-
PDF
- Split View
-
Views
-
Cite
Cite
Andrew E Bryan, Maksym Krutko, Jennifer Westphal, Maulee Sheth, Leyla Esfandiari, Greg M Harris, Ultrasound-Activated Piezoelectric Polyvinylidene Fluoride–Trifluoroethylene Scaffolds for Tissue Engineering Applications, Military Medicine, Volume 188, Issue Supplement_6, November/December 2023, Pages 61–66, https://doi.org/10.1093/milmed/usad018
- Share Icon Share
ABSTRACT
Severe peripheral nervous system (PNS) injuries have limited options for therapeutic solutions to regain functional recovery. This can be attributed in part to the lack of regeneration pathways promoted by recapitulating chemical, physical, and electrical cues to direct nerve guidance. To address this, we examined ultrasonic stimulation of a piezoelectric polyvinylidene fluoride–triflouroethylene (PVDF-TrFE) scaffold as a potentially clinically relevant therapy for PNS regeneration. Owing to the piezoelectric modality of PVDF-TrFE, we hypothesize that ultrasound stimulation will activate the scaffold to electrically stimulate cells in response to the mechanical deformation mediated by sound waves. Biocompatible PVDF-TrFE scaffolds were fabricated to be used as an ultrasound-activated, piezoelectric biomaterial to enhance cellular activity for PNS applications. NIH-3T3 fibroblasts were cultured on PVDF-TrFE nanofibers and stimulated with low-, medium-, or high-powered ultrasound. 3-(4,5-dimethylthiazol-2-yl)-2,5-diphenyl-2H-tetrazolium bromide (MTT) assays were performed on fibroblasts to measure the metabolic activity of the cells following stimulation. MTT assays showed that ultrasound-stimulated fibroblasts on PVDF-TrFE scaffolds had increased metabolic activity as power was increased, whereas on plain polystyrene, an opposite trend was observed where cells had a decreased metabolic activity with ascending levels of ultrasound power. Ultrasound-stimulated PVDF-TrFE nanofibers hold exciting potential as a therapy for PNS injuries by promoting increased metabolic activity and proliferation. The ability to noninvasively stimulate implantable piezoelectric nanofibers to promote mechanical and electrical stimulation for nerve repair offers a promising benefit to severe trauma patients.
INTRODUCTION
Peripheral nervous system (PNS) injury results from damage because of traumatic stretching, severing, or compressing of the peripheral nerve. Often causing discomfort, numbness, weakness, and pain, PNS injuries occur in approximately 3% of trauma patients and vary in severity and complexity.1,2 Peripheral injuries are also a leading cause of disability and the most common nerve-related combat injury within the military health system population.3,4 Despite many recent studies on the regeneration of damaged peripheral nerves, reliable therapeutics hoping to promote full functional recovery are still deficient. Because of this constraint, those suffering often experience neuropathic pain and loss of sensory and motor function after injury. As such, new advanced therapies are needed to expand resources for the regeneration of nerve injuries. This work seeks to address the lack of appropriate signaling to the nerve by developing a polyvinylidene fluoride–trifluoroethylene (PVDF-TrFE) piezoelectric scaffold that can electrically stimulate cells on demand via therapeutic, noninvasive ultrasonic waves1,5–8
Peripheral nerve fibroblasts play a crucial role in regulating neuronal development and regeneration. Fibroblasts secrete structural extracellular matrix (ECM) proteins and factors such as neuregulin-1β1 and tenascin-C that promote Schwann cell migration and enhance plasticity that can ultimately promote functional recovery.9 Fibroblasts that release these factors can assist in de-differentiating Schwann cells into a repair phenotype, which initiates and aids peripheral nerve regeneration.9 Toward this, fibroblasts play a role in the directional Schwann cell migration that helps axon regeneration across the wound gap to bridge the transection of the nerve.10 It has also been shown that conditioned media from epineural fibroblasts increase Schwann cell migration and neurite outreach of sensory neurons. Likewise, ECM stiffness, controlled in part by ECM assembly of fibroblasts, regulates Schwann cell behaviors such as migration, proliferation, and expression of different regenerative protein markers.11–13 Therefore, this study seeks to evaluate cell–biomaterial interactions to observe the effect of ultrasound-mediated electric signaling from PVDF-TrFE.
Many modern therapeutics involving electrical stimuli require invasive, percutaneous devices or electrodes, which also find themselves lacking spatial resolution and efficacy.14 In this study, we utilize piezoelectric PVDF-TrFE scaffolds that can be activated by noninvasive mechanical stimuli to produce an electrical response capable of penetrating tissue without the need for electrodes or wires. These electrical responses can be transmitted, on demand, to target areas with high spatial resolution and precision, using an external mechanical activator such as an ultrasound transducer.1,15 Using a transducer, scaffolds can be stimulated by the mechanical deformation provided by the ultrasound waves. Therefore, the behavior of stimulated fibroblast cells and the electric potential provided by the scaffold can be exploited. Wireless mechanical stimulation of piezoelectric materials can overcome shortcomings that current stimulation methods face, as these materials can be activated by external means in a controlled, localized, and noninvasive manner.14,15 Ultrasound waves are commonly used in clinical settings for their safe and noninvasive medical applications in imaging, but they also possess utility where mechanical energy can be harnessed for various implementations. In clinical applications, ultrasound-mediated vibrations are administered through different power settings, most commonly as low-intensity pulsed ultrasound (LIPUS). High-intensity ultrasound has been previously used to precisely destroy malignant or benign tumors where the heat produced causes tissue damage to the area. However, this thermal effect is minimal in LIPUS, making this modality more suitable for modulating and stimulating scaffolds.15 Recent work shows that LIPUS can improve soft tissue regeneration, enhance neuronal activity by regulating the secretion of cytokines and neurotrophic factors, and decrease inflammatory responses.16–18 Additionally, several studies have shown that the application of LIPUS-based therapies on nerve injury has positive effects on promyelinating genes as well as regulating cytokines and neurotrophic factors present during PNS regeneration.19 Yet, even with many promising benefits of LIPUS on PNS repair, the comprehensive effectiveness of ultrasound in neurodegenerative conditions or trauma remains complex and understudied. Because of these factors, the application of LIPUS in nerve repair is still scarce.
In this study, we explore the biocompatibility and metabolic response of NIH-3T3 fibroblasts when seeded on polystyrene and PVDF-TrFE scaffolds subjected to mechanical and electrical stimulation (Fig. 1). The dual stimulation is mediated by LIPUS and ultrasonic intensities slightly greater than traditional LIPUS ranges. It is shown that PVDF-TrFE scaffolds can utilize the noninvasive LIPUS to enhance metabolic activity in fibroblasts compared to polystyrene.
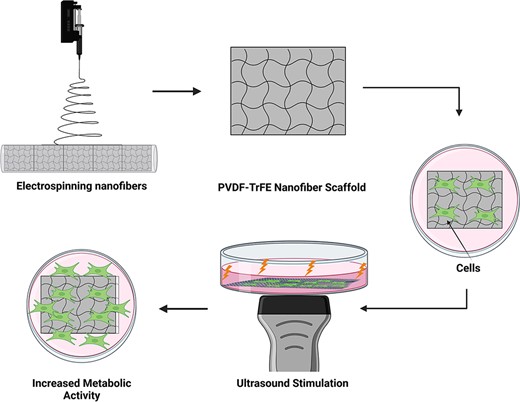
A schematic detailing experimental approach. Stimulating cells cultured on polyvinylidene fluoride–triflouroethylene scaffolds through ultrasonic waves improves cell metabolic activity, thus facilitating nerve regeneration. Scaffolds used in the experiment formed from electrospun nanofibers consisting of a 20% polyvinylidene fluoride–triflouroethylene solution spun for 3 hours. Ultrasound stimulation applied directly beneath the scaffold from under the tissue culture plate provided enough power to mechanically deform the scaffold, instigating a mechanoelectrical response from the piezoelectric fibers.
MATERIALS AND METHODS
Preparation of Nanofibers
PVDF-TrFE nanofibers were prepared (Fig. 1) as described by our previous work.7,8 Briefly, a polymer solution consisting of 20% PVDF-TrFE (70/30) (PolyK Technologies, State College, PA) and solvent of N,N-dimethylformamide and acetone (6/4 v/v) was added to a 5-mL syringe fitted with a 20-gauge needle. The syringe pump was set to supply a flow rate of 1 mL h−1. The needle tip was positioned 10 cm away from the collector which rotated at 2,000 RPM to produce aligned fibers wrapped on a conductive polymer liner (McMaster-Carr). An 18-kV voltage was applied between the needle tip and the collector.
Scaffold Characterization
Fiber morphology was analyzed using scanning electron microscopy (SEM) (Apreo C SEM, Thermo Fisher). Two samples per experimental condition were prepared by sputter coating a layer of gold for approximately 10 seconds. An acceleration voltage of 5 kV was used with a working distance of 5 mm. For cell culture experiments, PVDF-TrFE nanofiber sheets were cut into 5 cm × 5 cm square segments.
Cell Culture
NIH-3T3 fibroblasts (ATCC) were cultured in HyClone Dulbecco’s high glucose modified eagle’s medium (Cytiva) supplemented with 1% Pen/Strep and 10% bovine calf serum. Cultures were incubated at 37 °C at 5% CO2 and 95% relative humidity and routinely passaged upon reaching sub-confluence. Square PVDF-TrFE scaffold segments were attached to the bottom of 60 mm × 15 mm cell culture plates (Falcon). Scaffold corners were attached to the bottom of plates using quick-hardening mounting medium (Sigma-Aldrich) to ensure submersion within media and prevent free-flowing movement during ultrasonic stimulation. Upon attachment, scaffolds were pretreated for cell culture with a 70% ethanol rinse followed by three subsequent rinses with PBS. Additionally, scaffolds were subjected to UV-light exposure (Novascan, Milwaukee, WI) for 120 minutes followed by an additional PBS rinse. Fibroblasts were seeded at 50 cells mm−2 and examined 24 hours after stimulation to assess viability.
SEM and Immunofluorescence Microscopy
For SEM imaging, PVDF-TrFE scaffolds were seeded with NIH-3T3 fibroblasts at 1,500 cell mm−2 and allowed to adhere and proliferate. Cell-seeded scaffolds were subsequently treated with a glutaraldehyde solution and dried with serial dilutions of ethanol as described in previous work.7 Three samples were prepared by sputter coating a layer of gold for approximately 10 seconds. An acceleration voltage of 5 kV was used with a working distance of 5 mm for imaging.
Rhodamine phalloidin (Invitrogen) and 4ʹ,6ʹ-diamidino-2-phenylindole dihydrochloride (DAPI) (Abcam) were used to observe cell morphology (Phalloidin) and visualize cell nuclei (DAPI), respectively.
Scaffolds were washed thrice in PBS before being fixed in 3.7% formaldehyde for 15 minutes. Following two additional PBS washes, samples were permeabilized with 0.1% Triton X-100 (Fisher) at 4 °C for 5 minutes followed with an additional PBS wash. Samples were incubated in a 1:100 dilution of rhodamine phalloidin (10 µg ml−1) at 37 °C for 30 minutes followed by three PBS washes. Scaffolds were then incubated in 300 nM DAPI antibody and mounted on glass microscope slides with antifade mounting medium (Abcam). Samples were sealed onto microscope slides using clear nail polish.
Wide-field microscopy images were captured using a Nikon Eclipse Ti2 inverted microscope in tandem with a Nikon DS-Qi2 camera using both fluorescent and phase microscopy.
Ultrasound Stimulation
Cells were stimulated with an ultrasound device (US PRO 2000 2nd Edition Model No. DU3035) from beneath the polystyrene cell culture plates with and without PVDF-TrFE scaffolds, after transmission gel was applied. The ultrasound device was equipped with three different power settings including low (0.08 W cm−2 ± 20%), medium (0.80 W cm−2 ± 20%), and high (1.60 W cm−2 ± 20%). Each power setting was applied at a frequency of 1.0 MHz ± 10% for 3 minutes, 24 hours after cells were seeded to allow for sufficient adhesion.
MTT Colorimetric Assay
MilliporeSigma 3-(4,5-dimethylthiazol-2-yl)-2,5-diphenyl-2H-tetrazolium bromide (MTT), Calbiochem (Fisher Scientific) was used to perform a colorimetric measurement of metabolic activity, as previously described.20 NIH-3T3 fibroblast cells were used as a standard to create a linear absorbance curve for the colorimetric reduction of MTT by metabolic enzyme carriers, where the optical density detected by the microplate reader (Biorad) at 540 nm (OD540nm) serves as an indicative measure of cell proliferation. Cells were seeded at 50 cells mm−2 on either PVDF-TrFE scaffolds or polystyrene cell culture plates and examined for metabolic activity after 48 hours. Briefly, at the 48-hour time point, cell cultures were aspirated and replaced with fresh media supplemented with a 12-mM MTT solution. Cultures were incubated for 30 minutes before the addition of dimethyl sulfoxide to solubilize the reduced MTT (formazan) following a second incubation period of 10 minutes. Contents were evenly mixed and distributed to a 96-well plate to measure relative absorbance.
Statistical Analysis
Data were reported as mean ± SD. Statistical analyses were performed using Microsoft Excel and GraphPad PRISM (v. 9.4.1) to analyze fiber diameter and metabolic activity. One-way and two-way ANOVA and Brown Forsythe, Welch, and Šídák’s multiple comparison tests were used to find statistical significance between groups.
RESULTS AND DISCUSSION
Development of Ultrasound-Mediated PVDF-TrFE Scaffolds
PVDF-TrFE scaffolds have multiple tunable characteristics, such as porosity, alignment of fibers, elastic modulus, and piezoelectric capacity that lend themselves to be desirable for PNS therapeutics. Our group has previously characterized a collection of electrospinning properties to develop PVDF-TrFE scaffolds for tissue engineering. Specifically, mechanically stimulated PVDF-TrFE scaffolds generate an electrical response of 22 nanoamps of current and up to an approximate voltage of 1.5 V.13,21 Scaffolds with aligned fibers spun for 3 hours also display the enhanced piezoelectric response that can lead to on-demand cellular signaling and be beneficial for nerve regeneration (Fig. 2A).7 As such, this study uses 3-hour electrospun nanofibrous PVDF-TrFE scaffolds to qualitatively demonstrate their ability, with ultrasonic stimulation, to allow favorable cell adhesion and proliferation. Integration of fibroblasts into PVDF-TrFE scaffolds is visualized using SEM imaging (Fig. 2B). Biocompatibility of fibroblast cells cultured on PVDF-TrFE scaffolds is shown through immunofluorescent microscopy (Fig. 2C).
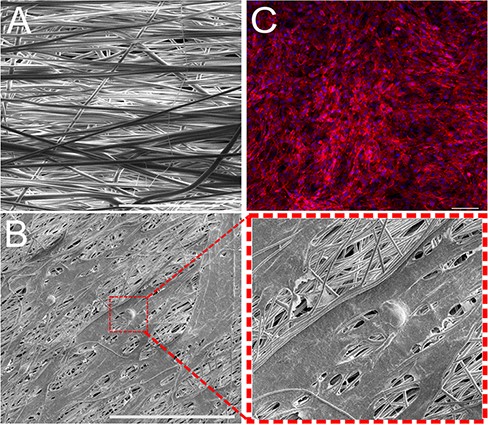
(A) Scanning electron microscopy image of the polyvinylidene fluoride–triflouroethylene (PVDF-TrFE) scaffold consisting of 20% copolymer solution electrospun for 3 hours. Image taken at 1,000× magnification (scale bar = 20 μm). (B) Scanning electron microscopy image of fibroblast cells cultured on electrospun, PVDF-TrFE scaffold. Image taken at 1,000× magnification (scale bar = 100 μm). (C) Fibroblasts integrate with PVDF-TrFE to orient themselves in the direction of nanofiber alignment (scale bar = 100 μm). Cell nuclei stained with 4ʹ,6ʹ-diamidino-2-phenylindole dihydrochloride (blue) and cytoskeleton stained with rhodamine phalloidin (red).
Ultrasonic Stimulation of Fibroblasts
Metabolic activity has previously been shown to be indicative of cell function.22 The application of ultrasound at varying levels resulted in a different metabolic activity in fibroblasts for polystyrene and piezoelectric PVDF-TrFE nanofiber scaffold conditions. In the polystyrene group (Fig. 3A), a significant increase in activity is seen under low-powered ultrasound conditions (0.08 W cm−2). Upon increasing the power settings, a significant decrease is seen in metabolic activity under medium-powered (0.8 W cm−2) and high-powered (1.60 W cm−2) treatment compared to treatments under low intensity. This is further validated by a significant decrease in metabolic activity between the non-treated group and the high-powered ultrasound-treated group. These results can be attributed to LIPUS or low-intensity powered ultrasound being beneficial in activating specific cell functions and signaling.18–20 Higher-powered ultrasound tends to produce heat-triggering apoptotic cascades within cells because of the production of heat shock proteins, which is often utilized in cancer therapy treatments.21–23 In this study, the medium- and high-powered settings on the ultrasound device lie on the edge and outside the range of traditional LIPUS parameters, respectively.23 These intensity values appeared to negatively affect fibroblast proliferation when cultured on polystyrene. However, ultrasound therapies are based on various parameters; thus, intensity should not be the only measurement on which treatments are based. When cells on PVDF-TrFE scaffolds were stimulated, a different trend was seen. A steady increase in mean relative absorbance was observed across all increases in ultrasound-power levels (Fig. 3B). Additionally, we observed a strong increase in relative absorbance between no ultrasound treatment and medium-powered intensity and a strong significant increase between no treatment and high-powered treatment. When the different groups, polystyrene and PVDF-TrFE, are cross-compared at the same level of ultrasound-powered treatments, a significant increase in activity between each group is seen (Fig. 3C). It is of note that a smaller difference in means of relative absorbance is seen in the low-powered ultrasound treatment group.
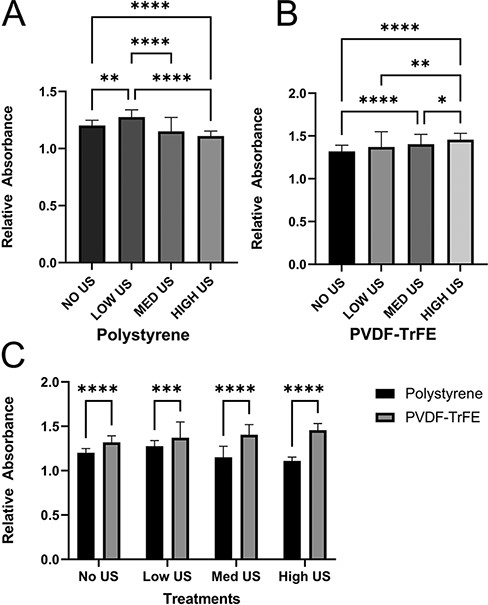
3-(4,5-dimethylthiazol-2-yl)-2,5-diphenyl-2H-tetrazolium bromide (MTT) absorbance quantification of fibroblasts 24 hours after being subject to various intensities of ultrasonic stimulation (48 hours after cell seeding). (A) Relative absorbance measured with cells seeded on polystyrene. (B) Relative absorbance measured with cells seeded on polyvinylidene fluoride–triflouroethylene scaffolds. Significant differences among all intensity levels. (C) Direct comparison between polystyrene measurements and scaffold measurements. Polyvinylidene fluoride–triflouroethylene scaffolds improve proliferative counts across all intensity values. Data are reported as mean ± SD. *P ≤ .05; **P ≤ .01; ***P ≤ .001; ****P ≤ .0001.
The mechanism by which ultrasound improves the metabolic activity of fibroblasts may be dyadic in nature. Ultrasonic waves provide mechanical deformation, and by using piezoelectric nanofibers, electrical stimulation is also present. Numerous studies have detailed the importance of mechanical stress in cellular microenvironments.24–26 Studies subjecting cells to mechanical stimulation using a variety of methods have detailed their benefits to proliferation, consistent with the results presented here.27,28 Further evidence suggests that mechanical stress leads to a greater production of ECM proteins and proteoglycan content.29 Coinciding with our results, increasing fibroblast density would also result in greater ECM production because of the availability of more cells to secrete the primary ECM components.29 Possessing diverse responsibilities, the ECM regulates multiple cellular functions including survival, growth, proliferation, migration, and differentiation. Many of these factors become important downstream in the nerve healing process after a new ECM has formed at the injury site.30 Schwann cells, for instance, require their phenotype to be altered from their myelinating phase to a more regenerative state.13 Increasing fibroblast density at the wound site could, therefore, be important to improving the neuroregenerative process.
In addition to mechanical stimuli, it has been shown that electrical stimulation is effective in accelerating wound healing.31–33 Gordon et al. showed that axon outgrowth across injury sites can be improved through brief, low-frequency electrical stimulation by enhancing neuronal cell activity.34,35 Hoop et al. demonstrated the ability of ultrasound to instigate the mechanoelectrical response of PVDF membranes, thus activating calcium ion channels that lead to neurite regeneration.36 The implications of these reports and our findings suggest that the presence of mechanical and electrical activities is fundamental for creating a microenvironment in which to promote nerve regeneration.
CONCLUSION
In this study, we have shown the potential for ultrasonic therapy to positively impact the cellular microenvironment, specifically regarding nerve regeneration. Here, a piezoelectric PVDF-TrFE scaffold was created by electrospinning to possess properties capable of being utilized in conjunction with ultrasonic therapies to facilitate nerve repair. Non-invasive, ultrasonic waves stimulated a PVDF-TrFE scaffold to provide mechanical and electrical cues to fibroblasts on the scaffold. Stimulation of the scaffold was shown to enhance metabolic activity in the cells in comparison to controls. This approach to utilize a noninvasive, ultrasonic technique along with piezoelectric scaffolds provides great insight into the development of a functional tissue engineering platform that can improve current outcomes for PNS repair. Furthermore, PVDF-TrFE scaffolds are in development to be fabricated into conduits and implanted in vivo for the repair of sciatic or median nerve PNS injuries. Additional studies are required to evaluate the foreign body response of implantable scaffolds as well as therapeutic metrics of LIPUS stimulation in vivo.
SUPPLEMENT SPONSORSHIP
This article appears as part of the supplement “Proceedings of the 2022 Military Health System Research Symposium,” sponsored by the Assistant Secretary of Defense for Health Affairs.
ACKNOWLEDGMENTS
The authors would like to thank Dr. Melodie Fickenscher for assistance with SEM imaging.
FUNDING
The authors gratefully acknowledge support from the DoD (DM190692 to G.M.H. and L.E.), the National Science Foundation (DMR-2104639 to G.M.H. and L.E.), and the University of Cincinnati (G.M.H. and L.E.).
CONFLICT OF INTEREST STATEMENT
None declared.
DATA AVAILABILITY
The raw data for this study were generated at the University of Cincinnati. Derived data supporting the findings of this study are available upon reasonable request from the corresponding authors.
REFERENCES
Author notes
The authors contributed equally to work.
This study was presented as an oral presentation at the 2022 Military Health System Research Symposium, Kissimmee, FL; MHSRS-22-05148.
The views expressed are solely those of the authors and do not reflect the official policy or position of the U.S. Army, U.S. Navy, U.S. Air Force, the DoD, or the U.S. Government.