-
PDF
- Split View
-
Views
-
Cite
Cite
Bipin K Pandey, Timothy S George, Hannah V Cooper, Craig J Sturrock, Tom Bennett, Malcolm J Bennett, Root RADAR: how ‘rhizocrine’ signals allow roots to detect and respond to their soil environment and stresses, Journal of Experimental Botany, Volume 76, Issue 6, 9 April 2025, Pages 1500–1509, https://doi.org/10.1093/jxb/erae490
- Share Icon Share
Abstract
Agricultural intensification coupled with changing climate are causing soils to become increasingly vulnerable to stresses such as drought, soil erosion, and compaction. The mechanisms by which roots detect and respond to soil stresses remain poorly understood. Recent breakthroughs show that roots release volatile and soluble hormone signals into the surrounding soil, then monitor their levels to sense soil stresses. Our review discusses how hormones can act ‘outside the plant’ as ‘rhizocrine’ signals that function to improve plant resilience to different soil stresses. We also propose a novel signalling paradigm which we term ‘root RADAR’ where ‘rhizocrine’ levels change in soil in response to environmental stresses, feeding back to roots and triggering adaptive responses.
Introduction
Plant root systems forage for water and nutrients in soil substrates that are physically, chemically, and biologically complex (Lehmann et al., 2020; Harris et al., 2022). A typical soil consists of a matrix of particles of different types and sizes, interspersed with air spaces (pores), which experience dynamic changes in hydration status (Brady and Weil, 1999; Grusson et al., 2021). This physical environment also contains inorganic mineral ions, some that act as plant nutrients (ammonium, nitrate, phosphate, etc.), others which are toxic (e.g. cadmium and lead), and some that are both (iron, copper, etc.). Finally, soil also hosts a diverse array of organisms (bacteria, fungi, insects, etc.), including plants themselves, all of which release complex organic compounds into the soil (Gregory, 2008). Hence, a typical soil represents a highly heterogeneous environment for plant growth.
To fulfil its foraging function, a root system must grow in such a way as to optimize the uptake of water and mineral nutrients. To facilitate this, plants have evolved acclimatory mechanisms to actively respond to soil biotic and abiotic factors by altering root placement patterns (Morris et al., 2017; de Britto Costa et al., 2021; Wang et al., 2023). Nevertheless, our understanding of how root systems can sense, integrate, and adapt to the sheer complexity and heterogeneity of soil, both to optimize their architecture and to maximize stress resilience, is very limited (Li et al., 2021; Colombi et al., 2024).
The ability of plants to detect different classes of chemicals in the rhizosphere (i.e. the thin layer of soil adjacent to roots) is critical to sense and adapt to their local environmental conditions. These chemicals include inorganic macro- and micro-nutrients, toxic elements, and many organic molecules released by other organisms (Perry et al., 2007; Rizaludin et al., 2021). Plants also release a wide array of low and high molecular weight chemicals to signal to other organisms (e.g. flavonoids) and modulate soil properties (e.g. exudates; Rasmann and Turlings, 2016; Massalha et al., 2017; Enagbonma et al., 2023; Wang et al., 2023). Many of these specialized signals derive from secondary metabolic pathways and represent a substantial component of the resources acquired by the plant, accounting for 10–20% of the total plant nitrogen and the photosynthetically fixed carbon (Jamil et al., 2022). These root-derived signalling molecules include volatile (low molecular weight and gaseous at ambient temperature, e.g. ethylene) and soluble [low or high molecular weight and non-gaseous, e.g. strigolactone (SL)] compounds (Fig. 1).
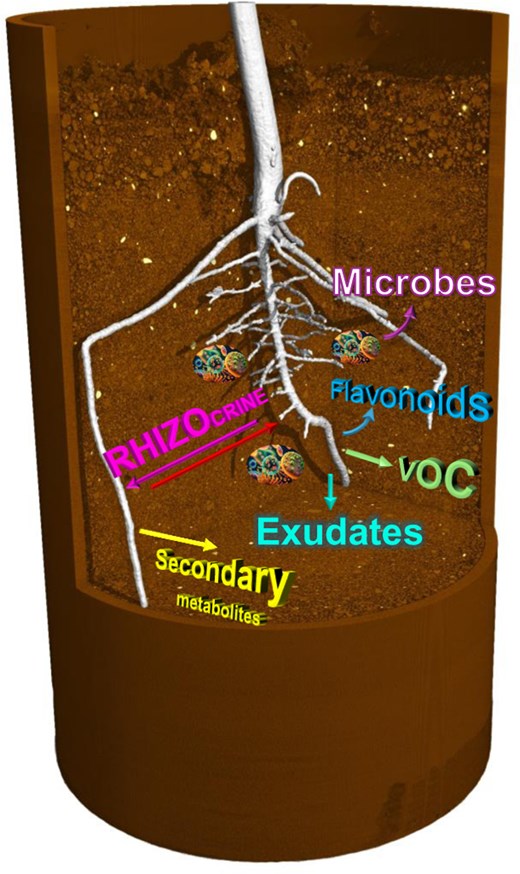
Plant roots release a wide array of chemicals to signal to other organisms and modify soil properties. Examples of different classes of root-derived signals are illustrated in the schematic.
Recent research reveals that plant roots release selected classes of signals into the rhizosphere for the apparent purpose of sensing changes in their soil environment and potentially associated stresses, which then feed back and modify root growth (Pandey et al., 2021; Wheeldon et al., 2022). Given that these signals (which include known hormones such as ethylene) spend time outside the plant in the rhizosphere, but act upon plants themselves, we have classified them as ‘rhizocrine’ signals (Fig. 2). By releasing ‘rhizocrine’ signals, then detecting changes in their concentration, roots can establish important information about the local biological, chemical, or physical environment in which they are growing. In this way, these signals act akin to RADAR (radio detection and ranging) used in aviation, where emission of a signal and detection of its reflection allow obstacles to be identified. The aim of this review is to first examine evidence for any ‘root RADAR’ signals that plants might use to ‘sense’ changes in the rhizosphere and then develop a conceptual framework explaining how these different ‘rhizocrine’ signals could help plants successfully adapt to specific soil stresses.
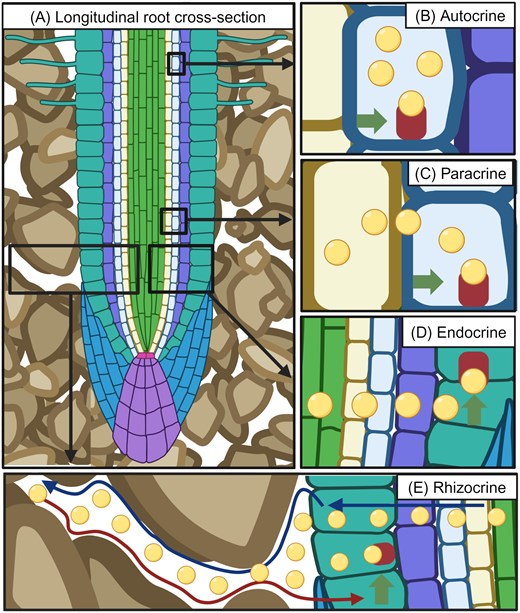
Roots employ several classes of hormones including rhizocrine signals that spend time outside of plant tissues in the rhizosphere. A schematic diagram illustrating (A) a longitudinal cross-section of a root growing in soil, and (B–E) examples of the contrasting modes of action for different hormone classes (denoted as yellow circles) involving (B) autocrine signalling (i.e. acting within the source cell), (C) paracrine signalling (i.e. acting close to the source cell), (D) endocrine signalling (i.e. acting in other organs after long-distance transport), and (E) rhizocrine signalling, where this class of hormone moves outside its root source via the soil (denoted by the blue arrow), returning to the root in response to a soil stress (denoted by the red arrow), triggering an adaptive response, denoted by the green arrow and receptor binding of the hormone signal.
Rhizocrine signalling: a conceptual framework
Classically, hormones have been classified as acting either on the cell in which the signalling molecule was originally produced (‘autocrine’; Fig. 2B), or on cells close to the signal source cell (‘paracrine’; Fig. 2C), or in tissues and organs distinct from the signal source cell, often involving long-distance transport (‘endocrine’; Fig. 2D). Despite their contrasting spatial scales of action, these categories of hormone act within the anatomical boundaries of the plant (Fig. 2A). While these classifications are not typically used when referring to plant hormones in the plant body (which are typically simultaneously auto-, para-, and endocrine by these definitions), we are proposing the term ‘rhizocrine’ to refer to a class of signal that is released beyond the anatomical boundaries of the root (Fig. 2E), into the soil environment, but which ultimately signals to roots on the same (or adjacent) plant. By spending time ‘outside’ the plant hormone’s organismal source, rhizocrine signals function more like animal ‘exocrine’ (rather than endocrine) signals such as pheromones released externally by exocrine glands in termites, for example (Costa-Leonarda and Haifig, 2010).
Distinct classes of rhizocrine signals could exist that adopt different modes of communication (Fig. 3). For example, using analogies to different phone modalities, volatile rhizocrine classes (e.g. ethylene) can be compared with satellite communication as these signals are likely to transmit over long distances in soil via interconnected micropore networks. In contrast, rhizocrine signals transmitted between roots via mycorrhizae networks (e.g. jasmonates) can be compared with communicating via a ‘land line’. Rhizocrine signals acting over just a very short distance from the root surface, such as the depth of the rhizosphere (i.e. <1 mm), can be considered to mimic ‘Bluetooth’ communication.
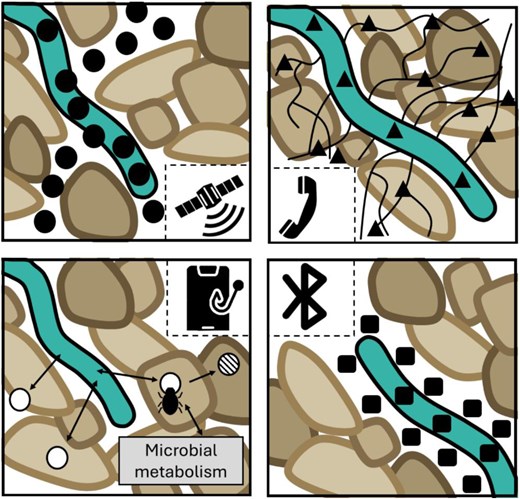
The different signalling modes of action for rhizocrine signals. The top left-hand panel shows the ‘satellite’ class of rhizocrine signal travelling long distances through the soil. The top right-hand panel shows the ‘landline’ class of rhizocrine signal which utilizes mycorrhizae networks to travel via soil to trigger root responses. The bottom right-hand panel shows the ‘Bluetooth’ class of rhizocrine signal where hormone release is limited to the rhizoplane. The bottom left-hand panel shows the process of ‘phone-hacking’ where microbes degrade and alter rhizocrine hormone levels to manipulate adaptive responses by the root.
In general, the distance over which rhizocrine signals act changes depending on physical and environmental stress conditions in the soil. For example, gaseous signals can move long distances in soil when air-filled micropore networks permit rapid gas diffusion (Fig. 3). However, water logging will disrupt diffusion of gaseous signals as soil pores will be blocked. Conversely, drying soil will prevent diffusion of soluble rhizocrine signals, increasing their concentration near to the root surface. For example, the concentration of rhizocrine signals belonging to this ‘Bluetooth’ class [which may include soluble hormones such as abscisic acid (ABA)] is likely to increase when rhizosphere moisture levels drop, providing a potentially important extracellular source of this abiotic stress signalling molecule. The ability of roots to probe their surrounding environment using a suite of hormones also enables plants to directly regulate their growth, development, and physiology in response to soil stresses. Since soil stresses are often highly heterogeneously distributed (e.g. soil compaction or areas of low hydration), a signalling solution operating at a local scale is essential.
Signalling in the rhizosphere: a noisy neighbourhood …
Knowledge of rhizosphere signals has increased exponentially in recent years (Fig. 1). Classic examples include flavonoids and Nod factor signals exchanged between legume roots and rhizobia during nodulation (Phillips and Tsai, 1992; Hassan and Mathesius, 2012). Similarly, mycorrhizal colonization of roots involves exchange of SYM and SL signals (Philips and Tsai, 1992; Nadal and Paszkowski, 2013; Clark and Bennett, 2024). More recent discoveries include plant signals such as salicylic acid (SA) and methyl jasmonate (MeJA), inducing assembly and/or biofilm formation in soil microbiome communities (X. Wang et al., 2023; Kulkarni et al., 2024; Li et al., 2024). Many of these plant signals are reported to communicate with organisms from other kingdoms of life such as l-DOPA which acts as a plant pheromone for below-ground anti-herbivory (Cascone et al., 2023). However, could some of these root-derived molecules released into the rhizosphere, many of which include classical plant hormones (Table 1), primarily function to signal to plant roots?
Examples of root-derived hormones and signalling molecules detected in the rhizosphere and bulk soil fractions for a range of plant species
Examples of root-derived hormones and signalling molecules detected in the rhizosphere and bulk soil fractions for a range of plant species
Plant hormones are hypothesized to be part of a diverse group of metabolites that acquired a regulatory role over the course of embryophyte evolution (Gasperini and Howe, 2024). Plant hormones are classically known to regulate root growth and development (Banda et al., 2019). Recent evidence has shown that hormonal signals such as ABA also help roots adapt to dynamic changes in their soil environment such as transient water stress (Mehra et al., 2022). Intriguingly, many classes of plant hormone signals (including their precursors and modified forms) have been detected ‘beyond the root’ in the rhizosphere and bulk soil fractions for multiple plant species by independent labs (High et al., 2019; Zhao et al., 2011; Sheflin et al., 2019; Lu et al., 2021; Table 1). For example, this includes the gaseous hormone ethylene and its immediate precursor 1-amino cyclopropane-1-carboxylic acid (ACC), and SA and its methylated derivative (MeSA) (Table 1). Beyond classical hormones, there are many other low molecular weight molecules released by plants into the soil, which might act as signalling molecules within or between plants.
One of the important challenges in discovering potential new rhizosphere signals is to precisely measure these molecules in the rhizoplane, rhizosphere, and bulk soil fractions. Soil is a complex matrix with varying physical and chemical properties, which affect the diffusion and detection of molecules, especially gaseous molecules. Moreover, molecules can be adsorbed onto soil particles or degraded by soil microbes, making detection difficult. Due to the short-lived and dynamic nature of many potential signalling molecules, it is challenging to measure them at the appropriate time and place. Many of these molecules are produced by both plant roots and microbes, making it challenging to attribute specific compounds to a specific source. For example, the hormonal signals ethylene, cytokinin, ABA, SA, and MeJA are produced by both plant roots and microbes in the rhizosphere (Egamberdieva et al., 2017). One way to discriminate the source is to tag the signal in the plant biosynthesis pathway with either a fluorophore or any conjugating agent. Moreover, isotopic labelling (e.g. using 13C-labelled compounds) can help distinguish plant-derived molecules from microbial ones.
Most potential signalling molecules are released into the rhizosphere in very small amounts. For example, 10 g of fresh rice roots produce 0.2–1.5 nmol of ethylene. However, soil stresses such as submergence can increase ethylene levels by >25 times within hours (Hattori et al., 2009). Therefore, the development of direct measurement techniques for different plant-derived rhizosphere signals is of paramount importance. The current sampling methods are mostly invasive and require disrupting soil structures while measuring these signals. Measuring volatile organic compounds (VOCs) released by plant roots in soils presents a complex set of challenges due to the dynamic and interactive nature of the soil environment. However, researchers have used several methods to monitor VOC signals from soil conditions (summarized in Box 1).
Static and dynamic chambers enclose the root system or a section of the soil to collect gaseous/volatile signals over time. The headspace (the air above the soil or plant) is then sampled.
Separates and identifies compounds based on mass and charge. Headspace samples are injected into the GC-MS for analysis.
Allows for real-time monitoring of gaseous and volatile signals with high sensitivity and fast response time. Measures trace gases by proton transfer reaction ionization.
SPME-coated fibres are exposed to the headspace of soil or plant chambers to adsorb volatile signals. The fibres are then desorbed in the injector of a GC-MS. This method is non-destructive and does not require solvents, making it suitable for field studies.
Tubes packed with adsorbent materials collect gaseous signals, which are later desorbed thermally into a GC-MS for analysis. These tubes are useful for concentrating signals from low-concentration environments.
This is an advanced analytical technique for measuring volatile organic compounds (VOCs) in soils, allowing for high sensitivity, selectivity, and real-time monitoring of these compounds in complex soil matrices. It involves inserting a small, semi-permeable probe into the soil, allowing the collection of VOCs in situ.
Allows for direct sampling of volatile and gaseous signals in aqueous environments and is useful for studying volatiles dissolved in soil water. The membrane selectively allows volatiles to pass through, which are then ionized and analysed by MS. Real-time analysers, such as Fast-GC and laser-based spectroscopy, provide continuous monitoring of volatile signals without the need for sample collection.
FTIR detects VOCs in soils based on their unique infrared absorption spectra, which are linked to molecular vibrations. It provides molecular fingerprints for VOCs, enabling identification and quantification without requiring sample pre-treatment.
PAS detects VOCs by measuring sound waves generated by the absorption of light (laser) by VOC molecules. A laser source irradiates the headspace around a soil sample. VOCs in the headspace absorb the laser energy and emit sound waves that are detected by microphones.
Measurements of root-derived soluble molecules from soil fractions are often very challenging (Hassan and Mathesius, 2012). Indeed, most studies performed to date employ hydroponic approaches to harvest plant metabolites from root exudates (Oburger et al., 2022). Add to this that many of these rhizo-deposits will last for only very short periods in the hostile soil environment, for example flavonoids last <72 h (Hassan and Mathesius, 2012), and the challenge is clear. New approaches are needed to sample exudates and soluble molecules in situ from the rhizosphere and soil, such as quick and in situ detection of signalling chemicals in plant and soil by microdialysis combined with UPLC-MS. Once solved, many additional plant-derived signals are likely to be detected outside of root tissues in soil fractions in the future. In addition, access to in situ sampling approaches would help generate much greater understanding about the spatial and temporal changes in the distribution of root-derived signals in response to soil stresses. For example, does a soil stress cause selected signals to accumulate close to the root in the rhizosphere? Do soluble root-derived ‘rhizocrine’ signals form steeper gradients after experiencing a soil stress? Such insights are vital for understanding the relationship between soil stress and signals released from plants.
Rhizocrine signals: modulating root growth and resilience to soil stresses
So, what are these known and potential plant-derived signals doing outside the root? Recent evidence suggests that at least some of the hormonal signals released into the rhizosphere help plant roots to sense environmental stresses, then bring about root adaptive responses. For example, ethylene is released by roots into soil where it normally diffuses into the bulk soil via interconnected pores (Fig. 4; Pandey et al., 2021). However, compacted soil features reduced the numbers of interconnected pores. Impeded diffusion in the compacted rhizosphere will cause ethylene to accumulate around (and eventually within) root tissues, triggering growth inhibition (Fig. 4). Hence, ethylene provides a clear example of a ‘rhizocrine’ signal, that is to say one which is released into the rhizosphere and acts upon the root system that released it (Fig. 2). It also exemplifies the root RADAR paradigm, in which the extent of the ‘reflection’ of a signal released by a root allows detection of a stress in their soil environment (Fig. 4).
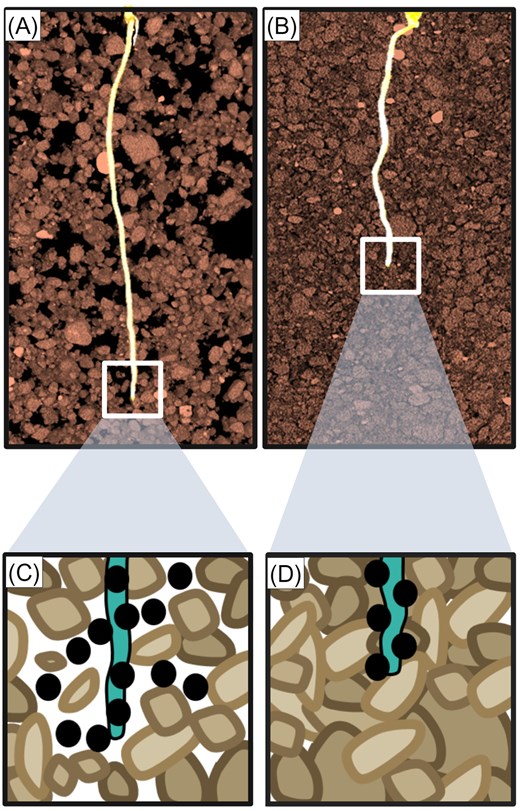
Levels of rhizocrine signals change in response a soil stress such as compaction to trigger a root adaptive response. (A and B) Computed tomography (CT) images showing higher porosity in uncompacted (A) versus compacted soil (B). (C and D) Schematic figures of ethylene diffusion (denoted by black circles) in uncompacted (C) versus compacted (D) soil, illustrating preferential accumulation of ethylene around and in root tissues when roots encounter a region of compacted soil.
SLs are another class of hormone which may act in a rhizocrine manner. SLs are well-established rhizosphere signalling molecules; indeed, this pre-dates their description as plant hormonal signalling molecules (Clark and Bennett, 2024). Mutants lacking SL synthesis have been shown to contain SLs when co-cultured with wild-type plants, implying that plants can re-absorb rhizospheric SL, and utilize it to regulate their growth (Wheeldon et al., 2022). It has been shown that dilution of a low molecular weight signal within the soil allows plants to detect their root growth space, since the larger the volume, the more diluted the signal becomes. Plants then use the concentration of the signal to adjust their early shoot growth, to match their size to their soil volume [and hence lifetime availability of nutrients and water (Wheeldon and Bennett, 2021)]. It has been suggested that this signal is SLs (Wheeldon et al., 2022; Yoneyama et al., 2022), and thus exudation of SLs into the soil, and their RADAR-like re-detection, allows plants to gauge their soil volume.
Thus, ethylene and SLs appear to reveal new ‘rhizocrine’ roles for plant hormones, where these signals spend time outside their organismal source in the rhizosphere, but ultimately act on the emitting plant. Whilst an attractive and logical mechanism, critical functional evidence is missing for other classes of plant-derived rhizosphere signals (Table 1). Indeed, we do not currently have a comprehensive list of root-derived signals released into soil, or of those which can help to confer resilience to an environmental stress. Nevertheless, ethylene and SLs point the way forward for further investigations of the other signalling molecules that plants release into the soil.
The adaptive basis of rhizocrine signalling
The evidence presented here critically evaluates the existence of rhizocrine signals, but an interesting conceptual discussion is whether this is the primary selective basis for such signalling. An alternative model is that rhizocrine signals have evolved primarily as signals ‘between’ roots on the same plant. Thus, the local concentration of rhizocrine signals provides roots with information on where other roots in the same system are, and, by growing away from high signal concentrations, roots grow away from each other, into uncolonized soil.
Another possibility is that these signals also communicate to the roots of other plants, which by triggering growth responses in the neighbouring roots, help to prevent invasion of a plant’s ‘territory’ (de Britto Costa et al., 2021; Wang et al., 2023). It is very difficult to distinguish between these possibilities, since—once the signals are in the rhizosphere—they will simultaneously perform all three functions whether intentionally or not. It would be virtually impossible for a plant to prevent other roots from detecting the signals released by a given root, and it is unlikely to be beneficial to do so anyway. Indeed, from the perspective of an individual root, it only needs to ‘know’ whether to vary its response (e.g. grow more/grow slow)—it does not matter exactly why it should undergo that response. For instance, a root approaching compacted soil, another root from the same plant, or a root from another plant should do the same thing—stop growing. If the individual roots make the right ‘decisions’, the plant as a whole benefits from exuding the signals. Indeed, if the plant ultimately has increased reproductive success as a result of exuding the signal into the rhizosphere, then it does not matter exactly why the signal is beneficial. Thus, rhizocrine signals are likely to simultaneously provide plants with information on both their physical and biological environment—though for different signals, one role may be more prominent that the others.
Rhizocrine signals and other soil organisms
The foregoing discussion highlights another likely feature of rhizocrine signalling, which is that a large variety of organisms present in the soil might ‘eavesdrop’ on these signals. This might include other plants, bacteria, fungi, parasitic nematodes, or insects, which in the latter cases would aid below-ground plant herbivory (Cascone et al., 2023). Thus, rhizocrine signalling may also have unintentional side effects that are neutral or detrimental, depending on which organisms are able to eavesdrop on the signals. The case of SLs is a particularly pertinent example of this. They undoubtedly evolved originally as molecules emitted to signal to mycorrhizal fungi (Akiyama and Hayashi, 2006; Yoshida et al., 2012; Gutjahr et al., 2015; Liu et al., 2019) in plants that lacked roots, but—once roots evolved in vascular plants—started to fulfil a secondary adaptive role as rhizocrine signals, and a tertiary adaptive role as plant-to-plant signals (Wheeldon et al., 2022; Yoneyama et al., 2022). However, parasitic plants eavesdrop on these signals to time their germination (Akiyama and Hayashi, 2006), and rhizospheric bacteria may also use these signals as indicators of host availability (Venturi and Keel, 2016).
Furthermore, organisms in the rhizosphere may even be able ‘hack’ rhizocrine signals, in order to manipulate plant growth or physiology to their benefit (Fig. 4). The most likely mechanism for this is by attenuating the signal, causing roots to perceive lower effective concentrations. Since plants represent the primary carbon source in soil for many microbes, encouraging roots to continue to grow and release carbon into soil is vital for microbial growth. Thus, hacking rhizocrine signals could carry significant benefits for soil microbes. This might, for example, potentially explain the widespread distribution of soil bacteria expressing the ACC deaminase enzyme (Nascimento et al., 2019), which targets the plant ethylene precursor ACC. Reducing levels of ACC will also lower ethylene, de-repressing root growth and encouraging carbon release into the rhizosphere. Indeed, such manipulations may have a selective basis, given the strong selective pressure between the plant and its microbiome, which can be considered to form a co-evolved unit termed a ‘holobiont’ (Bordenstein and Theis, 2015). Rhizocrine signals such as ethylene and ABA, that can be modulated by microbes, offer opportunities to co-evolve and optimize beneficial functional relationships between the plant host and soil microbiome to co-regulate important processes such as carbon deposition or water stress strategies, respectively.
Conclusion and future perspectives
Our Darwin Review discusses how plant hormones may act ‘outside the root’ as ‘rhizocrine’ signals on the releasing root, on other roots of the same plant, and most probably on the roots of neighbouring plants. These signals are likely to function to improve plant resilience to different environmental stresses, and such a model might help to explain the preponderance of low molecular weight molecules released into the rhizosphere by plants. We also propose a novel signalling paradigm termed ‘root RADAR’ where levels of ‘rhizocrine’ signals change in the soil in response environmental stresses, feeding back to roots and triggering adaptive responses. It is likely that other plant-derived molecules act in a similar manner, but identifying other signals that act as rhizocrine signals requires innovations in sampling and profiling of root and soil volatiles and exudates, and assays to demonstrate their functional importance.
Understanding how rhizocrine signals regulate root growth and physiology, and over which temporal and physical scale, offers new opportunities for crop breeding and engineering, particularly concerning improved resilience against soil stresses and interactions with other organisms. As an extension of this idea, genetic augmentation or attenuation of rhizocrine signalling is likely to provide promising targets to modulate resilience to specific soil stresses in field crops. For instance, since most crops grow in at least partly compacted soil, modulating ethylene sensitivity in roots may promote increased root growth under agricultural conditions, thereby improving water and nutrient uptake. Whilst root systems have rarely been the target of crop breeding programmes, improved understanding of rhizocrine signalling is likely to provide highly specific breeding targets that can improve crop performance in a changing climate.
Author contributions
All authors contributed equally to discussions, formulation of the core conceptual ideas, and writing of the article.
Conflict of interest
The authors declare they have no conflict of interest.
Funding
Biotechnology and Biological Sciences Research Council (BBSRC) grants BB/T001437/1, BB/W008874/1, and BB/W015080/1 and European Research Council grant HYDROSENSING (101118769) to MB. BBSRC grant BB/X001423/1 to TB. BP acknowledges UKRI Frontiers Research grant (EP/Y036697/1).
Comments