 348 | −638 ± 251 | −1297 ± 284 | 8507 ± 107 | 6922 ± 126 | 0.422 ± 0.014 | −638 ± 251 | 0.075 ± 0.030 | 19 |
SN 2011fe | + 380 | −340 ± 297 | −1047 ± 356 | 9051 ± 291 | 6980 ± 226 | 0.397 ± 0.038 | −340 ± 297 | 0.071 ± 0.029 | 18 |
SN 2012cg | + 279 | −1070 ± 223 | −1830 ± 354 | 7813 ± 164 | 5603 ± 94 | 0.187 ± 0.005 | −1070 ± 223 | 0.032 ± 0.013 | 20 |
SN 2012cg | + 339 | −1111 ± 234 | −841 ± 314 | 8716 ± 101 | 7279 ± 141 | 0.267 ± 0.010 | −1111 ± 234 | 0.047 ± 0.019 | 21 |
SN 2012fr | + 222 | 2041 ± 264 | 2926 ± 397 | 8033 ± 93 | 5650 ± 155 | 0.145 ± 0.007 | 2041 ± 264 | 0.023 ± 0.009 | 22 |
SN 2012fr | + 261 | 2030 ± 219 | 2305 ± 793 | 7338 ± 785 | 5583 ± 308 | 0.185 ± 0.007 | 2030 ± 219 | 0.031 ± 0.013 | 22 |
SN 2012fr | + 357 | 2205 ± 226 | 2633 ± 226 | 8059 ± 52 | 5393 ± 55 | 0.208 ± 0.006 | 2205 ± 226 | 0.037 ± 0.015 | 21 |
SN 2012fr | + 367 | 2085 ± 248 | 3303 ± 235 | 8799 ± 267 | 4567 ± 40 | 0.234 ± 0.014 | 2085 ± 248 | 0.042 ± 0.017 | 22 |
SN 2012hr | + 282 | 133 ± 241 | 72 ± 641 | 8342 ± 349 | 7553 ± 863 | 0.168 ± 0.041 | 133 ± 241 | 0.029 ± 0.014 | 23 |
SN 2013aa | + 400 | −691 ± 229 | −1072 ± 260 | 8237 ± 113 | 6666 ± 171 | 0.268 ± 0.012 | −691 ± 229 | 0.048 ± 0.020 | 23 |
SN 2013cs | + 262 | 1474 ± 234 | 2622 ± 246 | 9232 ± 68 | 4838 ± 74 | 0.147 ± 0.003 | 1474 ± 234 | 0.025 ± 0.010 | 23 |
SN 2013cs | + 304 | 1343 ± 245 | 1452 ± 327 | 8179 ± 170 | 6069 ± 140 | 0.220 ± 0.012 | 1343 ± 245 | 0.038 ± 0.015 | 21 |
SN 2013dy | + 333 | −1161 ± 222 | −1478 ± 273 | 7664 ± 97 | 6722 ± 220 | 0.224 ± 0.010 | −1161 ± 222 | 0.040 ± 0.016 | 24 |
SN 2013gy | + 276 | −367 ± 213 | −438 ± 402 | 8200 ± 186 | 7044 ± 259 | 0.322 ± 0.015 | −367 ± 213 | 0.055 ± 0.022 | 23 |
SN 2014J | + 231 | 336 ± 213 | 673 ± 252 | 8291 ± 88 | 9370 ± 229 | 0.349 ± 0.019 | 336 ± 213 | 0.057 ± 0.023 | 22 |
SN 2014J | + 265 | 465 ± 213 | 354 ± 280 | 8071 ± 116 | 7682 ± 175 | 0.273 ± 0.016 | 465 ± 213 | 0.046 ± 0.019 | 18 |
SN 2014J | + 292 | 581 ± 232 | 239 ± 356 | 7826 ± 108 | 7671 ± 136 | 0.323 ± 0.012 | 581 ± 232 | 0.056 ± 0.022 | 18 |
SN 2015F | + 280 | −545 ± 303 | −1233 ± 254 | 7269 ± 154 | 9694 ± 799 | 0.857 ± 0.136 | −545 ± 303 | 0.147 ± 0.063 | 23 |
SN 2017cbvb | + 318 | −1279 ± 294 | −1279 ± 294 | 7826 ± 293 | 10293 ± 958 | 0.421 ± 0.072 | −1279 ± 294 | 0.074 ± 0.032 | 25 |
SN 2017fgc | + 384 | 2026 ± 319 | 2112 ± 444 | 8603 ± 798 | 6175 ± 642 | 0.358 ± 0.081 | 2026 ± 319 | 0.064 ± 0.030 | 26 |
SN 2018oh | + 268 | −1852 ± 376 | −3934 ± 813 | 7017 ± 344 | 5299 ± 662 | 0.219 ± 0.048 | −1852 ± 376 | 0.037 ± 0.017 | 25 |
ASASSN-14jg | + 221 | 1326 ± 274 | 1113 ± 283 | 8065 ± 78 | 3666 ± 411 | 0.068 ± 0.010 | 1326 ± 274 | 0.011 ± 0.005 | 27 |
ASASSN-14jg | + 267 | 1612 ± 244 | 984 ± 560 | 7662 ± 62 | 5537 ± 437 | 0.111 ± 0.005 | 1612 ± 244 | 0.019 ± 0.008 | 23 |
ASASSN-14jg | + 323 | 1674 ± 261 | 2236 ± 596 | 8406 ± 778 | 4992 ± 552 | 0.159 ± 0.021 | 1674 ± 261 | 0.028 ± 0.012 | 21 |
Name . | Phase . | [Fe ii] Vel. . | [Ni ii] Vel. . | [Fe ii] FWHM . | [Ni ii] FWHM . | Flux ratio . | Nebular vel. . | MNi/MFe . | Ref. . |
---|---|---|---|---|---|---|---|---|---|
. | (d) . | (km s−1) . | (km s−1) . | (km s−1) . | (km s−1) . | λ7155/λ7378 . | (km s−1) . | t → ∞ . | spec. . |
SN 2019ein | + 313 | −11 ± 623 | 2436 ± 326 | 12531 ± 434 | 4368 ± 379 | 0.370 ± 0.023 | 2436 ± 326 | 0.065 ± 0.026 | 1 |
SN 2019np | + 303 | −1700 ± 227 | −3258 ± 412 | 6980 ± 80 | 5520 ± 240 | 0.233 ± 0.008 | −1700 ± 227 | 0.041 ± 0.016 | 2 |
SN 2019np | + 368 | −1526 ± 318 | −3057 ± 404 | 7128 ± 331 | 5639 ± 79 | 0.305 ± 0.018 | −1526 ± 318 | 0.055 ± 0.022 | 2 |
SN 2021hpr | + 263 | 790 ± 249 | 125 ± 325 | 7453 ± 626 | 5366 ± 236 | 0.338 ± 0.036 | 790 ± 249 | 0.057 ± 0.024 | 3 |
SN 2021hpr | + 288 | 623 ± 231 | 676 ± 295 | 8363 ± 222 | 5998 ± 82 | 0.438 ± 0.028 | 623 ± 231 | 0.076 ± 0.031 | 3 |
SN 2021wuf | + 208 | −3226 ± 351 | −2776 ± 242 | 7691 ± 162 | 4597 ± 207 | 0.494 ± 0.038 | −2776 ± 242 | 0.079 ± 0.032 | 4 |
SN 2021wuf | + 299 | −3040 ± 467 | −2503 ± 362 | 9977 ± 144 | 4880 ± 266 | 0.488 ± 0.022 | −2503 ± 362 | 0.085 ± 0.034 | 4 |
SN 2022hrs | + 297 | 628 ± 554 | 777 ± 403 | 9064 ± 213 | 6097 ± 359 | 0.367 ± 0.057 | 628 ± 554 | 0.064 ± 0.027 | 5 |
SN 2022hrs | + 327 | 890 ± 498 | 739 ± 657 | 8212 ± 515 | 6510 ± 357 | 0.491 ± 0.044 | 890 ± 498 | 0.087 ± 0.035 | 5 |
SN 1986Ga | + 256 | −965 ± 237 | −965 ± 237 | 8109 ± 163 | 3569 ± 393 | 0.293 ± 0.005 | −965 ± 237 | 0.049 ± 0.020 | 6 |
SN 1990N | + 227 | −1696 ± 236 | −3606 ± 323 | 8875 ± 192 | 6472 ± 103 | 0.108 ± 0.007 | −1696 ± 236 | 0.018 ± 0.007 | 7 |
SN 1990N | + 255 | −1354 ± 274 | −1827 ± 569 | 9268 ± 272 | 6655 ± 806 | 0.158 ± 0.042 | −1354 ± 274 | 0.027 ± 0.013 | 7 |
SN 1990N | + 280 | −1315 ± 229 | −1968 ± 418 | 9017 ± 114 | 8165 ± 358 | 0.191 ± 0.018 | −1315 ± 229 | 0.033 ± 0.014 | 7 |
SN 1998bu | + 236 | −1322 ± 227 | −1604 ± 272 | 8198 ± 68 | 5533 ± 319 | 0.471 ± 0.020 | −1322 ± 227 | 0.078 ± 0.031 | 8 |
SN 1998bu | + 280 | −1429 ± 265 | −1686 ± 289 | 8393 ± 437 | 4824 ± 303 | 0.353 ± 0.009 | −1429 ± 265 | 0.061 ± 0.024 | 8 |
SN 1999aa | + 260 | 114 ± 263 | −209 ± 277 | 8030 ± 100 | 3392 ± 211 | 0.138 ± 0.009 | 114 ± 263 | 0.023 ± 0.009 | 8 |
SN 2002bo | + 312 | 1251 ± 278 | 1859 ± 324 | 7505 ± 579 | 8452 ± 668 | 1.105 ± 0.143 | 1251 ± 278 | 0.194 ± 0.081 | 9 |
SN 2002dj | + 275 | 1224 ± 276 | 2202 ± 334 | 9535 ± 210 | 5445 ± 208 | 0.222 ± 0.015 | 1224 ± 276 | 0.038 ± 0.015 | 10 |
SN 2003du | + 221 | −2001 ± 292 | −3024 ± 741 | 8112 ± 355 | 8075 ± 803 | 0.323 ± 0.085 | −2001 ± 292 | 0.052 ± 0.025 | 11 |
SN 2003gsa | + 201 | 807 ± 258 | 807 ± 258 | 9687 ± 212 | 4932 ± 87 | 0.303 ± 0.021 | 807 ± 258 | 0.048 ± 0.019 | 8 |
SN 2003hv | + 320 | −2575 ± 218 | −3688 ± 257 | 8525 ± 225 | 4984 ± 107 | 0.541 ± 0.030 | −3688 ± 257 | 0.095 ± 0.038 | 12 |
SN 2003kf | + 401 | 302 ± 396 | 115 ± 728 | 10651 ± 212 | 4944 ± 1585 | 0.144 ± 0.021 | 302 ± 396 | 0.026 ± 0.011 | 9 |
SN 2004eo | + 228 | −994 ± 249 | −2266 ± 352 | 8329 ± 149 | 8556 ± 325 | 0.459 ± 0.040 | −994 ± 249 | 0.075 ± 0.031 | 13 |
SN 2005cf | + 319 | 67 ± 237 | −84 ± 1647 | 8574 ± 1106 | 6790 ± 461 | 0.208 ± 0.021 | 67 ± 237 | 0.036 ± 0.015 | 14 |
SN 2006X | + 277 | 2298 ± 277 | 2248 ± 237 | 8052 ± 114 | 5434 ± 117 | 0.472 ± 0.031 | 2298 ± 277 | 0.081 ± 0.033 | 15 |
SN 2006X | + 360 | 2731 ± 235 | 2488 ± 244 | 9182 ± 212 | 5272 ± 72 | 0.531 ± 0.024 | 2731 ± 235 | 0.095 ± 0.038 | 8 |
SN 2007af | + 303 | 421 ± 226 | 367 ± 349 | 7744 ± 367 | 5775 ± 223 | 0.265 ± 0.029 | 421 ± 226 | 0.046 ± 0.019 | 9 |
SN 2007le | + 307 | 1497 ± 246 | 1614 ± 293 | 8809 ± 287 | 5238 ± 77 | 0.255 ± 0.019 | 1497 ± 246 | 0.045 ± 0.018 | 8 |
SN 2008Q | + 201 | −2239 ± 318 | −1485 ± 228 | 11131 ± 225 | 4765 ± 29 | 0.419 ± 0.024 | −1485 ± 228 | 0.066 ± 0.027 | 8 |
SN 2011by | + 207 | −1536 ± 277 | −2845 ± 488 | 7675 ± 278 | 5547 ± 435 | 0.246 ± 0.029 | −1536 ± 277 | 0.039 ± 0.016 | 16 |
SN 2011by | + 311 | −1066 ± 212 | −1716 ± 243 | 7970 ± 155 | 5080 ± 40 | 0.318 ± 0.013 | −1066 ± 212 | 0.056 ± 0.022 | 16 |
SN 2011fe | + 205 | −1143 ± 210 | −2217 ± 241 | 8423 ± 78 | 5606 ± 56 | 0.222 ± 0.007 | −1143 ± 210 | 0.035 ± 0.014 | 17 |
SN 2011fe | + 226 | −1027 ± 229 | −2077 ± 308 | 8148 ± 128 | 5709 ± 145 | 0.254 ± 0.012 | −1027 ± 229 | 0.041 ± 0.017 | 18 |
SN 2011fe | + 311 | −1068 ± 251 | −1077 ± 332 | 8329 ± 215 | 7072 ± 224 | 0.395 ± 0.022 | −1068 ± 251 | 0.069 ± 0.028 | 18 |
SN 2011fe | + 348 | −638 ± 251 | −1297 ± 284 | 8507 ± 107 | 6922 ± 126 | 0.422 ± 0.014 | −638 ± 251 | 0.075 ± 0.030 | 19 |
SN 2011fe | + 380 | −340 ± 297 | −1047 ± 356 | 9051 ± 291 | 6980 ± 226 | 0.397 ± 0.038 | −340 ± 297 | 0.071 ± 0.029 | 18 |
SN 2012cg | + 279 | −1070 ± 223 | −1830 ± 354 | 7813 ± 164 | 5603 ± 94 | 0.187 ± 0.005 | −1070 ± 223 | 0.032 ± 0.013 | 20 |
SN 2012cg | + 339 | −1111 ± 234 | −841 ± 314 | 8716 ± 101 | 7279 ± 141 | 0.267 ± 0.010 | −1111 ± 234 | 0.047 ± 0.019 | 21 |
SN 2012fr | + 222 | 2041 ± 264 | 2926 ± 397 | 8033 ± 93 | 5650 ± 155 | 0.145 ± 0.007 | 2041 ± 264 | 0.023 ± 0.009 | 22 |
SN 2012fr | + 261 | 2030 ± 219 | 2305 ± 793 | 7338 ± 785 | 5583 ± 308 | 0.185 ± 0.007 | 2030 ± 219 | 0.031 ± 0.013 | 22 |
SN 2012fr | + 357 | 2205 ± 226 | 2633 ± 226 | 8059 ± 52 | 5393 ± 55 | 0.208 ± 0.006 | 2205 ± 226 | 0.037 ± 0.015 | 21 |
SN 2012fr | + 367 | 2085 ± 248 | 3303 ± 235 | 8799 ± 267 | 4567 ± 40 | 0.234 ± 0.014 | 2085 ± 248 | 0.042 ± 0.017 | 22 |
SN 2012hr | + 282 | 133 ± 241 | 72 ± 641 | 8342 ± 349 | 7553 ± 863 | 0.168 ± 0.041 | 133 ± 241 | 0.029 ± 0.014 | 23 |
SN 2013aa | + 400 | −691 ± 229 | −1072 ± 260 | 8237 ± 113 | 6666 ± 171 | 0.268 ± 0.012 | −691 ± 229 | 0.048 ± 0.020 | 23 |
SN 2013cs | + 262 | 1474 ± 234 | 2622 ± 246 | 9232 ± 68 | 4838 ± 74 | 0.147 ± 0.003 | 1474 ± 234 | 0.025 ± 0.010 | 23 |
SN 2013cs | + 304 | 1343 ± 245 | 1452 ± 327 | 8179 ± 170 | 6069 ± 140 | 0.220 ± 0.012 | 1343 ± 245 | 0.038 ± 0.015 | 21 |
SN 2013dy | + 333 | −1161 ± 222 | −1478 ± 273 | 7664 ± 97 | 6722 ± 220 | 0.224 ± 0.010 | −1161 ± 222 | 0.040 ± 0.016 | 24 |
SN 2013gy | + 276 | −367 ± 213 | −438 ± 402 | 8200 ± 186 | 7044 ± 259 | 0.322 ± 0.015 | −367 ± 213 | 0.055 ± 0.022 | 23 |
SN 2014J | + 231 | 336 ± 213 | 673 ± 252 | 8291 ± 88 | 9370 ± 229 | 0.349 ± 0.019 | 336 ± 213 | 0.057 ± 0.023 | 22 |
SN 2014J | + 265 | 465 ± 213 | 354 ± 280 | 8071 ± 116 | 7682 ± 175 | 0.273 ± 0.016 | 465 ± 213 | 0.046 ± 0.019 | 18 |
SN 2014J | + 292 | 581 ± 232 | 239 ± 356 | 7826 ± 108 | 7671 ± 136 | 0.323 ± 0.012 | 581 ± 232 | 0.056 ± 0.022 | 18 |
SN 2015F | + 280 | −545 ± 303 | −1233 ± 254 | 7269 ± 154 | 9694 ± 799 | 0.857 ± 0.136 | −545 ± 303 | 0.147 ± 0.063 | 23 |
SN 2017cbvb | + 318 | −1279 ± 294 | −1279 ± 294 | 7826 ± 293 | 10293 ± 958 | 0.421 ± 0.072 | −1279 ± 294 | 0.074 ± 0.032 | 25 |
SN 2017fgc | + 384 | 2026 ± 319 | 2112 ± 444 | 8603 ± 798 | 6175 ± 642 | 0.358 ± 0.081 | 2026 ± 319 | 0.064 ± 0.030 | 26 |
SN 2018oh | + 268 | −1852 ± 376 | −3934 ± 813 | 7017 ± 344 | 5299 ± 662 | 0.219 ± 0.048 | −1852 ± 376 | 0.037 ± 0.017 | 25 |
ASASSN-14jg | + 221 | 1326 ± 274 | 1113 ± 283 | 8065 ± 78 | 3666 ± 411 | 0.068 ± 0.010 | 1326 ± 274 | 0.011 ± 0.005 | 27 |
ASASSN-14jg | + 267 | 1612 ± 244 | 984 ± 560 | 7662 ± 62 | 5537 ± 437 | 0.111 ± 0.005 | 1612 ± 244 | 0.019 ± 0.008 | 23 |
ASASSN-14jg | + 323 | 1674 ± 261 | 2236 ± 596 | 8406 ± 778 | 4992 ± 552 | 0.159 ± 0.021 | 1674 ± 261 | 0.028 ± 0.012 | 21 |
Notes. References: (1) Xi et al. (2022); (2) Sai et al. (2022); (3) Iskandar et al. (in preparation); (4) Zeng et al. (in preparation); (5) Liu et al. (in preparation); (6) Cristiani et al. (1992); (7) Gómez & López (1998); (8) Silverman et al. (2012a); (9) Blondin et al. (2012); (10) Pignata et al. (2008); (11) Stanishev et al. (2007); (12) Leloudas et al. (2009); (13) Pastorello et al. (2007); (14) Wang et al. (2009a); (15) Wang et al. (2008); (16) Silverman et al. (2013); (17) Mazzali et al. (2015); (18) Stahl et al. (2020); (19) Graham et al. (2015); (20) Amanullah et al. (2015); (21) Maguire et al. (2018); (22) Childress et al. (2015); (23) Graham et al. (2017); (24) Pan et al. (2015b); (25) Tucker, Shappee & Wisniewski (2019); (26) Zeng et al. (2021); and (27) Tucker et al. (2020). The sample from our own project is put at the top of the table and separated from the public sample by a line.
aThe velocity shifts of iron and nickel features are set to the same values, and [Ca ii] emission lines are added to the fits.
bThe velocity shifts of iron and nickel features are set to the same values.
Multicomponent Gaussian-fit parameters of nebular-phase emission lines, and implications.
Name . | Phase . | [Fe ii] Vel. . | [Ni ii] Vel. . | [Fe ii] FWHM . | [Ni ii] FWHM . | Flux ratio . | Nebular vel. . | MNi/MFe . | Ref. . |
---|---|---|---|---|---|---|---|---|---|
. | (d) . | (km s−1) . | (km s−1) . | (km s−1) . | (km s−1) . | λ7155/λ7378 . | (km s−1) . | t → ∞ . | spec. . |
SN 2019ein | + 313 | −11 ± 623 | 2436 ± 326 | 12531 ± 434 | 4368 ± 379 | 0.370 ± 0.023 | 2436 ± 326 | 0.065 ± 0.026 | 1 |
SN 2019np | + 303 | −1700 ± 227 | −3258 ± 412 | 6980 ± 80 | 5520 ± 240 | 0.233 ± 0.008 | −1700 ± 227 | 0.041 ± 0.016 | 2 |
SN 2019np | + 368 | −1526 ± 318 | −3057 ± 404 | 7128 ± 331 | 5639 ± 79 | 0.305 ± 0.018 | −1526 ± 318 | 0.055 ± 0.022 | 2 |
SN 2021hpr | + 263 | 790 ± 249 | 125 ± 325 | 7453 ± 626 | 5366 ± 236 | 0.338 ± 0.036 | 790 ± 249 | 0.057 ± 0.024 | 3 |
SN 2021hpr | + 288 | 623 ± 231 | 676 ± 295 | 8363 ± 222 | 5998 ± 82 | 0.438 ± 0.028 | 623 ± 231 | 0.076 ± 0.031 | 3 |
SN 2021wuf | + 208 | −3226 ± 351 | −2776 ± 242 | 7691 ± 162 | 4597 ± 207 | 0.494 ± 0.038 | −2776 ± 242 | 0.079 ± 0.032 | 4 |
SN 2021wuf | + 299 | −3040 ± 467 | −2503 ± 362 | 9977 ± 144 | 4880 ± 266 | 0.488 ± 0.022 | −2503 ± 362 | 0.085 ± 0.034 | 4 |
SN 2022hrs | + 297 | 628 ± 554 | 777 ± 403 | 9064 ± 213 | 6097 ± 359 | 0.367 ± 0.057 | 628 ± 554 | 0.064 ± 0.027 | 5 |
SN 2022hrs | + 327 | 890 ± 498 | 739 ± 657 | 8212 ± 515 | 6510 ± 357 | 0.491 ± 0.044 | 890 ± 498 | 0.087 ± 0.035 | 5 |
SN 1986Ga | + 256 | −965 ± 237 | −965 ± 237 | 8109 ± 163 | 3569 ± 393 | 0.293 ± 0.005 | −965 ± 237 | 0.049 ± 0.020 | 6 |
SN 1990N | + 227 | −1696 ± 236 | −3606 ± 323 | 8875 ± 192 | 6472 ± 103 | 0.108 ± 0.007 | −1696 ± 236 | 0.018 ± 0.007 | 7 |
SN 1990N | + 255 | −1354 ± 274 | −1827 ± 569 | 9268 ± 272 | 6655 ± 806 | 0.158 ± 0.042 | −1354 ± 274 | 0.027 ± 0.013 | 7 |
SN 1990N | + 280 | −1315 ± 229 | −1968 ± 418 | 9017 ± 114 | 8165 ± 358 | 0.191 ± 0.018 | −1315 ± 229 | 0.033 ± 0.014 | 7 |
SN 1998bu | + 236 | −1322 ± 227 | −1604 ± 272 | 8198 ± 68 | 5533 ± 319 | 0.471 ± 0.020 | −1322 ± 227 | 0.078 ± 0.031 | 8 |
SN 1998bu | + 280 | −1429 ± 265 | −1686 ± 289 | 8393 ± 437 | 4824 ± 303 | 0.353 ± 0.009 | −1429 ± 265 | 0.061 ± 0.024 | 8 |
SN 1999aa | + 260 | 114 ± 263 | −209 ± 277 | 8030 ± 100 | 3392 ± 211 | 0.138 ± 0.009 | 114 ± 263 | 0.023 ± 0.009 | 8 |
SN 2002bo | + 312 | 1251 ± 278 | 1859 ± 324 | 7505 ± 579 | 8452 ± 668 | 1.105 ± 0.143 | 1251 ± 278 | 0.194 ± 0.081 | 9 |
SN 2002dj | + 275 | 1224 ± 276 | 2202 ± 334 | 9535 ± 210 | 5445 ± 208 | 0.222 ± 0.015 | 1224 ± 276 | 0.038 ± 0.015 | 10 |
SN 2003du | + 221 | −2001 ± 292 | −3024 ± 741 | 8112 ± 355 | 8075 ± 803 | 0.323 ± 0.085 | −2001 ± 292 | 0.052 ± 0.025 | 11 |
SN 2003gsa | + 201 | 807 ± 258 | 807 ± 258 | 9687 ± 212 | 4932 ± 87 | 0.303 ± 0.021 | 807 ± 258 | 0.048 ± 0.019 | 8 |
SN 2003hv | + 320 | −2575 ± 218 | −3688 ± 257 | 8525 ± 225 | 4984 ± 107 | 0.541 ± 0.030 | −3688 ± 257 | 0.095 ± 0.038 | 12 |
SN 2003kf | + 401 | 302 ± 396 | 115 ± 728 | 10651 ± 212 | 4944 ± 1585 | 0.144 ± 0.021 | 302 ± 396 | 0.026 ± 0.011 | 9 |
SN 2004eo | + 228 | −994 ± 249 | −2266 ± 352 | 8329 ± 149 | 8556 ± 325 | 0.459 ± 0.040 | −994 ± 249 | 0.075 ± 0.031 | 13 |
SN 2005cf | + 319 | 67 ± 237 | −84 ± 1647 | 8574 ± 1106 | 6790 ± 461 | 0.208 ± 0.021 | 67 ± 237 | 0.036 ± 0.015 | 14 |
SN 2006X | + 277 | 2298 ± 277 | 2248 ± 237 | 8052 ± 114 | 5434 ± 117 | 0.472 ± 0.031 | 2298 ± 277 | 0.081 ± 0.033 | 15 |
SN 2006X | + 360 | 2731 ± 235 | 2488 ± 244 | 9182 ± 212 | 5272 ± 72 | 0.531 ± 0.024 | 2731 ± 235 | 0.095 ± 0.038 | 8 |
SN 2007af | + 303 | 421 ± 226 | 367 ± 349 | 7744 ± 367 | 5775 ± 223 | 0.265 ± 0.029 | 421 ± 226 | 0.046 ± 0.019 | 9 |
SN 2007le | + 307 | 1497 ± 246 | 1614 ± 293 | 8809 ± 287 | 5238 ± 77 | 0.255 ± 0.019 | 1497 ± 246 | 0.045 ± 0.018 | 8 |
SN 2008Q | + 201 | −2239 ± 318 | −1485 ± 228 | 11131 ± 225 | 4765 ± 29 | 0.419 ± 0.024 | −1485 ± 228 | 0.066 ± 0.027 | 8 |
SN 2011by | + 207 | −1536 ± 277 | −2845 ± 488 | 7675 ± 278 | 5547 ± 435 | 0.246 ± 0.029 | −1536 ± 277 | 0.039 ± 0.016 | 16 |
SN 2011by | + 311 | −1066 ± 212 | −1716 ± 243 | 7970 ± 155 | 5080 ± 40 | 0.318 ± 0.013 | −1066 ± 212 | 0.056 ± 0.022 | 16 |
SN 2011fe | + 205 | −1143 ± 210 | −2217 ± 241 | 8423 ± 78 | 5606 ± 56 | 0.222 ± 0.007 | −1143 ± 210 | 0.035 ± 0.014 | 17 |
SN 2011fe | + 226 | −1027 ± 229 | −2077 ± 308 | 8148 ± 128 | 5709 ± 145 | 0.254 ± 0.012 | −1027 ± 229 | 0.041 ± 0.017 | 18 |
SN 2011fe | + 311 | −1068 ± 251 | −1077 ± 332 | 8329 ± 215 | 7072 ± 224 | 0.395 ± 0.022 | −1068 ± 251 | 0.069 ± 0.028 | 18 |
SN 2011fe | + 348 | −638 ± 251 | −1297 ± 284 | 8507 ± 107 | 6922 ± 126 | 0.422 ± 0.014 | −638 ± 251 | 0.075 ± 0.030 | 19 |
SN 2011fe | + 380 | −340 ± 297 | −1047 ± 356 | 9051 ± 291 | 6980 ± 226 | 0.397 ± 0.038 | −340 ± 297 | 0.071 ± 0.029 | 18 |
SN 2012cg | + 279 | −1070 ± 223 | −1830 ± 354 | 7813 ± 164 | 5603 ± 94 | 0.187 ± 0.005 | −1070 ± 223 | 0.032 ± 0.013 | 20 |
SN 2012cg | + 339 | −1111 ± 234 | −841 ± 314 | 8716 ± 101 | 7279 ± 141 | 0.267 ± 0.010 | −1111 ± 234 | 0.047 ± 0.019 | 21 |
SN 2012fr | + 222 | 2041 ± 264 | 2926 ± 397 | 8033 ± 93 | 5650 ± 155 | 0.145 ± 0.007 | 2041 ± 264 | 0.023 ± 0.009 | 22 |
SN 2012fr | + 261 | 2030 ± 219 | 2305 ± 793 | 7338 ± 785 | 5583 ± 308 | 0.185 ± 0.007 | 2030 ± 219 | 0.031 ± 0.013 | 22 |
SN 2012fr | + 357 | 2205 ± 226 | 2633 ± 226 | 8059 ± 52 | 5393 ± 55 | 0.208 ± 0.006 | 2205 ± 226 | 0.037 ± 0.015 | 21 |
SN 2012fr | + 367 | 2085 ± 248 | 3303 ± 235 | 8799 ± 267 | 4567 ± 40 | 0.234 ± 0.014 | 2085 ± 248 | 0.042 ± 0.017 | 22 |
SN 2012hr | + 282 | 133 ± 241 | 72 ± 641 | 8342 ± 349 | 7553 ± 863 | 0.168 ± 0.041 | 133 ± 241 | 0.029 ± 0.014 | 23 |
SN 2013aa | + 400 | −691 ± 229 | −1072 ± 260 | 8237 ± 113 | 6666 ± 171 | 0.268 ± 0.012 | −691 ± 229 | 0.048 ± 0.020 | 23 |
SN 2013cs | + 262 | 1474 ± 234 | 2622 ± 246 | 9232 ± 68 | 4838 ± 74 | 0.147 ± 0.003 | 1474 ± 234 | 0.025 ± 0.010 | 23 |
SN 2013cs | + 304 | 1343 ± 245 | 1452 ± 327 | 8179 ± 170 | 6069 ± 140 | 0.220 ± 0.012 | 1343 ± 245 | 0.038 ± 0.015 | 21 |
SN 2013dy | + 333 | −1161 ± 222 | −1478 ± 273 | 7664 ± 97 | 6722 ± 220 | 0.224 ± 0.010 | −1161 ± 222 | 0.040 ± 0.016 | 24 |
SN 2013gy | + 276 | −367 ± 213 | −438 ± 402 | 8200 ± 186 | 7044 ± 259 | 0.322 ± 0.015 | −367 ± 213 | 0.055 ± 0.022 | 23 |
SN 2014J | + 231 | 336 ± 213 | 673 ± 252 | 8291 ± 88 | 9370 ± 229 | 0.349 ± 0.019 | 336 ± 213 | 0.057 ± 0.023 | 22 |
SN 2014J | + 265 | 465 ± 213 | 354 ± 280 | 8071 ± 116 | 7682 ± 175 | 0.273 ± 0.016 | 465 ± 213 | 0.046 ± 0.019 | 18 |
SN 2014J | + 292 | 581 ± 232 | 239 ± 356 | 7826 ± 108 | 7671 ± 136 | 0.323 ± 0.012 | 581 ± 232 | 0.056 ± 0.022 | 18 |
SN 2015F | + 280 | −545 ± 303 | −1233 ± 254 | 7269 ± 154 | 9694 ± 799 | 0.857 ± 0.136 | −545 ± 303 | 0.147 ± 0.063 | 23 |
SN 2017cbvb | + 318 | −1279 ± 294 | −1279 ± 294 | 7826 ± 293 | 10293 ± 958 | 0.421 ± 0.072 | −1279 ± 294 | 0.074 ± 0.032 | 25 |
SN 2017fgc | + 384 | 2026 ± 319 | 2112 ± 444 | 8603 ± 798 | 6175 ± 642 | 0.358 ± 0.081 | 2026 ± 319 | 0.064 ± 0.030 | 26 |
SN 2018oh | + 268 | −1852 ± 376 | −3934 ± 813 | 7017 ± 344 | 5299 ± 662 | 0.219 ± 0.048 | −1852 ± 376 | 0.037 ± 0.017 | 25 |
ASASSN-14jg | + 221 | 1326 ± 274 | 1113 ± 283 | 8065 ± 78 | 3666 ± 411 | 0.068 ± 0.010 | 1326 ± 274 | 0.011 ± 0.005 | 27 |
ASASSN-14jg | + 267 | 1612 ± 244 | 984 ± 560 | 7662 ± 62 | 5537 ± 437 | 0.111 ± 0.005 | 1612 ± 244 | 0.019 ± 0.008 | 23 |
ASASSN-14jg | + 323 | 1674 ± 261 | 2236 ± 596 | 8406 ± 778 | 4992 ± 552 | 0.159 ± 0.021 | 1674 ± 261 | 0.028 ± 0.012 | 21 |
Name . | Phase . | [Fe ii] Vel. . | [Ni ii] Vel. . | [Fe ii] FWHM . | [Ni ii] FWHM . | Flux ratio . | Nebular vel. . | MNi/MFe . | Ref. . |
---|---|---|---|---|---|---|---|---|---|
. | (d) . | (km s−1) . | (km s−1) . | (km s−1) . | (km s−1) . | λ7155/λ7378 . | (km s−1) . | t → ∞ . | spec. . |
SN 2019ein | + 313 | −11 ± 623 | 2436 ± 326 | 12531 ± 434 | 4368 ± 379 | 0.370 ± 0.023 | 2436 ± 326 | 0.065 ± 0.026 | 1 |
SN 2019np | + 303 | −1700 ± 227 | −3258 ± 412 | 6980 ± 80 | 5520 ± 240 | 0.233 ± 0.008 | −1700 ± 227 | 0.041 ± 0.016 | 2 |
SN 2019np | + 368 | −1526 ± 318 | −3057 ± 404 | 7128 ± 331 | 5639 ± 79 | 0.305 ± 0.018 | −1526 ± 318 | 0.055 ± 0.022 | 2 |
SN 2021hpr | + 263 | 790 ± 249 | 125 ± 325 | 7453 ± 626 | 5366 ± 236 | 0.338 ± 0.036 | 790 ± 249 | 0.057 ± 0.024 | 3 |
SN 2021hpr | + 288 | 623 ± 231 | 676 ± 295 | 8363 ± 222 | 5998 ± 82 | 0.438 ± 0.028 | 623 ± 231 | 0.076 ± 0.031 | 3 |
SN 2021wuf | + 208 | −3226 ± 351 | −2776 ± 242 | 7691 ± 162 | 4597 ± 207 | 0.494 ± 0.038 | −2776 ± 242 | 0.079 ± 0.032 | 4 |
SN 2021wuf | + 299 | −3040 ± 467 | −2503 ± 362 | 9977 ± 144 | 4880 ± 266 | 0.488 ± 0.022 | −2503 ± 362 | 0.085 ± 0.034 | 4 |
SN 2022hrs | + 297 | 628 ± 554 | 777 ± 403 | 9064 ± 213 | 6097 ± 359 | 0.367 ± 0.057 | 628 ± 554 | 0.064 ± 0.027 | 5 |
SN 2022hrs | + 327 | 890 ± 498 | 739 ± 657 | 8212 ± 515 | 6510 ± 357 | 0.491 ± 0.044 | 890 ± 498 | 0.087 ± 0.035 | 5 |
SN 1986Ga | + 256 | −965 ± 237 | −965 ± 237 | 8109 ± 163 | 3569 ± 393 | 0.293 ± 0.005 | −965 ± 237 | 0.049 ± 0.020 | 6 |
SN 1990N | + 227 | −1696 ± 236 | −3606 ± 323 | 8875 ± 192 | 6472 ± 103 | 0.108 ± 0.007 | −1696 ± 236 | 0.018 ± 0.007 | 7 |
SN 1990N | + 255 | −1354 ± 274 | −1827 ± 569 | 9268 ± 272 | 6655 ± 806 | 0.158 ± 0.042 | −1354 ± 274 | 0.027 ± 0.013 | 7 |
SN 1990N | + 280 | −1315 ± 229 | −1968 ± 418 | 9017 ± 114 | 8165 ± 358 | 0.191 ± 0.018 | −1315 ± 229 | 0.033 ± 0.014 | 7 |
SN 1998bu | + 236 | −1322 ± 227 | −1604 ± 272 | 8198 ± 68 | 5533 ± 319 | 0.471 ± 0.020 | −1322 ± 227 | 0.078 ± 0.031 | 8 |
SN 1998bu | + 280 | −1429 ± 265 | −1686 ± 289 | 8393 ± 437 | 4824 ± 303 | 0.353 ± 0.009 | −1429 ± 265 | 0.061 ± 0.024 | 8 |
SN 1999aa | + 260 | 114 ± 263 | −209 ± 277 | 8030 ± 100 | 3392 ± 211 | 0.138 ± 0.009 | 114 ± 263 | 0.023 ± 0.009 | 8 |
SN 2002bo | + 312 | 1251 ± 278 | 1859 ± 324 | 7505 ± 579 | 8452 ± 668 | 1.105 ± 0.143 | 1251 ± 278 | 0.194 ± 0.081 | 9 |
SN 2002dj | + 275 | 1224 ± 276 | 2202 ± 334 | 9535 ± 210 | 5445 ± 208 | 0.222 ± 0.015 | 1224 ± 276 | 0.038 ± 0.015 | 10 |
SN 2003du | + 221 | −2001 ± 292 | −3024 ± 741 | 8112 ± 355 | 8075 ± 803 | 0.323 ± 0.085 | −2001 ± 292 | 0.052 ± 0.025 | 11 |
SN 2003gsa | + 201 | 807 ± 258 | 807 ± 258 | 9687 ± 212 | 4932 ± 87 | 0.303 ± 0.021 | 807 ± 258 | 0.048 ± 0.019 | 8 |
SN 2003hv | + 320 | −2575 ± 218 | −3688 ± 257 | 8525 ± 225 | 4984 ± 107 | 0.541 ± 0.030 | −3688 ± 257 | 0.095 ± 0.038 | 12 |
SN 2003kf | + 401 | 302 ± 396 | 115 ± 728 | 10651 ± 212 | 4944 ± 1585 | 0.144 ± 0.021 | 302 ± 396 | 0.026 ± 0.011 | 9 |
SN 2004eo | + 228 | −994 ± 249 | −2266 ± 352 | 8329 ± 149 | 8556 ± 325 | 0.459 ± 0.040 | −994 ± 249 | 0.075 ± 0.031 | 13 |
SN 2005cf | + 319 | 67 ± 237 | −84 ± 1647 | 8574 ± 1106 | 6790 ± 461 | 0.208 ± 0.021 | 67 ± 237 | 0.036 ± 0.015 | 14 |
SN 2006X | + 277 | 2298 ± 277 | 2248 ± 237 | 8052 ± 114 | 5434 ± 117 | 0.472 ± 0.031 | 2298 ± 277 | 0.081 ± 0.033 | 15 |
SN 2006X | + 360 | 2731 ± 235 | 2488 ± 244 | 9182 ± 212 | 5272 ± 72 | 0.531 ± 0.024 | 2731 ± 235 | 0.095 ± 0.038 | 8 |
SN 2007af | + 303 | 421 ± 226 | 367 ± 349 | 7744 ± 367 | 5775 ± 223 | 0.265 ± 0.029 | 421 ± 226 | 0.046 ± 0.019 | 9 |
SN 2007le | + 307 | 1497 ± 246 | 1614 ± 293 | 8809 ± 287 | 5238 ± 77 | 0.255 ± 0.019 | 1497 ± 246 | 0.045 ± 0.018 | 8 |
SN 2008Q | + 201 | −2239 ± 318 | −1485 ± 228 | 11131 ± 225 | 4765 ± 29 | 0.419 ± 0.024 | −1485 ± 228 | 0.066 ± 0.027 | 8 |
SN 2011by | + 207 | −1536 ± 277 | −2845 ± 488 | 7675 ± 278 | 5547 ± 435 | 0.246 ± 0.029 | −1536 ± 277 | 0.039 ± 0.016 | 16 |
SN 2011by | + 311 | −1066 ± 212 | −1716 ± 243 | 7970 ± 155 | 5080 ± 40 | 0.318 ± 0.013 | −1066 ± 212 | 0.056 ± 0.022 | 16 |
SN 2011fe | + 205 | −1143 ± 210 | −2217 ± 241 | 8423 ± 78 | 5606 ± 56 | 0.222 ± 0.007 | −1143 ± 210 | 0.035 ± 0.014 | 17 |
SN 2011fe | + 226 | −1027 ± 229 | −2077 ± 308 | 8148 ± 128 | 5709 ± 145 | 0.254 ± 0.012 | −1027 ± 229 | 0.041 ± 0.017 | 18 |
SN 2011fe | + 311 | −1068 ± 251 | −1077 ± 332 | 8329 ± 215 | 7072 ± 224 | 0.395 ± 0.022 | −1068 ± 251 | 0.069 ± 0.028 | 18 |
SN 2011fe | + 348 | −638 ± 251 | −1297 ± 284 | 8507 ± 107 | 6922 ± 126 | 0.422 ± 0.014 | −638 ± 251 | 0.075 ± 0.030 | 19 |
SN 2011fe | + 380 | −340 ± 297 | −1047 ± 356 | 9051 ± 291 | 6980 ± 226 | 0.397 ± 0.038 | −340 ± 297 | 0.071 ± 0.029 | 18 |
SN 2012cg | + 279 | −1070 ± 223 | −1830 ± 354 | 7813 ± 164 | 5603 ± 94 | 0.187 ± 0.005 | −1070 ± 223 | 0.032 ± 0.013 | 20 |
SN 2012cg | + 339 | −1111 ± 234 | −841 ± 314 | 8716 ± 101 | 7279 ± 141 | 0.267 ± 0.010 | −1111 ± 234 | 0.047 ± 0.019 | 21 |
SN 2012fr | + 222 | 2041 ± 264 | 2926 ± 397 | 8033 ± 93 | 5650 ± 155 | 0.145 ± 0.007 | 2041 ± 264 | 0.023 ± 0.009 | 22 |
SN 2012fr | + 261 | 2030 ± 219 | 2305 ± 793 | 7338 ± 785 | 5583 ± 308 | 0.185 ± 0.007 | 2030 ± 219 | 0.031 ± 0.013 | 22 |
SN 2012fr | + 357 | 2205 ± 226 | 2633 ± 226 | 8059 ± 52 | 5393 ± 55 | 0.208 ± 0.006 | 2205 ± 226 | 0.037 ± 0.015 | 21 |
SN 2012fr | + 367 | 2085 ± 248 | 3303 ± 235 | 8799 ± 267 | 4567 ± 40 | 0.234 ± 0.014 | 2085 ± 248 | 0.042 ± 0.017 | 22 |
SN 2012hr | + 282 | 133 ± 241 | 72 ± 641 | 8342 ± 349 | 7553 ± 863 | 0.168 ± 0.041 | 133 ± 241 | 0.029 ± 0.014 | 23 |
SN 2013aa | + 400 | −691 ± 229 | −1072 ± 260 | 8237 ± 113 | 6666 ± 171 | 0.268 ± 0.012 | −691 ± 229 | 0.048 ± 0.020 | 23 |
SN 2013cs | + 262 | 1474 ± 234 | 2622 ± 246 | 9232 ± 68 | 4838 ± 74 | 0.147 ± 0.003 | 1474 ± 234 | 0.025 ± 0.010 | 23 |
SN 2013cs | + 304 | 1343 ± 245 | 1452 ± 327 | 8179 ± 170 | 6069 ± 140 | 0.220 ± 0.012 | 1343 ± 245 | 0.038 ± 0.015 | 21 |
SN 2013dy | + 333 | −1161 ± 222 | −1478 ± 273 | 7664 ± 97 | 6722 ± 220 | 0.224 ± 0.010 | −1161 ± 222 | 0.040 ± 0.016 | 24 |
SN 2013gy | + 276 | −367 ± 213 | −438 ± 402 | 8200 ± 186 | 7044 ± 259 | 0.322 ± 0.015 | −367 ± 213 | 0.055 ± 0.022 | 23 |
SN 2014J | + 231 | 336 ± 213 | 673 ± 252 | 8291 ± 88 | 9370 ± 229 | 0.349 ± 0.019 | 336 ± 213 | 0.057 ± 0.023 | 22 |
SN 2014J | + 265 | 465 ± 213 | 354 ± 280 | 8071 ± 116 | 7682 ± 175 | 0.273 ± 0.016 | 465 ± 213 | 0.046 ± 0.019 | 18 |
SN 2014J | + 292 | 581 ± 232 | 239 ± 356 | 7826 ± 108 | 7671 ± 136 | 0.323 ± 0.012 | 581 ± 232 | 0.056 ± 0.022 | 18 |
SN 2015F | + 280 | −545 ± 303 | −1233 ± 254 | 7269 ± 154 | 9694 ± 799 | 0.857 ± 0.136 | −545 ± 303 | 0.147 ± 0.063 | 23 |
SN 2017cbvb | + 318 | −1279 ± 294 | −1279 ± 294 | 7826 ± 293 | 10293 ± 958 | 0.421 ± 0.072 | −1279 ± 294 | 0.074 ± 0.032 | 25 |
SN 2017fgc | + 384 | 2026 ± 319 | 2112 ± 444 | 8603 ± 798 | 6175 ± 642 | 0.358 ± 0.081 | 2026 ± 319 | 0.064 ± 0.030 | 26 |
SN 2018oh | + 268 | −1852 ± 376 | −3934 ± 813 | 7017 ± 344 | 5299 ± 662 | 0.219 ± 0.048 | −1852 ± 376 | 0.037 ± 0.017 | 25 |
ASASSN-14jg | + 221 | 1326 ± 274 | 1113 ± 283 | 8065 ± 78 | 3666 ± 411 | 0.068 ± 0.010 | 1326 ± 274 | 0.011 ± 0.005 | 27 |
ASASSN-14jg | + 267 | 1612 ± 244 | 984 ± 560 | 7662 ± 62 | 5537 ± 437 | 0.111 ± 0.005 | 1612 ± 244 | 0.019 ± 0.008 | 23 |
ASASSN-14jg | + 323 | 1674 ± 261 | 2236 ± 596 | 8406 ± 778 | 4992 ± 552 | 0.159 ± 0.021 | 1674 ± 261 | 0.028 ± 0.012 | 21 |
Notes. References: (1) Xi et al. (2022); (2) Sai et al. (2022); (3) Iskandar et al. (in preparation); (4) Zeng et al. (in preparation); (5) Liu et al. (in preparation); (6) Cristiani et al. (1992); (7) Gómez & López (1998); (8) Silverman et al. (2012a); (9) Blondin et al. (2012); (10) Pignata et al. (2008); (11) Stanishev et al. (2007); (12) Leloudas et al. (2009); (13) Pastorello et al. (2007); (14) Wang et al. (2009a); (15) Wang et al. (2008); (16) Silverman et al. (2013); (17) Mazzali et al. (2015); (18) Stahl et al. (2020); (19) Graham et al. (2015); (20) Amanullah et al. (2015); (21) Maguire et al. (2018); (22) Childress et al. (2015); (23) Graham et al. (2017); (24) Pan et al. (2015b); (25) Tucker, Shappee & Wisniewski (2019); (26) Zeng et al. (2021); and (27) Tucker et al. (2020). The sample from our own project is put at the top of the table and separated from the public sample by a line.
aThe velocity shifts of iron and nickel features are set to the same values, and [Ca ii] emission lines are added to the fits.
bThe velocity shifts of iron and nickel features are set to the same values.
Author notes
Wood Specialist in Astronomy
Bengier-Winslow-Robertson Postdoctoral Fellow.
Eustace Specialist in Astronomy.