-
PDF
- Split View
-
Views
-
Cite
Cite
Hao-Kun Xu, Jie-Xi Liu, Ze-Kai Zhou, Chen-Xi Zheng, Bing-Dong Sui, Yuan Yuan, Liang Kong, Yan Jin, Ji Chen, Osteoporosis under psychological stress: mechanisms and therapeutics, Life Medicine, Volume 3, Issue 1, February 2024, lnae009, https://doi.org/10.1093/lifemedi/lnae009
- Share Icon Share
Abstract
Psychological stress has been associated with the onset of several diseases, including osteoporosis. However, the underlying pathogenic mechanism remains unknown, and effective therapeutic strategies are still unavailable. Growing evidence suggests that the sympathetic nervous system regulates bone homeostasis and vascular function under psychological stress, as well as the coupling of osteogenesis and angiogenesis in bone development, remodeling, and regeneration. Furthermore, extracellular vesicles (EVs), particularly mesenchymal stem cell extracellular vesicles (MSC–EVs), have emerged as prospecting therapies for stimulating angiogenesis and bone regeneration. We summarize the role of sympathetic regulation in bone homeostasis and vascular function in response to psychological stress and emphasize the relationship between vessels and bone. Finally, we suggest using MSC–EVs as a promising therapeutic method for treating osteoporosis in psychological stress.
Introduction
Psychological stress is a state of tension characterized by physiological, emotional, and behavioral changes due to adversity, perceived stress, or threat [1–3]. People are experiencing significantly increased psychological pressure and stress caused by negative life events, which seriously impact physical health as the pace of life and work continues to accelerate [4]. Under normal circumstances, the stress response system has robust basic activity and response capabilities, critical for happiness, task achievement, and appropriate social interaction. However, the excessive or insufficient activity of this system impairs development and growth, resulting in various behavioral changes and pathological conditions [5]. Increasing evidence suggests that chronic psychological stress affects brain function and disrupts the balance of the stress system, resulting in inflammatory diseases, cardiovascular diseases, obesity, diabetes, atherosclerosis, cancer, neurodegenerative diseases, and osteoporosis [6–12].
Osteoporosis is a systemic skeletal disease that causes reduced bone mass and deterioration of bone tissue microstructure, increasing the risk of fractures [13]. Osteoporosis affected over 12.6% of non-institutionalized Americans aged 50 and over in 2017–2018 [14]. In 2017, 20 million people aged 50 or older in Europe had osteoporosis, with 2.7 million suffering osteoporotic fractures, resulting in a total treatment cost of 37.5 million euros and a considerable economic burden on society [15]. An increasing body of evidence indicates a substantial correlation between chronic psychological stress and bone loss [16–18], with studies revealing a positive relationship between the risk of osteoporotic fractures and psychological stress [19]. Furthermore, individuals diagnosed with post-traumatic stress disorder (PTSD) are more likely to develop osteoporosis [20], and long-term chronic psychological stress leads to depression, which is related to reduced bone mineral density (BMD) compared to non-depressed individuals [21].
Although earlier research has suggested a tight relationship between osteoporosis and psychological stress, the underlying mechanism and effective treatment techniques and approaches for osteoporosis under chronic psychological stress remain unknown and unestablished. We summarize the correlation between psychological stress and osteoporosis and highlight the function of the sympathetic nervous system (SNS) in regulating bone homeostasis. We also discuss the role of vasculature in bone development, remodeling, and regeneration, along with the effects of SNS on vascular regulation in bone. Furthermore, we focus on emerging therapeutics of extracellular vesicles (EVs), particularly mesenchymal stem cell-derived EV (MSC–EVs). We detail the impacts and mechanisms underpinning the use of MSC–EVs in vascular regulation and bone regeneration and propose the MSC–EV engineering strategy to enhance their efficiency and achieve more precise targeting.
Psychological stress and osteoporosis
Response mechanisms and sympathetic nervous system effects under psychological stress
The neuroendocrine response to stress causes numerous metabolic and functional changes in multiple organs and cells to maintain individual homeostasis in the face of possible dangers. The hypothalamus-pituitary-adrenal (HPA) axis and the sympathetic-adrenal medullary (SAM) axis are the two most important neuroendocrine response axes [22]. The HPA axis is activated when the hypothalamus secretes corticotropin-releasing hormone (CRH), stimulating adrenocorticotropic hormone (ACTH) release from the pituitary. As a result, glucocorticoid (GC) production and secretion from the adrenal cortex are regulated. GCs play crucial roles in reacting to harmful stimuli, maintaining blood pressure and blood sugar levels, mobilizing lipids, fighting cell damage, and regulating inflammatory reactions. Sympathetic activation increases, and norepinephrine (NE) is released from sympathetic nerve terminals when SAM axis is activated (Fig. 1). This activates the adrenal medulla to rapidly generate large amounts of catecholamines, mobilizing and regulating respiration, metabolism, and blood circulation, resulting in a state of alertness in the body and ensuring that critical organs can meet stress demands [23–26].
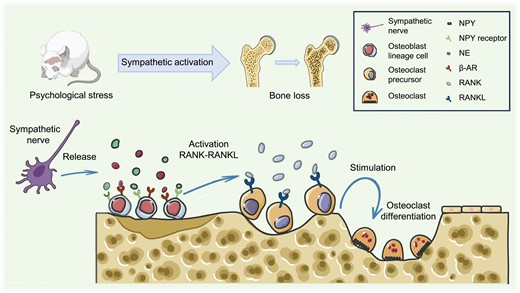
Psychological stress-induced sympathoexcitation leads to bone loss.Activation of sympathetic nerves triggers the release of NE and NPY, which bind to osteoblast β-AR and NPY receptors, initiating the RANK-RANKL pathway and driving osteoclast differentiation, ultimately resulting in bone loss. NE, norepinephrine; β-AR, beta-adrenergic receptor; NPY, neuropeptide Y.
The SNS is widely distributed, with postganglionic fibers from neurons in the paravertebral ganglia innervating the pupils, heart, respiratory system, blood vessels, facial and jaw muscles, trunk, and exocrine glands of the limbs. At the same time, postganglionic fibers from neurons in the prevertebral ganglia innervate the organs in the abdomen, pelvis, and perineum [27]. NE is the main SNS neurotransmitter, transmitting signals through adrenergic receptors (ARs), which are classified into α and β types and are both G protein-coupled receptors [28]. Dysfunction of the SNS is closely related to the onset and progression of many diseases, such as peripheral neuropathy, congestive heart failure, hypertension, diabetes, and immune dysfunction [29–33].
Regulation of bone homeostasis under psychological stress
Bones are critical organs that maintain posture and mobility, protect vital organs, and serve as crucial sites for hematopoiesis and mineral storage, in addition to playing an essential role in endocrine regulation and homeostasis [34, 35]. In the early 21st century, cross-sectional investigations on bone mass, body weight, and gonadal function have revealed new insights into how the nervous system regulates bone remodeling. Leptin was discovered in 2002 to alter SNS tone by acting on the hypothalamus, resulting in the release of NE into the local microenvironment and the activation of β-adrenergic receptors (β-ARs) produced by osteoblasts to modulate bone mass [36]. Additionally, neuropeptide U also regulates bone mass by signal processing through the SNS [37]. As research on the local microenvironment of bone progresses, the crucial regulatory role of SNS in bone metabolism has gradually emerged. The SNS is widely distributed in the periosteum and bone, densely distributed in the growth plate and metaphysis of long bones, and runs parallel to blood vessels. ARs and neuropeptide receptors have also been detected in osteoblasts and osteoclasts [38–40]. These findings imply that the SNS regulates the role of bone tissue.
Regulation of bone homeostasis is critical for adapting to changes in the physiology and external environment while maintaining bone morphology and function. This is mostly accomplished through bone remodeling, which involves the absorption of existing bone and the production of new bone, as conducted by osteoclasts and osteoblasts, respectively (Fig. 1). The balance between osteoblast and osteoclast activity maintains normal bone homeostasis [41]. The process of bone remodeling is mediated by the κ-β nuclear receptor (RANK)/RANK ligand (RANKL)/osteoprotegerin (OPG) axis. RANKL released by cells in the osteoblast lineage binds to its receptor RANK on precursor cells of osteoclasts. Then, RANK-RANKL binding drives precursor differentiation of precursor cells into osteoclasts, which merge to produce mature multinucleated osteoclasts.
Furthermore, osteoblasts release OPG, which functions as a decoy receptor for RANKL and inhibits RANK-RANKL interaction, reducing osteoclastogenesis [42]. Psychological stress activates the SNS. NE produced by nerve fibers activates β-ARs on osteoblasts, causing them to create interleukin 6 (IL-6) and interleukin 11 (IL-11), inhibiting bone formation. It also induces osteoblast lineage cells to produce RANKL, promoting osteoclast differentiation [43–45]. Neuropeptide Y (NPY), frequently generated with NE, negatively regulates bone development and contributes to bone loss [46, 47].
In addition to directly regulating bone homeostasis through neurotransmitter release, the SNS also exerts indirect control on bone via paracrine mechanisms involving EVs and cytokines. Sui et al. demonstrated that the application of isoproterenol (ISO), a beta-adrenergic receptor agonist, induced osteoblasts to transport EVs containing miR-21 to osteoclasts [48]. Subsequently, miR-21 played a crucial role in modulating osteoclastogenesis in vivo by inhibiting programmed cell death 4 (Pdcd4), resulting in bone loss, not through a direct reduction in osteoblast activity [49]. Elefteriou et al. revealed that sympathetic activation induced by leptin triggers bone resorption by upregulating the expression of the osteoclast differentiation factor RANKL in osteoblast progenitor cells [50]. This process is mediated through phosphorylated activating transcription factor 4 (ATF4), which plays an essential role in osteoblast differentiation and function, rather than via direct regulation of osteoclasts. Considering the indirect regulatory role of the SNS in bone homeostasis, various therapeutic strategies have been proposed. For instance, targeted delivery approaches specifically designed to silence osteoblastic miR-21 or employing (D-Asp8)-lipid nanoparticle-mediated targeted inhibition of osteoclastic miR-21, as well as clinically relevant drugs, show promise in restoring the balance of bone remodeling and alleviating ISO- and CVS-induced osteoporosis [48, 49]. Additionally, considering the significance of ADRB2, an effective, bone-specific pharmacological blockade targeting ADRB2 signaling would be highly beneficial in mitigating bone loss induced by sympathetic activation.
Models of psychological stress and osteoporosis
Since the groundbreaking research by Holmes and Rahe in 1967, exploring the impact of life events on health, there has been a focused effort to establish precise animal models dedicated to studying the effects of psychological stress [51]. Currently, animal research models related to psychological stress are categorized into acute stress models, chronic stress models, and drug-induced models. Acute stress models, such as acute restraint stress and single prolonged stress, are designed to simulate situations in humans where experiences trigger intense negative emotions and a sense of trauma-inducing tension. These symptoms usually develop rapidly within minutes or hours–a quick reaction [52, 53]. Conversely, chronic stress models like social defeat, chronic variable stress, and trauma witness aim to simulate chronic changes in cognitive, emotional, physical, and behavioral aspects caused by a consistent sense of feeling pressured and overwhelmed over an extended period [52, 53].
Additionally, psychological stress models can also be established by administering drugs such as ISO and corticosterone (CORT) to mimic changes in hormones during psychological stress. The widespread use of this method is attributed to its singular treatment factor and predictable model establishment outcomes. Specific establishing methods of psychological stress related models have been summarized in Table 1. Moreover, the success of model establishment can be assessed through detections such as serum biomarker analysis, sucrose preference test, feeding experiments, social interaction trials, open field tests, forced swim tests, etc [48, 49, 79–83]. Among these psychological stress models, several have been confirmed to induce osteoporosis. For instance, in the study by Sui et al., models inducing psychological stress-related bone loss were established using chronic variable stress and ISO induction methods [48, 49]. Similarly, Zhang et al. detected reduced bone mass in the psychological stress model established through chronic social defeat stress methods [67].
Models . | Methods . | Detections of sympathetic activation . | Bone loss . | Ref. . |
---|---|---|---|---|
Time-dependent sensitization (TDS) | Exposure to brief, intense stimuli followed by subsequent pharmacological and non-pharmacological stressors. | Not enumerated | Not investigated | [54] |
Electric shock (ES) | Inescapable electric foot shocks (shock duration and current depend on frequency), sometimes combined with situational reminders. | Open-field test, noise test, elevated plus-maze test | Not investigated | [55–57] |
Underwater trauma (UT) | One-minute forced swim followed by 20–45 seconds of forced submersion in a water tank. | Transfer test | Not investigated | [58, 59]. |
Restraint stress (RS) | Immobilization on wooden boards or in a restraint container (e.g. syringe, wire mesh tube, 50 mL centrifuge tube) for 1–2 hours. | Elevated plus-maze test, biomarker assays | Yes | [60–63] |
Single prolonged stress (SPS) | Two-hour restraint followed by a 20-minute forced swim and diethyl ether anesthesia until loss of consciousness. | Biomarker assays | Not investigated | [64] |
Predator-based psychosocial stress (PPS) | Exposure to a natural predator or predator-related stimuli, sometimes coupled with unstable housing conditions for 3–4 weeks. | Elevated plus-maze test, noise test, radial-arm water maze, novel object recognition, biomarker assays | Not investigated | [65, 66] |
Social defeat (SD) | Conspecific trained aggressor exposure for 6 hours daily for 5 or 10 days. | Biomarker assays, open-field test, forced swim test, sucrose preference test, tail suspension test, partition test ethogram evaluation, urine marking test | Yes | [67, 68] |
Social isolation (SI) | Social isolation (complete or near-complete lack of social interaction) during 3–4 weeks. | Training test, contextual test, cued test, extinction test | Yes | [69, 70] |
Trauma witness (TW) | Witnessing the social defeat event three times in one week. | Open-field test, elevated plus-maze test, light-dark exploration, forced swim stress, sucrose preference test, memory function test | Not investigated | [71] |
Early life stress (ELS) | Separating animal pups from mothers for several hours daily during postnatal days 1–10, followed by re-exposure to stressors (e.g. underwater trauma) in adulthood. | Biomarker assays, elevated plus-maze test, acoustic startle response test, ultrasonic vocalizations, open-field test | Yes | [72–74] |
Tail suspension (TS) | Suspension by the tail for 2–4 weeks. | Not enumerated | Yes | [75, 76] |
Chronic variable stress (CVS) | Exposure to variable stressors for 5–9 weeks, e.g. overnight illumination, changing cage mates, food deprivation and so on. | Biomarker assays, sucrose preference test | Yes | [48, 49] |
Chronic subordinate colony housing paradigm (CSC) | Housing in prolonged subordination to a dominant resident mouse for 19 consecutive days. | Biomarker assays, elevated plus-maze test | Not investigated | [77] |
Drug induction | Daily intraperitoneal injections of ISO solution (10 mg/kg) for 4 weeks. | Biomarker assays | Yes | [48, 49] |
Daily subcutaneous injections of CORT solution (20 mg/kg) for 6 weeks. | Tail suspension test, forced swimming test, open field test | Not investigated | [78] |
Models . | Methods . | Detections of sympathetic activation . | Bone loss . | Ref. . |
---|---|---|---|---|
Time-dependent sensitization (TDS) | Exposure to brief, intense stimuli followed by subsequent pharmacological and non-pharmacological stressors. | Not enumerated | Not investigated | [54] |
Electric shock (ES) | Inescapable electric foot shocks (shock duration and current depend on frequency), sometimes combined with situational reminders. | Open-field test, noise test, elevated plus-maze test | Not investigated | [55–57] |
Underwater trauma (UT) | One-minute forced swim followed by 20–45 seconds of forced submersion in a water tank. | Transfer test | Not investigated | [58, 59]. |
Restraint stress (RS) | Immobilization on wooden boards or in a restraint container (e.g. syringe, wire mesh tube, 50 mL centrifuge tube) for 1–2 hours. | Elevated plus-maze test, biomarker assays | Yes | [60–63] |
Single prolonged stress (SPS) | Two-hour restraint followed by a 20-minute forced swim and diethyl ether anesthesia until loss of consciousness. | Biomarker assays | Not investigated | [64] |
Predator-based psychosocial stress (PPS) | Exposure to a natural predator or predator-related stimuli, sometimes coupled with unstable housing conditions for 3–4 weeks. | Elevated plus-maze test, noise test, radial-arm water maze, novel object recognition, biomarker assays | Not investigated | [65, 66] |
Social defeat (SD) | Conspecific trained aggressor exposure for 6 hours daily for 5 or 10 days. | Biomarker assays, open-field test, forced swim test, sucrose preference test, tail suspension test, partition test ethogram evaluation, urine marking test | Yes | [67, 68] |
Social isolation (SI) | Social isolation (complete or near-complete lack of social interaction) during 3–4 weeks. | Training test, contextual test, cued test, extinction test | Yes | [69, 70] |
Trauma witness (TW) | Witnessing the social defeat event three times in one week. | Open-field test, elevated plus-maze test, light-dark exploration, forced swim stress, sucrose preference test, memory function test | Not investigated | [71] |
Early life stress (ELS) | Separating animal pups from mothers for several hours daily during postnatal days 1–10, followed by re-exposure to stressors (e.g. underwater trauma) in adulthood. | Biomarker assays, elevated plus-maze test, acoustic startle response test, ultrasonic vocalizations, open-field test | Yes | [72–74] |
Tail suspension (TS) | Suspension by the tail for 2–4 weeks. | Not enumerated | Yes | [75, 76] |
Chronic variable stress (CVS) | Exposure to variable stressors for 5–9 weeks, e.g. overnight illumination, changing cage mates, food deprivation and so on. | Biomarker assays, sucrose preference test | Yes | [48, 49] |
Chronic subordinate colony housing paradigm (CSC) | Housing in prolonged subordination to a dominant resident mouse for 19 consecutive days. | Biomarker assays, elevated plus-maze test | Not investigated | [77] |
Drug induction | Daily intraperitoneal injections of ISO solution (10 mg/kg) for 4 weeks. | Biomarker assays | Yes | [48, 49] |
Daily subcutaneous injections of CORT solution (20 mg/kg) for 6 weeks. | Tail suspension test, forced swimming test, open field test | Not investigated | [78] |
Models . | Methods . | Detections of sympathetic activation . | Bone loss . | Ref. . |
---|---|---|---|---|
Time-dependent sensitization (TDS) | Exposure to brief, intense stimuli followed by subsequent pharmacological and non-pharmacological stressors. | Not enumerated | Not investigated | [54] |
Electric shock (ES) | Inescapable electric foot shocks (shock duration and current depend on frequency), sometimes combined with situational reminders. | Open-field test, noise test, elevated plus-maze test | Not investigated | [55–57] |
Underwater trauma (UT) | One-minute forced swim followed by 20–45 seconds of forced submersion in a water tank. | Transfer test | Not investigated | [58, 59]. |
Restraint stress (RS) | Immobilization on wooden boards or in a restraint container (e.g. syringe, wire mesh tube, 50 mL centrifuge tube) for 1–2 hours. | Elevated plus-maze test, biomarker assays | Yes | [60–63] |
Single prolonged stress (SPS) | Two-hour restraint followed by a 20-minute forced swim and diethyl ether anesthesia until loss of consciousness. | Biomarker assays | Not investigated | [64] |
Predator-based psychosocial stress (PPS) | Exposure to a natural predator or predator-related stimuli, sometimes coupled with unstable housing conditions for 3–4 weeks. | Elevated plus-maze test, noise test, radial-arm water maze, novel object recognition, biomarker assays | Not investigated | [65, 66] |
Social defeat (SD) | Conspecific trained aggressor exposure for 6 hours daily for 5 or 10 days. | Biomarker assays, open-field test, forced swim test, sucrose preference test, tail suspension test, partition test ethogram evaluation, urine marking test | Yes | [67, 68] |
Social isolation (SI) | Social isolation (complete or near-complete lack of social interaction) during 3–4 weeks. | Training test, contextual test, cued test, extinction test | Yes | [69, 70] |
Trauma witness (TW) | Witnessing the social defeat event three times in one week. | Open-field test, elevated plus-maze test, light-dark exploration, forced swim stress, sucrose preference test, memory function test | Not investigated | [71] |
Early life stress (ELS) | Separating animal pups from mothers for several hours daily during postnatal days 1–10, followed by re-exposure to stressors (e.g. underwater trauma) in adulthood. | Biomarker assays, elevated plus-maze test, acoustic startle response test, ultrasonic vocalizations, open-field test | Yes | [72–74] |
Tail suspension (TS) | Suspension by the tail for 2–4 weeks. | Not enumerated | Yes | [75, 76] |
Chronic variable stress (CVS) | Exposure to variable stressors for 5–9 weeks, e.g. overnight illumination, changing cage mates, food deprivation and so on. | Biomarker assays, sucrose preference test | Yes | [48, 49] |
Chronic subordinate colony housing paradigm (CSC) | Housing in prolonged subordination to a dominant resident mouse for 19 consecutive days. | Biomarker assays, elevated plus-maze test | Not investigated | [77] |
Drug induction | Daily intraperitoneal injections of ISO solution (10 mg/kg) for 4 weeks. | Biomarker assays | Yes | [48, 49] |
Daily subcutaneous injections of CORT solution (20 mg/kg) for 6 weeks. | Tail suspension test, forced swimming test, open field test | Not investigated | [78] |
Models . | Methods . | Detections of sympathetic activation . | Bone loss . | Ref. . |
---|---|---|---|---|
Time-dependent sensitization (TDS) | Exposure to brief, intense stimuli followed by subsequent pharmacological and non-pharmacological stressors. | Not enumerated | Not investigated | [54] |
Electric shock (ES) | Inescapable electric foot shocks (shock duration and current depend on frequency), sometimes combined with situational reminders. | Open-field test, noise test, elevated plus-maze test | Not investigated | [55–57] |
Underwater trauma (UT) | One-minute forced swim followed by 20–45 seconds of forced submersion in a water tank. | Transfer test | Not investigated | [58, 59]. |
Restraint stress (RS) | Immobilization on wooden boards or in a restraint container (e.g. syringe, wire mesh tube, 50 mL centrifuge tube) for 1–2 hours. | Elevated plus-maze test, biomarker assays | Yes | [60–63] |
Single prolonged stress (SPS) | Two-hour restraint followed by a 20-minute forced swim and diethyl ether anesthesia until loss of consciousness. | Biomarker assays | Not investigated | [64] |
Predator-based psychosocial stress (PPS) | Exposure to a natural predator or predator-related stimuli, sometimes coupled with unstable housing conditions for 3–4 weeks. | Elevated plus-maze test, noise test, radial-arm water maze, novel object recognition, biomarker assays | Not investigated | [65, 66] |
Social defeat (SD) | Conspecific trained aggressor exposure for 6 hours daily for 5 or 10 days. | Biomarker assays, open-field test, forced swim test, sucrose preference test, tail suspension test, partition test ethogram evaluation, urine marking test | Yes | [67, 68] |
Social isolation (SI) | Social isolation (complete or near-complete lack of social interaction) during 3–4 weeks. | Training test, contextual test, cued test, extinction test | Yes | [69, 70] |
Trauma witness (TW) | Witnessing the social defeat event three times in one week. | Open-field test, elevated plus-maze test, light-dark exploration, forced swim stress, sucrose preference test, memory function test | Not investigated | [71] |
Early life stress (ELS) | Separating animal pups from mothers for several hours daily during postnatal days 1–10, followed by re-exposure to stressors (e.g. underwater trauma) in adulthood. | Biomarker assays, elevated plus-maze test, acoustic startle response test, ultrasonic vocalizations, open-field test | Yes | [72–74] |
Tail suspension (TS) | Suspension by the tail for 2–4 weeks. | Not enumerated | Yes | [75, 76] |
Chronic variable stress (CVS) | Exposure to variable stressors for 5–9 weeks, e.g. overnight illumination, changing cage mates, food deprivation and so on. | Biomarker assays, sucrose preference test | Yes | [48, 49] |
Chronic subordinate colony housing paradigm (CSC) | Housing in prolonged subordination to a dominant resident mouse for 19 consecutive days. | Biomarker assays, elevated plus-maze test | Not investigated | [77] |
Drug induction | Daily intraperitoneal injections of ISO solution (10 mg/kg) for 4 weeks. | Biomarker assays | Yes | [48, 49] |
Daily subcutaneous injections of CORT solution (20 mg/kg) for 6 weeks. | Tail suspension test, forced swimming test, open field test | Not investigated | [78] |
Currently, osteoporosis is categorized into primary and secondary types. Primary osteoporosis involves gonadectomy and aging, while secondary osteoporosis includes conditions such as diabetes, drug induction, disuse, psychological stress, and radiation exposure [84]. Table 2 outlines specific methodologies used to create models related to osteoporosis. Notably, some of these methods have been observed to trigger sympathetic activation (Table 2). For instance, Sui et al. uncovered that various osteoporotic mouse models such as ovariectomized mice and aging mice exhibited a significant increase in serum levels of the sympathetic activation marker–NE, but the mechanism remains unclear [48]. Moreover, the intricate distribution of nerves, blood vessels, and other components in bone, along with their interactions, remains a crucial area for investigation. In appropriate animal models, further elucidating whether and how the SNS regulates bone homeostasis through influencing blood vessels and other structures is of paramount importance in better understanding the relationship between psychological stress and osteoporosis.
Models . | Methods . | Sympathetic activation . | Ref. . |
---|---|---|---|
Gonadectomy | Ovariectomy (OVX) or orchidectomy (ORX). | Yes | [48] |
Aging | Natural aging or using senescence accelerated-prone mice. | ||
Drug induction | Daily intraperitoneal injection of dexamethasone (20 mg/kg) for 4 weeks. | ||
Disuse | Tail suspension or hindlimb immobilization for 2 weeks. | ||
Psychological stress | Exposure to various stressors or daily ISO induction (10 mg/kg) for 4 weeks. | ||
Type 1 Diabetes | Daily intraperitoneal injection of streptozotocin (50 mg/kg) for 5 days. | ||
Type 2 Diabetes | Induction of insulin resistance via high-fat diet or other means. | Yes | [85] |
Radiation | Focal radiation as a single dose of 20 Gy. | Not investigated | [86] |
Models . | Methods . | Sympathetic activation . | Ref. . |
---|---|---|---|
Gonadectomy | Ovariectomy (OVX) or orchidectomy (ORX). | Yes | [48] |
Aging | Natural aging or using senescence accelerated-prone mice. | ||
Drug induction | Daily intraperitoneal injection of dexamethasone (20 mg/kg) for 4 weeks. | ||
Disuse | Tail suspension or hindlimb immobilization for 2 weeks. | ||
Psychological stress | Exposure to various stressors or daily ISO induction (10 mg/kg) for 4 weeks. | ||
Type 1 Diabetes | Daily intraperitoneal injection of streptozotocin (50 mg/kg) for 5 days. | ||
Type 2 Diabetes | Induction of insulin resistance via high-fat diet or other means. | Yes | [85] |
Radiation | Focal radiation as a single dose of 20 Gy. | Not investigated | [86] |
Models . | Methods . | Sympathetic activation . | Ref. . |
---|---|---|---|
Gonadectomy | Ovariectomy (OVX) or orchidectomy (ORX). | Yes | [48] |
Aging | Natural aging or using senescence accelerated-prone mice. | ||
Drug induction | Daily intraperitoneal injection of dexamethasone (20 mg/kg) for 4 weeks. | ||
Disuse | Tail suspension or hindlimb immobilization for 2 weeks. | ||
Psychological stress | Exposure to various stressors or daily ISO induction (10 mg/kg) for 4 weeks. | ||
Type 1 Diabetes | Daily intraperitoneal injection of streptozotocin (50 mg/kg) for 5 days. | ||
Type 2 Diabetes | Induction of insulin resistance via high-fat diet or other means. | Yes | [85] |
Radiation | Focal radiation as a single dose of 20 Gy. | Not investigated | [86] |
Models . | Methods . | Sympathetic activation . | Ref. . |
---|---|---|---|
Gonadectomy | Ovariectomy (OVX) or orchidectomy (ORX). | Yes | [48] |
Aging | Natural aging or using senescence accelerated-prone mice. | ||
Drug induction | Daily intraperitoneal injection of dexamethasone (20 mg/kg) for 4 weeks. | ||
Disuse | Tail suspension or hindlimb immobilization for 2 weeks. | ||
Psychological stress | Exposure to various stressors or daily ISO induction (10 mg/kg) for 4 weeks. | ||
Type 1 Diabetes | Daily intraperitoneal injection of streptozotocin (50 mg/kg) for 5 days. | ||
Type 2 Diabetes | Induction of insulin resistance via high-fat diet or other means. | Yes | [85] |
Radiation | Focal radiation as a single dose of 20 Gy. | Not investigated | [86] |
Vascular regulation and bone homeostasis
Vasculature coupled with bone formation and regeneration
Blood vessels are widely distributed in tissues and organs and perform the physiological function of blood transport and substance exchange by joining arteries, capillaries, and veins in sequence as a continuous and relatively closed tube system. Blood vessels also have a role in the physiological and pathological processes of bone, such as skeletal development, regeneration, hematopoiesis, and homeostasis regulation [87]. The connection between vascularization and osteogenesis is illustrated by endochondral ossification. Hypertrophic chondrocytes (HCs) begin hypoxia-inducible factor (HIF) signaling, enhance the expression of vascular endothelial growth factor (VEGF) target genes, and promote blood vessel formation [88] in the primary ossification center (POC). Matrix metalloproteinases (MMPs) are secreted by osteoclasts and endothelial cells (ECs), which degrade the extracellular matrix (ECM) and increase VEGF signaling. VEGF-mediated signaling promotes migration and proliferation of these cell types [89, 90] when ECs and osteoblasts express VEGFR2.
Researchers are increasingly interested in the relationship between angiogenesis and osteogenesis. Kusumbe et al. discovered in 2014 that different vascular types exhibit heterogeneity of ECs in bone. ECs in the metaphysis and endosteum had high expressions of CD31 and endomucin (EMCN) (CD31hiEMCNhi), identifying them as type H vessels. At the same time, ECs in the bone shaft had low CD31 and EMCN (CD31loEMCNlo) expressions, identifying them as type L vessels [91]. Type H vessels are in tight contact with osteoprogenitor cells to provide necessary nutrients for bone formation, satisfy metabolic demands of bone development, and maintain bone homeostasis [92–94]. The expression levels of platelet-derived growth factor (PDGF), transforming growth factor (TGF), and other cytokines related to osteoprogenitor cell survival and proliferation were significantly higher in type H endothelium than in type L endothelium. Furthermore, in the metaphysis and endosteum, MSCs positive for platelet-derived growth factor receptor β (PDGFRβ), osteoblast-lineage cells positive for runt-related transcription factor 2 (RUNX2), and osteoblast-specific transcription factor (OSX) aggregate with type H endothelium. As a result, they exhibit a substantial spatial correlation, demonstrating the maintenance effect of type H endothelium on osteoblast-lineage cells.
Vascular regulation by sympathetic nervous system
The regulation of angiotasis requires the intricate integration of several signals that promote vasodilation and vasoconstriction to maintain blood pressure and volume, ensuring appropriate oxygen supply to metabolically active tissues. There is a state of tensional balance between the vasoactive substances released by the endothelium and the neurotransmitters produced by sympathetic nerve terminals [95]. The endothelium produces and releases numerous vasoactive substances in normal conditions, including endothelin 1 (ET-1) and angiotensin II (Ang II) for vasoconstriction and nitric oxide (NO), prostacyclin (PGI2), and endothelium-derived hyperpolarizing factor (EDHF) for vasodilation [96]. Under sympathoexcitation, the released NE causes systemic vasoconstriction and increases blood pressure by causing smooth muscle contraction via increasing the intercellular concentration of Ca2+ (Fig. 2). The endothelium inhibits sympathetic activity by promoting the metabolism and breakdown of NE to relieve vasoconstriction, providing a physical barrier to prevent NE diffusion into the bloodstream [97]. Moreover, in the coronary and large arteries, the activation of α2-ARs and β-ARs expressed on ECs leads to NO release and further vasodilation [98].
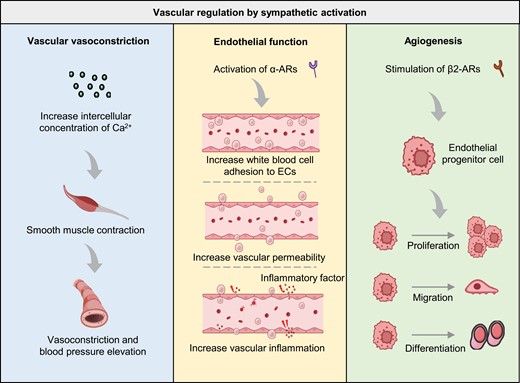
Sympathetic activation governs various aspects of vascular function.Sympathetic activation influences vascular vasoconstriction via Ca2+ concentration modulation. Endothelial functions, including adhesion, permeability, and inflammation, are regulated by the activation of α-ARs during sympathetic activation. Additionally, angiogenesis of endothelial progenitor cells (EPCs) is governed by β2-AR stimulation, which promotes EPC proliferation, migration, and differentiation. α-AR, alpha-adrenergic receptor; β2-AR, beta 2-adrenergic receptor; EPC, endothelial progenitor cell.
The SNS has been found to not only affect vasoconstriction and vasodilation, but also influence endothelial function and angiogenesis through Ars, with the continuous deepening of research on neurovascular interactions (Fig. 2). Angiogenesis refers to the biological process of producing new blood vessels on existing vasculature, often by sprouting and intussusception, and it is critical in physiological and pathological processes [99, 100]. Intussusceptive angiogenesis is the process by which a single blood vessel divides to form two lumens, also known as splitting angiogenesis [101, 102]. Sprouting angiogenesis involves forming new blood vessels from existing ones by endothelial sprouting [103, 104]. Studies have shown that the activation of α-ARs leads to endothelial dysfunction characterized by increased white blood cell adhesion to ECs, vascular inflammation, and increased vascular permeability [105–107]. Furthermore, stimulating β2-ARs on endothelial progenitor cells (EPCs) leads to its proliferation, migration, differentiation, and angiogenesis [108].
Specialized neurovascular coupling regulated by sympathetic nervous system
Bone blood vessels are densely distributed and continually transport oxygen, nutrients, and waste. At the same time, they also play a critical role in physiological and pathological processes. The discovery of type H vessels confirms the heterogeneity of ECs and provides evidence supporting the theory of angiogenesis–osteogenesis coupling. Research on promoting bone formation by type H vessels is increasing, highlighting the importance of specialized vasculature in bone homeostasis and regeneration.
Notably, close spatial connections between sympathetic nerves and type H vessels in metaphysis have been proven, in which ADRBs, particularly ADRB2, negatively regulate type H vasculature and bone homeostasis (Fig. 3).
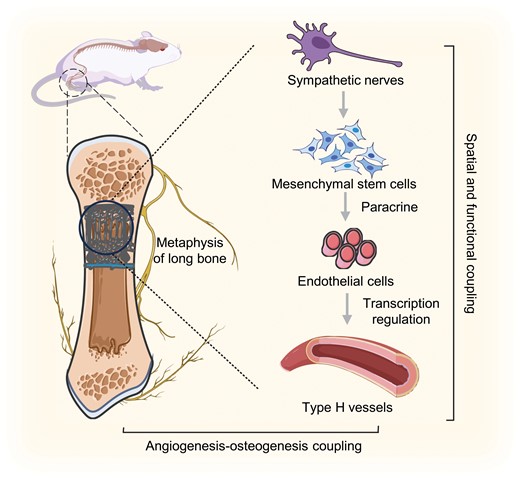
Neurovascular coupling and their regulation in bone homeostasis and regeneration under sympathetic activation.Close spatial connections and functional coupling exist between sympathetic nerves and type H vessels in the metaphysis. Sympathetic activation stimulates MSCs to transcriptionally regulate type H vessel angiogenesis via paracrine effects, subsequently impacting bone homeostasis and regeneration through the coupling of angiogenesis and osteogenesis.
Sympathoexcitation induces a reduction in type H vessels and bone mass in vivo, primarily through indirect sympathetic modulation of angiogenesis mediated by the paracrine effects of mesenchymal stem cells (MSCs). This regulatory mechanism involves alterations in the transcriptional activity of several angiogenic genes within ECs.
Xu et al. elucidated a distinct type of neurovascular coupling, proposing a novel approach centered on neural signaling and targeted vasculature for osteoanabolic therapies. Although the mechanisms by which pericytes respond to sympathetic activation and influence the formation of type H vessels through paracrine mechanisms remain unclear, this indirect regulatory mechanism may provide a new perspective for understanding neurovascular coupling in bone. EVs, as a crucial mode of intercellular signaling, have the capability to indirectly respond to sympathetic activation, thereby regulating bone homeostasis. Hence, investigating whether and how they participate in the sympathetic regulation of vasculature holds significant importance in further elucidating the mechanisms behind sympathoexcitation in regulating specialized type H endothelium and its potential effects on bone homeostasis and regeneration under psychological stress.
Extracellular vesicles and vascular regulation
Functions and applications of extracellular vesicles
EVs are small membrane-bound vesicles secreted actively by cells [109]. Initially, they were considered a form of waste excreted by cells. However, Harding and Pan discovered in 1983 that reticulocytes secreted small vesicles during maturation, transferring transferrin to the extracellular space [110, 111]. Later, Raposo et al. found that EVs secreted by B lymphocytes activated T lymphocytes and participated in immune regulation [112]. Since then, the functions of EVs have gained more attention. EVs are categorized according to their size and are distinguished as exosomes (40–160 nm), microvesicles (100–1000 nm), and apoptotic bodies (1000–5000 nm) [113, 114]. EVs remove excess or unnecessary components from cells to maintain cellular homeostasis and play a vital role in regulating intercellular communication by carrying proteins, nucleic acids, small molecules, and structural signaling molecules [115–117]. The key and complex processes that mediate intercellular communication include EVs recognition, binding, cellular uptake, and intracellular transport. EVs possess integrins, ECM proteins, lectins, proteoglycans, and glycolipids, enabling them to recognize and bind to specific receptors on target cells [118]. Subsequently, EVs mainly deliver their contents to the interior of cells by binding to receptors on target cells, fusing with the target cell plasma membranes, or being internalized, exerting biological effects.
The potential of EVs for diagnosing and treating several diseases, including neurodegenerative diseases and cardiovascular diseases, has recently been revealed [119–122]. EVs are abundant in blood and other bodily fluids, and their amounts and cargo of nucleic acids, proteins, lipids, and other substances reflect the physiological and pathological processes of the body, prompting them to be examined as biomarkers for various diseases [123]. Regarding treatment, EVs include functional substances, such as exogenous drugs, therapeutic RNA molecules, or proteins. They have been changed on their surface to create biological effects on receptor cells, allowing them to be used as a functional therapy for specific diseases [124–126].
Angiogenesis regulation by EVs
EVs control several physiological and pathological processes by delivering bioactive molecules. Numerous studies have shown that EVs play a critical role in angiogenesis regulation. For example, EVs produced from ECs contain β1 integrin, MMP-2, and MMP-9, which promote EC invasion and capillary-like structure formation [127]. EVs derived from endothelial colony-forming cells (ECFCs), which possess clear endothelial differentiation potential and proliferation activity, engage with α4 and β1 integrins to activate angiogenesis by conveying mRNA from the eNOS and PI3K/Akt signaling pathways [128, 129]. Recently, EVs have been considered potential mediators of remote ischemic adaptation and general protective signals. The ability of EVs to regulate angiogenesis offers new therapeutic opportunities for regenerative medicine and tissue engineering [130, 131]. Intramyocardial treatment with EVs can increase the perfusion of ischemic myocardial tissue by inducing arteriole and capillary growth in chronic ischemic heart disease [132]. EVs derived from ECFCs prevented capillary rarefaction and subsequent tissue damage in a rat model of renal ischemia-reperfusion injury [133]. EVs secreted by ECFCs enhance angiogenesis and accelerate wound healing by strengthening blood vessels at the wound site [134].
Furthermore, MSC-EVs has been confirmed to promote the formation of type H vessels in bone. Zhuang et al. induced the proangiogenic activity of MSCs through a hypoxic microenvironment. They confirmed that miR-210-3p, upregulated in small EVs derived from hypoxic MSCs, downregulated EFNA3 expression and enhanced the phosphorylation of the PI3K/AKT pathway, which facilitated the proliferation, migration, and angiogenesis abilities of ECs and ultimately enhanced bone regeneration and type H vessel formation in the critical size calvarial bone defect model [135]. Not only in bone, MSC-EVs have also been proven to promote the formation of type H vessels in the skin. Liu et al. demonstrated that EVs derived from engineered mesenchymal stem/stromal cell aggregates enriched angiogenic proteins. These proteins activated the Notch-related signaling pathway, promoting the formation of type H vessels in diabetic wounds, and ultimately facilitating their healing [136].
In summary, EVs are intercellular communication mediators used for diagnosing and treating various diseases. EVs produced from blood-related cells or other sources have been demonstrated to influence angiogenesis and have effective therapeutic benefits. However, it is still unknown whether sympathetic under psychological stress affects the role of EVs in angiogenesis regulation. Type H vessels are tightly coupled with bone homeostasis and regeneration, and it is unclear how they respond to the regulation of endogenous EVs and the effects of exogenous EVs on type H vessel maintenance in the context of sympathetic activation. Therefore, it is critical to investigate the role of EVs in specialized regeneration-related vascular structure regulation under sympathetic activation to develop therapy methods for bone loss caused by psychological stress.
MSC-EVs regulate angiogenesis and bone regeneration
MSC-EVs in angiogenesis regulation
MSCs are a type of adult non-hematopoietic stem cell discovered by Friedenstein et al. in bone marrow. They possess characteristics such as adhesion to plastic, self-renewal, clonogenicity, and multipotential differentiation abilities [137, 138]. MSCs are isolated from various body parts, including blood, bone marrow, liver, umbilical cord, periodontal ligament, lung, and adipose tissue [139] (Fig. 4). During tissue regeneration, it was initially believed that MSCs exerted therapeutic effects by migrating to the injury site, engrafting, and subsequently differentiating into the cells required for tissue regeneration. However, increasing studies have shown that the therapeutic benefits of MSCs are attributed to their differentiation and the active substances they secrete, especially the role of EVs, which has received extensive attention [140–142]. MSC-EVs transmit information to damaged cells or tissues, participate in tissue regeneration and functional repair, regulate extracellular matrix remodeling, limit local inflammation, and regulate immune responses [143–145]. Numerous studies have found that EVs released by MSCs have therapeutic potential in repairing diseases and functional damage in various tissues and organs, such as the lung, kidney, liver, nervous system, bone, cartilage, and heart [146–152].
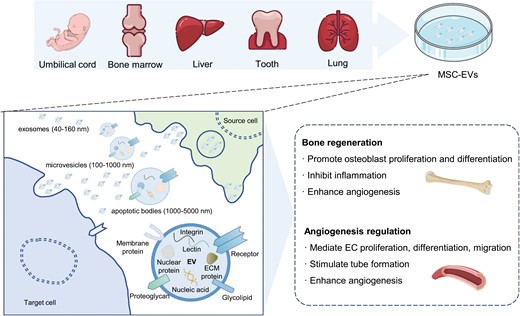
Regulation of angiogenesis and bone regeneration by MSC-EVs.MSCs are derived from various tissues, including the umbilical cord, bone marrow, liver, tooth, and lung. MSC-EVs encompass exosomes, microvesicles, and apoptotic bodies, containing diverse components like proteins and nucleic acids. MSC-EVs are employed for promoting bone regeneration and regulating angiogenesis. MSC, mesenchymal stem cells; MSC-EVs, mesenchymal stem cell extracellular vesicles.
Several studies have demonstrated the significant role of MSC-EVs in promoting angiogenesis [153, 154] (Fig. 4). For instance, under hypoxic stimulation, bone marrow-derived MSC-EVs are absorbed by ECs, thereby promoting angiogenesis and enhancing cardiac function in rat myocardial infarction models [155]. After low oxygen treatment, umbilical cord-derived MSC-EVs improve the formation of capillary-like structures and promote blood flow recovery in rat models of hindlimb ischemia [156, 157]. Moreover, umbilical cord-derived MSC-EVs overexpressing Akt stimulate EC migration, proliferation, and tube formation in vitro, increasing blood vessel formation in vivo [158]. Similarly, stem cells from human exfoliated deciduous teeth (SHED)-derived MSC-EVs mediate endothelial cell differentiation and enhance angiogenesis by transferring miR-26a via the TGF-β/SMAD2/3 signaling pathway [159].
MSC-EVs in bone regeneration
In recent years, multiple studies have confirmed that MSC-EVs directly promote bone cell proliferation, differentiation, and angiogenesis while inhibiting inflammatory responses in various pathological conditions [160–163] (Fig. 4). Treatment in the osteonecrosis model with MSC–EV increased trabecular bone cell numbers, decreased apoptotic cell numbers, and reduced adipose tissue [164]. MSC-EVs in the fracture model promoted bone callus formation and enhanced bone healing [165]. Profound remodeling of newly formed bone in the osteoporosis model was observed with MSC-EVs treatment. In contrast, the control group without MSC-EVs treatment exhibited bone loss or minimal mineralization and bone formation [166]. Furthermore, MSC-EVs promote angiogenesis in osteoporotic rats, which is beneficial for bone formation [163]. More experiments have also revealed the potential mechanism of MSC-EVs in bone regeneration therapy. MSC-EVs may promote the osteogenic differentiation of bone marrow MSCs and induce osteogenesis by regulating the PI3K/Akt signaling pathway [167]. Additionally, MSC-EVs stimulate the proliferation and differentiation of osteoblasts by regulating the BMP/Smad/Wnt signaling pathway, thereby promoting osteogenesis [165, 166].
Although MSC-EVs have shown great potential in regenerative therapy, their application is limited by low natural production. In recent years, engineering techniques have been developed to modify MSC-EVs to avoid potential pathogenic risks and enhance their efficacy and production to meet the demand for MSC-EVs in diseases [167]. Engineering MSC-EVs involves surface modification and content loading. Surface modification is achieved through cosmetic cell modification and non-covalent or covalent membrane binding to make MSC-EVs target specific cells or reduce the probability of being cleared [168]. Loading functional components, including nucleic acids, proteins, and small molecules, into the lipid membrane bilayer structure on the surface of MSC-EVs and the hydrophilic core surrounded by the lipid membrane is known as content loading. Passive loading involves co-incubating functional therapeutic substances with EVs or donor cells, whereas active loading involves ultrasonic treatment, extrusion procedures, electroporation, multiple freeze-thaw, and other techniques [152, 169, 170]. While passive loading is straightforward and maintains the EV membranes intact, it has poor loading efficiency. On the other hand, active loading can provide a high loading efficiency for hydrophilic or macromolecular compounds but might result in issues, such as damage to membrane integrity or condensation [171–173].
Overall, MSC-EVs derived from MSCs with multipotent differentiation potential promote tissue regeneration, limit local inflammation, regulate immune responses, and effectively promote angiogenesis and bone regeneration. However, it is unclear how MSC-EVs respond to and exert their regenerative-promoting effects in the overall environment under sympathetic activation. The low natural production and lack of specificity of MSC-EVs limit their broad application in regenerative medicine. Therefore, engineering modifications of MSC-EVs to improve their targeting and therapeutic effects will better meet the demand for MSC-EVs in bone regeneration. Modified MSC-EVs with proangiogenic and pro-osteogenic effects will provide new options and directions for treating bone loss under psychological stress.
Summary and prospectives
Psychological stress is closely related to the development and progression of several diseases, including bone loss and osteoporosis. SNS, one of the neuroendocrine foundations of psychological stress, has been shown to significantly contribute to this process via overactivation or malfunction. However, the mechanism underlying sympathetic activation-induced bone loss remains unknown, and no viable therapy strategies are available. Recent research suggests sympathetic activation modulates bone and vascular cell homeostasis by acting on bone cells and ECs. Notably, the close distribution and mutual interaction of nerves and blood vessels in bone suggest that sympathetic activation might participate in bone regeneration by influencing vascular function. Identifying type H vessels, a specialized vasculature linked to bone regeneration highlights the significance of vascularization in modulating the response to sympathetic activation-induced bone loss. An emerging therapy based on EVs, namely MSC–EVs, is proposed to repair bone loss under psychological stress, with favorable proangiogenic and pro-osteogenic effects. We also propose engineering modifications to improve the targeting and therapeutic benefits of MSC-EVs to develop an effective novel technique for treating osteoporosis under psychological stress.
Acknowledgements
This work is supported by grants from the National Natural Science Foundation of China (Nos. 82100969, 81930025, 82371020 and 82301028), the Key research and Development Program of Shaanxi Province (No. 2023-YBSF-189), the Natural Science Basic Research Program of Shaanxi Province (No. 2022JQ-929), the Special Project of National Clinical Research Center for Oral Diseases (No. LCB202006), the Shaanxi Province Innovation Capability Support Program Scientific and Technological Innovation Team (No. 2023-CX-TD-69), the National Defense Biotechnology Outstanding Young Talents Fund (No. 01-SWKJYCJJ24), the “Rapid Response” Research projects (No. 2023KXKT017), the China Postdoctoral Science Foundation (No. BX20230485) and the Young Science and Technology Rising Star Project of Shaanxi Province (No. 2023KJXX-027). Figures 1 to 4 were modified from Servier Medical Art (smart.servier.com), licensed under a Creative Common Attribution 3.0 Generic License (creativecommons.org/licenses/by/3.0).
Author contributions
Hao-Kun Xu (Funding acquisition [Supporting], Writing—original draft [Equal], Writing—review & editing [Supporting]), Jie-Xi Liu (Writing—original draft [Equal], Writing—review & editing [Supporting]), Ze-Kai Zhou (Writing—original draft [Equal]), Chen-Xi Zheng (Writing—review & editing [Supporting]), Bing-Dong Sui (Writing—review & editing [Supporting]), Yuan Yuan (Writing—original draft [Supporting]), Liang Kong (Funding acquisition [Supporting], Supervision [Supporting], Writing—review & editing [Supporting]), Yan Jin (Conceptualization [Equal], Funding acquisition [Supporting], Supervision [Supporting], Writing—review & editing [Supporting]), and Ji Chen (Conceptualization [Equal], Funding acquisition [Lead], Supervision [Lead], Writing—review & editing [Lead]).
Conflict of interest
The authors declare no competing interests.
References
Author notes
Hao-Kun Xu, Jie-Xi Liu and Ze-Kai Zhou contributed equally to this work.