-
PDF
- Split View
-
Views
-
Cite
Cite
M Mar Castellano, Alfonso Muñoz, Isabel C Okeke, Esther Novo-Uzal, René Toribio, Silvina Mangano, The role of the co-chaperone HOP in plant homeostasis during development and stress, Journal of Experimental Botany, Volume 75, Issue 14, 23 July 2024, Pages 4274–4286, https://doi.org/10.1093/jxb/erae013
- Share Icon Share
Abstract
Proteins need to acquire their native structure in order to become fully functional. In specific cases, the active conformation is obtained spontaneously; nevertheless, many proteins need the assistance of chaperones and co-chaperones to be properly folded. These proteins help to maintain protein homeostasis under control conditions and under different stresses. HOP (HSP70–HSP90 organizing protein) is a highly conserved family of co-chaperones that assist HSP70 and HSP90 in the folding of specific proteins. In the last few years, findings in mammals and yeast have revealed novel functions of HOP and re-defined the role of HOP in protein folding. Here, we provide an overview of the most important aspects of HOP regulation and function in other eukaryotes and analyse whether these aspects are conserved in plants. In addition, we highlight the HOP clients described in plants and the role of HOP in plant development and stress response.
Introduction
Proteins perform a wide range of functions in the cell; therefore, maintaining a healthy proteome is fundamental for all living organisms. Their activity and stability highly depend on the protein structure (Moran Luengo et al., 2018, 2019). Some proteins acquire their native structure by spontaneous folding; however, others need to be folded by chaperones to acquire their functional state (Moran Luengo et al., 2018, 2019).
Heat shock protein 90 (HSP90) is a main chaperone that, in mammals, promotes the specific folding of >200 signalling proteins. These proteins, known as HSP90 clients, are involved in a wide range of physiological processes, including cell cycle progression, signal transduction, and transcriptional regulation (Verma et al., 2016; Kravats et al., 2018). To promote the folding of these clients, HSP90 cooperates with the chaperone HSP70 and with different co-chaperones in the known HSP70–HSP90 cycle. In this cycle, the client proteins are recruited by HSP70 and are folded by HSP90, while the co-chaperones bind to HSP70, HSP90, or both to regulate different aspects of HSP70 or HSP90 function.
The cohort of HSP70 and HSP90 co-chaperones in eukaryotes is quite diverse. Some of these proteins are considered core co-chaperones of the HSP70–HSP90 cycle, since they are required for the folding of the general bulk of the HSP90 clients. This is the case of p23, which stabilizes HSP90’s closed conformation and inhibits its ATPase activity. In addition, HSP40, a HSP70 co-chaperone, could also be included in this category. This specific co-chaperone promotes the ATP hydrolysis by HSP70 and stabilizes the binding of this chaperone to its clients (Hernandez et al., 2002; Qiu et al., 2006). In contrast, other co-chaperones do not modulate general folding through the cycle; instead, they seem to account for specific chaperone functions or for special requirements for client identification and/or folding (Moran Luengo et al., 2019; Dean and Johnson, 2021). Among them, the co-chaperones containing TPR domains are of particular interest. These co-chaperones usually interact through their TPR domains with the C-terminal EEVD sequence of the HSP70 or HSP90 chaperones. Mammalian TPR co-chaperones include, among others, different proteins from the family of the FK506-binding proteins (FKBPs) and the co-chaperones CYP40, PP5, TPR2, TTC4, the suppressor of G2 allele of Skp1 (SGT1), the C-terminus of HSP70-interacting protein (CHIP), and the HSP70–HSP90 organizing protein (HOP) (Smith, 2004; Li et al., 2012).
Orthologs of the cited HSP90 co-chaperones have also been identified in plants (di Donato and Geisler, 2019). Interestingly, despite their high conservation, some plant co-chaperones show a particular mechanism of action compared with other eukaryotes. Remarkably, in contrast to its mammalian and yeast counterparts, wheat p23 does not seem to inhibit the ATPase activity of HSP90 in wheat germ extracts (Zhang et al., 2010). Moreover, different HSP90 co-chaperones have been shown to be involved in plant-specific processes. These processes include the maintenance of the auxin gradient in roots, the control of stem cell homeostasis in the shoot apical and floral meristems (D’Alessandro et al., 2015; Prunet et al., 2015), or the regulation of the stability and activity of proteins related to photosynthesis and abscisic acid signalling (di Donato and Geisler, 2019; Zhang et al., 2021).
Here, we focus on the family of HOPs. HOPs are TPR-domain containing co-chaperones, which bind simultaneously to HSP70 and HSP90 and facilitate the transfer of specific HSP90 clients from the chaperone HSP70 to HSP90 (Johnson et al., 1998; Odunuga et al., 2004; Lee et al., 2012; Baindur-Hudson et al., 2015; Toribio et al., 2020; Bhattacharya and Picard, 2021). HOPs are highly conserved in all eukaryotes; however, due to the relevance of HOP clients to mammalian development, the function of HOP and its involvement in the HSP70–HSP90 cycle have been studied in depth in mammals. Interestingly, recent advances in the study of the role of HOPs in plants have revealed that these co-chaperones display specific clients (different from those described in mammals and yeast), and participate in special processes (some of them plant specific). This makes the study of the roles of HOP in plant species especially interesting.
In this review, we give an overview of the most important advances in HOP function in eukaryotes. In addition, we analyse the common features and the diverging aspects of HOP’s function and regulation in non-plant and plant species. Finally, we gather the information about the possible clients of HOPs in plants and describe the most recent advances that qualify plant HOP co-chaperones as master assistants of the stress response and hormonal pathways.
The HOP family
STI1, the yeast ortholog of human HOP, was initially identified in a genetic screen for proteins involved in heat shock response (Nicolet and Craig, 1989). Since then, HOP orthologs have been identified in all analysed eukaryotic species, including 53 species of plants. This high conservation in eukaryotes suggests that its evolutionary origin dates back to the emergence of the first eukaryotic cells (Salinas Castellanos et al., 2015).
In some species, the HOP family has only one single member, as occurs in human or yeast (Nicolet and Craig, 1989; Honore et al., 1992) (Fig. 1). Nevertheless, based on the information displayed in the Arabidopsis database (www.arabidopsis.org), which should be verified species by species, the number of HOP genes in each plant species varies from one to seven (as predicted for Solanum lycopersicum and Triticum aestivum, respectively). This suggests that the number of genes that belong to the HOP family in each plant species is usually higher than in other eukaryotes. This seems to be derived from a diversification that is not observed in other non-plant eukaryotes and that does not seem to have occurred at the beginning of plant evolution (Fig. 1). In fact, there is just one single HOP member in basal flowering plants, such as Amborella. Furthermore, HOPs from phylogenetically distant plant species do not group together. Instead, it seems that there is a diversification into two groups that evolved independently in different plant taxa. This can be observed in the case of the families with more represented members, such as Poaceae or Solanaceae (Fig. 1).
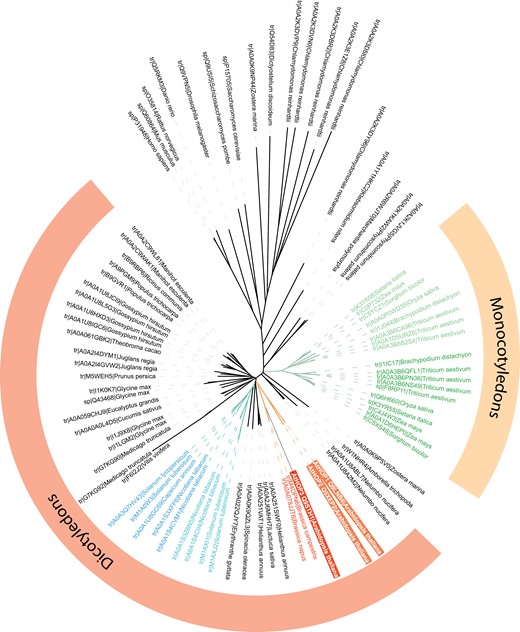
Phylogenetic tree of the HOPs from the main non-plant model eukaryotes and plants. Those proteins described as HOP plant orthologs in the TAIR database (www.arabidopsis.org) are included. Protein identification and species names are shown. The observed diversification into two groups in different plant taxa is highlighted by light and dark green colours in the case of Poaceae and light and dark blue colours in the case of Solanaceae. Arabidopsis HOPs are highlighted by red boxes. The amino acid sequences were aligned by CLUSTAL W, and the phylogenetic tree was constructed by MEGA 4.0 using the Neighbor–Joining method.
Among plants, the most studied family of HOP co-chaperones is that from Arabidopsis. This family encompasses three members: HOP1, HOP2, and HOP3 (Fernandez-Bautista et al., 2018; Toribio et al., 2020). These genes code for polypeptides of 572, 571, and 558 amino acids, respectively, and share a high degree of amino acid identity (HOP1 versus HOP2 83.10%; HOP1 versus HOP3 75.09%; HOP2 versus HOP3 74.91%) (Toribio et al., 2020).
Why does the HOP family present a larger number of genes in plant species compared with other eukaryotes such as mammals or yeast? Plants are sessile organisms and, therefore, they have evolved quite sophisticated mechanisms to cope with the environmental insults to which they are exposed. Many adverse conditions affect water/solute availability, and this availability impacts on thermodynamics of the molecules, disturbing protein folding and stability (Gidalevitz et al., 2011; Izumi, 2019). HOPs assist protein folding, and, therefore, the complexity of the HOP family in plants could be one sophisticated mechanism to ensure the folding of relevant proteins under control conditions, but also during stress responses. Remarkably, expression analyses have pointed out that while AtHOP1 and AtHOP2 seem to have a constitutive expression, AtHOP3 is highly induced by heat and moderately by endoplasmic reticulum (ER) stress. This differential expression points to a prevalent role for this specific member in response to proteotoxic stresses in Arabidopsis (Fernandez-Bautista et al., 2017, 2018). This observation highlights the interest in conducting comprehensive studies on the expression of the different HOP genes in those plant species that have a multigene family. This may help to understand the precise role of each member within the family and to evaluate whether, in general, gene family complexity fine-tunes HOP gene expression in response to different stresses in plants.
HOP structure
HOPs are structurally characterized by the presence of nine TPR motifs that cluster into three TPR domains (TPR1, TPR2A, and TPR2B), and two aspartate–proline-rich polypeptides (DPs): DP1 and DP2. Within HOP, these domains are ordered, from the N- to the C-terminal part of the molecule, following the pattern: TPR1, DP1, TPR2A, TPR2B, and DP2 (Schmid et al., 2012) (Fig. 2A). Interestingly, this domain organization seems to be evolutionarily derived from duplications of sequences coding for the TPR–DP modules through exon shuffling (Hernandez Torres et al., 2009; Salinas Castellanos et al., 2015). Despite the overall domain organization of HOP being conserved in most eukaryotes, including ~50 different plant species (Salinas Castellanos et al., 2015), there are some exceptions; for example, Caenorhabditis elegans HOP lacks the TPR1 and DP1 domains (Song et al., 2009).
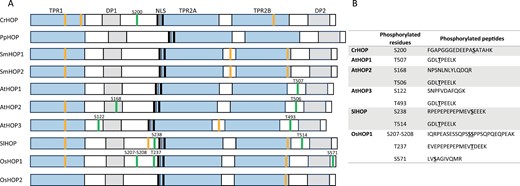
Structural domains and putative regulatory motifs found in HOPs from plant species belonging to different evolutionary groups. (A) Schematic representation of the structural domains, phosphorylation sites, and predicted SUMOylated motifs. Protein sequences were retrieved from UniProt and NCBI: Chlamydomonas reinhardtii (Cr; A0A2K3DY96), Physcomitrium patens (Pp; A0A2K1KAW2), Selaginella moellendorffii (Sm; XP_024543289.1, XP_024541869.1), Arabidopsis thaliana (At; Q9LNB6, Q5XEP2, Q9STH1), Solanum lycopersicum (Sl; A0A3Q7HV43), Oryza sativa (Os; Q0DZ61, Q0JBE4). Phosphorylation sites are marked as green lines. These sites were obtained from experimental data included in the Plant PTM viewer (https://www.psb.ugent.be/webtools/ptm-viewer/index.php). The positions of the predicted SUMOylated motifs (analysed through https://www.abcepta.com/sumoplot, score >0.9) are marked as orange lines. (B) List of phosphorylated residues and peptides shown in (A).
Predictions of HOP structure date back to its discovery. In the initial studies, the TPR domains were proposed to arrange into α-helices, while the DP domains were considered to be unstructured (Nicolet and Craig, 1989). Subsequent X-ray diffraction and solution NMR analyses of the structures of human and yeast HOP/STI1 proteins confirmed the highly organized α-helical structure of the TPR domains and revealed the presence of multiple α-helical folds in the DP1 and DP2 domains (Scheufler et al., 2000; Southworth and Agard, 2011; Schmid et al., 2012).
HOP was described early on to be in a complex with HSP70 and HSP90 in lysates from different eukaryotes, including plants, and proposed to be a central organizing component of the HSP70 and HSP90 complex (Smith et al., 1993; Chen et al., 1996). The domains involved in HOP interaction with HSP70 and HSP90 soon started to be characterized. In this regard, different studies revealed that the TPR1 and TPR2B domains contain binding sites for HSP70, while the TPR2A and TPR2B domains are involved in HSP90 binding. Furthermore, these studies also reported the important role of the C-terminal EEVD domains of HSP70 and HSP90 in these interactions (Chen et al., 1996; Scheufler et al., 2000; Van Der Spuy et al., 2000).
Interestingly, different functional and structural studies support that the essential domains in the molecule are TPR2A, TPR2B, and DP2. In this scenario, the TPR1 and DP1 domains seem to play an auxiliary role (Flom et al., 2006; Schmid et al., 2012). Quite recently, the TPR1 domain has been shown to be involved in the re-arrangement of the HSP90–client complex and as an exit site for HSP70 once the client protein has been transferred to HSP90 (Dahiya et al., 2022; Wang et al., 2022). Despite these observations, the function of both domains is still a matter of debate.
The role of HOP in the HSP70–HSP90 cycle
HOP is an important co-chaperone of the HSP70–HSP90 folding cycle. As stated above, in mammals, HOP promotes the folding of a specific set of HSP90 clients, including the glucocorticoid receptor (GR). Interestingly, although there seem to be other HSP90 client proteins assisted by HOP, the GR has become a reference model for client folding through the HSP70–HSP90 cycle (Lorenz et al., 2014). This is partly due to the possibility of carrying out in vitro GR reconstitution and ligand binding experiments using purified proteins. Early reconstitution analyses allowed the description of GR activation requiring the participation of the chaperones HSP70 and HSP90 and of the co-chaperones HSP40, HOP, and p23 (Lorenz et al., 2014). In addition, other analyses based on X-ray diffraction or NMR have been fundamental to obtaining clearer information of the dynamics, stoichiometry, and the action mechanisms of the different complexes formed during client folding (Scheufler et al., 2000; Kajander et al., 2009; Schmid et al., 2012; Darby et al., 2020). These studies allowed a global picture of HOP function in the transfer of client proteins between the chaperones in the HSP70–HSP90 cycle to be obtained. However, as discussed below, there are still specific aspects of HOP function that remain unresolved.
Gathering the information, mainly obtained from the GR model, the folding of the client proteins by the HSP70–HSP90 cycle could be divided into three different steps as described below (Fig. 3A),
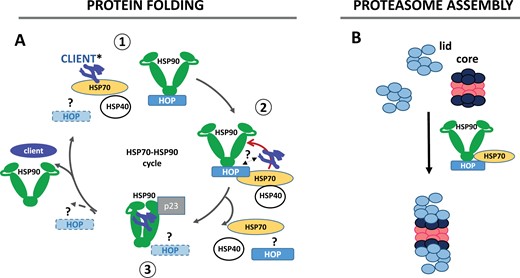
Proposed functions of HOP in non-plant eukaryotes. (A) Model of client folding through the HOP-dependent HSP70–HSP90 cycle. This model could be schematically divided into three different steps: (1) binding of HSP70 to the client and formation of the HOP–HSP90 complex; (2) enrolling the client protein to the HOP–HSP90 complex; and (3) reorganization of the complex and client folding by HSP90. The best known function of HOP in this folding pathway is the transfer of the client proteins from the chaperone HS70 to HSP90. Nevertheless, quite recently, other functions of HOP such as the remodelling of the client–HSP70–HSP40 complexes, the recognition of the client, and the promotion of the HSP90 close conformation have been described. Since these functions are still under study, they appear in the model in lighter colour and with question marks. (*) Based on recent information obtained from hop mutants in mammals and yeast, HOP may assist the HS70–HSP90 cycle to promote the folding of a highly specific set of HSP90 clients, while other HSP90 clients could be folded by a HSP70–HSP90 cycle lacking HOP. (B) HOP has also been involved in the assembly of the lid and core particles of the proteasome. Neither the existence of a binary complex nor the involvement of HOP in proteasome assembly has been explored in plants.
Binding of HSP70 to the client and formation of the HOP–HSP90 complex
To enter the HSP70–HSP90 cycle, the client protein binds to HSP70 in the presence of the co-chaperone HSP40. On the other hand, HOP interacts through the TPR2A module with the ADP-bound form of HSP90, forming a complex that most probably consists of two molecules of HSP90 and a single molecule of HOP (Scheufler et al., 2000; Brinker et al., 2002; Southworth and Agard, 2011; Alvira et al., 2014). Following this binding, which acts as docking, interactions of HOP with HSP90 extend to other parts of the HSP90 dimer (Southworth and Agard, 2011; Lee et al., 2012; Alvira et al., 2014; Darby et al., 2020). These interactions seem to produce a conformational change in the HSP90 dimer that facilitates the binding of the HSP70–client complex, while preventing the hydrolysis of ATP (Southworth and Agard, 2011; Lee et al., 2012; Darby et al., 2020).
Enrolling the client protein in the HOP–HSP90 complex.
In the context of the HSP70–HSP90 cycle, HSP90 is the chaperone in charge of most aspects of client remodelling (Moran Luengo et al., 2019). Consequently, the client protein should be handed over from HSP70 to HSP90 to be properly folded. In mammals, in the case of a selective set of HSP90 clients, such as the GR, this process is mediated by HOP. For this, HOP interacts simultaneously with HSP70 and HSP90, serving as a scaffold that allows the transfer of the client protein from HSP70 to the HSP90 chaperone (Wang et al., 2022).
Reorganization of the complex and client folding by HSP90
Once the client has bound to HSP90, the subsequent steps in the cycle are the binding of ATP and p23, the release of HOP and HSP70, and the acquisition of the HSP90–client closed conformation, which serves as a driving force for client remodelling. Finally, ATP hydrolysis by HSP90 returns HSP90 to the open conformation, releasing the client protein, and gaining competence to start a new folding cycle through the interaction with HOP (Lotz et al., 2003).
Despite the role of HOP as a physical connector of the chaperone machineries being out of the question, there are still multiple aspects of the transfer process that are under debate. Quite recently, two important articles have proposed unexpected functions for HOP in client loading and cycle progression (Dahiya et al., 2022; Wang et al., 2022). Indeed, it has been recently reported that, apart from its transfer function, HOP may also be involved in (i) remodelling the client–HSP40–HSP70 complexes (Dahiya et al., 2022); (ii) the recognition of the client protein through the DP2 domain (Wang et al., 2022); and (iii) the promotion, in complex with the co-chaperone p23, of the HSP90 closed conformation (Dahiya et al., 2022). Finally, in contrast to generalized knowledge, which suggests that just one single HSP70 molecule interacts with the HSP90–HOP complex, recent analyses indicate that HSP90–HOP complexes may interact with two different molecules of HSP70, one (called HSP70C) that binds to and brings the client protein to the HSP90–HOP complex, and another (called HSP70S) that scaffolds the whole complex and allows the HSP90 dimers to adopt a semi-closed conformation (Wang et al., 2022). These articles have clearly provided highly interesting data, but have also questioned specific aspects of the current models, such as how HOP enters the cycle or when HOP is released. In our opinion, the emergence of these new studies reflects the high interest in the field for fully understanding the molecular role of HOP. Nevertheless, it would be important to verify these recent data through alternative techniques. This will allow assembly of the correct pieces of the puzzle into a more comprehensive model of the HOP-dependent HSP70–HSP90 cycle.
As stated above, most of the information about the role of HOP has been obtained in the mammalian system using the GR as a client. Based on the conservation of the domains involved in HOP interactions with HSP70 and HSP90 in the HOPs in plants, it is tempting to speculate that probably most of the model of the mammalian HSP70–HSP90 cycle could be transferred to plants. However, for this, it is important that the GR folding model, on which much work has been done, is perfectly defined and that this knowledge is accompanied by a better understanding of the structure and characteristics of plant HOPs. This will allow specific aspects of the mammalian model to be adapted to the special characteristics of HOPs in plants. Currently, a relevant question is whether the interaction of DP2 with the client proteins is maintained in plants, since, based on current information, HOP clients in plants differ from mammalian GR in sequence, structure, and function.
HOP and its relationship with other co-chaperones: compete or cooperate?
The above description of the HSP70–HSP90 cycle was given in order to highlight the molecular function of HOP. Nevertheless, as mentioned in the Introduction, it should be considered that there are other co-chaperones that also assist the HSP70–HSP90 cycle and whose loading in the cycle could preclude the binding of HOP. Indeed, a competition between HOP and other co-chaperones has been described for FKBPs, CYP40, or AHA1 in mammals (Owens-Grillo et al., 1996; Harst et al., 2005; Ebong et al., 2016). This aspect is especially important in the case of the TPR-containing co-chaperones, since these co-chaperones share with HOP the TPR domains and bind, like HOP, to similar positions of the chaperones HSP70 and HSP90. Of special relevance is the mutually exclusive relationship of the co-chaperones HOP and CHIP (Edkins, 2015). Interestingly, in addition to folding, HSP70–HSP90 complexes also promote protein degradation, a process that is mediated by CHIP (Kundrat and Regan, 2010). CHIP is a TPR-containing co-chaperone with E3 ubiquitin ligase activity, whose loading in the HSP70–HSP90 cycle reduces HOP recruitment to the HSP70–HSP90 complex (Ballinger et al., 1999). Based on these data, it has been speculated that, in mammals, the presence of HOP in the HSP70–HSP90 complexes commits the client proteins to folding, while the presence of CHIP leads them to degradation (Marques et al., 2006; Edkins, 2015). As stated in the Introduction, the family of FKBP co-chaperones and CHIP are conserved in plants. However, direct competition between these TPR co-chaperones and HOP has not been fully addressed in these specific eukaryotes.
SGT1 is also a TPR-containing co-chaperone that, as HOP does, binds to HSP90 in its open conformation. Apart from the TPR domain, SGT1 contains a central CHORD (CS) domain and a C-terminal SGT-specific (SGS) domain. Interestingly, despite SGT1 proteins having the TPR domain, multiple data have demonstrated that SGT1 does not use its TPR domain to interact with the C-terminal end of the chaperone HSP90; instead, SGT1 binds through its CS domain to the N-terminal part of HSP90. This distinct binding could avoid direct competition with HOP, opening up the possibility that HOP and SGT1 could form part of a complex with HSP90 (Zhang et al., 2008). Interestingly, formation of a human HOP–HSP90–SGT1 ternary complex was previously described in vitro (Catlett and Kaplan, 2006); however, no common targets of HOP and SGT1 were described in mammals or yeast, which in our opinion could explain the lack of interest in the formation of the HOP–HSP90–SGT1 complexes in these eukaryotes.
In Arabidopsis, there are two members of the SGT1 family, SGT1a and SGT1b. SGT1b has been better characterized and shows important roles in defence and hormonal signalling (Austin et al., 2002; Azevedo et al., 2002, 2006; Boter et al., 2007; Zhang et al., 2015). It is noteworthy that, as described below, Oryza sativa HOPs and AtHOPs have also been shown to be involved in these specific processes, although no direct link between HOP and SGT1b became evident for a long time in plants (Azevedo et al., 2002, 2006; Takahashi et al., 2003; Miya et al., 2007; Chen et al., 2010). Quite recently, TIR1, a known client of SGT1b, has also been proposed as client of HOP in Arabidopsis. Consistently, TIR1 accumulation is reduced in the hop triple mutant and in the sgt1b single mutant, and these mutants show a phenotype of reduced sensitivity to auxin in terms of inhibition of root growth and promotion of lateral root formation. Interestingly, this phenotype is enhanced in the quadruple hop1 hop2 hop3 sgt1b mutants (Muñoz et al., 2022). This seems to suggest a possible cooperation of both co-chaperones in TIR1 folding and auxin signalling. Whether HOPs, in cooperation with SGT1b, assist the folding of other clients in plants is under investigation. Interestingly, in addition to TIR1, a different known target of SGT1b that could also be a possible client of HOP is COI1 (Muñoz et al., 2021), although in this case further experiments that demonstrate the direct effect of HOP over COI1 folding or stability are lacking. In this context, it would also be important to investigate whether HOP and SGT1b have specific clients of each co-chaperone while helping cooperatively in the folding of other specific proteins. Probably, this investigation would benefit from studies that allow the parallel identification of HOP and SGT1 interactors. All this work would shed light on the roles of HOP and SGT1 in plants. Remarkably, given the high conservation of HOP and SGT1 structures and of their binding to HSP90, it is very likely that HOP and SGT1 could also cooperate in the folding of common clients in other eukaryotes. This line of research could highly enrich our knowledge on the HSP70–HSP90 folding pathway.
HOP regulation: localization and post-translational modifications
In non-plant eukaryotes, HOP mainly accumulates in the cytosol, although it is also localized in the nucleus, the Golgi apparatus, the cell membrane, and the extracellular fraction (Bhattacharya and Picard, 2021). Interestingly, it has been described that murine HOP (mHOP) shuttles from the cytoplasm to the nucleus under different conditions, including heat stress (Longshaw et al., 2004; Daniel et al., 2008). Remarkably, this change in subcellular localization is dependent on the presence on HOP of a bipartite nuclear localization signal (NLS) and, specifically, on a short lysine arm (KK, amino acids 222–223). Interestingly, this transport is regulated by phosphorylation. Indeed, phosphorylation of the S189 residue by casein kinase II promotes HOP nuclear localization, while phosphorylation of the T198 residue by cdc2 enhances its cytoplasmic retention (Longshaw et al., 2000, 2004). In addition, in mammalian astrocytes, the nuclear localization of HOP was shown to be favored by its interaction with the E3 SUMO ligase PIAS1, which also promotes its SUMOylation (Soares et al., 2013).
HOPs have also been localized in multiple cellular compartments in plants. Certainly, the three members of the Arabidopsis HOP family seem to be mainly accumulated in the cytosol under non-stress conditions, although they are also present in the nucleus (Fernandez-Bautista et al., 2018). In addition, AtHOP3 was shown to co-localize with ER markers and to interact with the ER-resident protein BiP, which strongly suggests that part of HOP3 also accumulates in the ER (Fernandez-Bautista et al., 2017). The subcellular localization of HOPs has also been analysed under different stress conditions in plants. OsHOP was observed to be present in the ER and plasma membrane in a complex with CERK1 and RAC1 during pathogen-associated molecular pattern (PAMP)-triggered innate immunity (Chen et al., 2010). Moreover, HOP was localized in virus-induced membrane aggregates during potato virus Y (PVY) infection in tobacco (Lamm et al., 2017). Interestingly, as is also the case for mHOP (Daniel et al., 2008), the three members of the Arabidopsis HOP family shuttle from the cytoplasm to the nucleus in response to heat (Fernandez-Bautista et al., 2018).
As in the case of other eukaryotes, HOPs are also subjected to different post-transcriptional modifications (PTMs) in plants. However, whether these PTMs regulate their subcellular localization is not so clear. Interestingly, CrHOP and OsHOP1a are phosphorylated at S200 and S208, respectively (Fig. 2). These residues are located, as in the case of the T198 in mHOP, near the short arm of a bipartite NLS (Fig. 2A). This makes it possible that the phosphorylation of these residues could regulate, as happens with mHOP, their subcellular localization. Unfortunately, the region where these phosphorylation sites are found is not highly conserved among plant species. This lack of conservation makes it especially difficult to predict if this phosphorylation and/or its regulatory role will be conserved in the plant lineage.
In addition to these phosphorylation events, other phosphorylation sites have been described in quite conserved regions of specific plant HOPs. This is the case of the S168 residue in AtHOP2, which is located at the DP1domain, or the S571 residue, which is present at the C-terminal end of the molecule in OsHOP1 (Fig. 2). Interestingly, the three members of the AtHOP family are phosphorylated at the T507 residue (referred to HOP1) located within the TPR2B–DP2 domain (Fig. 2). This phosphorylation site is also conserved in SlHOP (T514; Fig. 2). Remarkably, despite these residues being located in important motifs/modules of HOP function, there is no available information regarding the potential significance of these phosphorylation events. In this sense, greater and more systematic efforts are needed to unravel different aspects of HOP phosphorylation, such as the conservation of the phosphorylation sites, the conditions that promote this post-translational modification, and the role and relevance of the different events for HOP structure and function.
In addition to phosphorylation, it is possible that HOPs are modified by SUMOylation in plants. Interestingly, primary sequence analyses predict the existence of conserved SUMOylation motifs (ΨKXE/D, where Ψ=hydrophobic, X=any amino acid) throughout the plant lineage (Fig. 2A). Based on these predictions, AtHOP2 and AtHOP3 (but not AtHOP1) are likely to be SUMOylated. Since specific members of the Arabidopsis HOP family are known to play prevalent roles over the others in different processes (see below), it would be interesting to analyse whether HOPs are selectively SUMOylated under different environmental challenges. SUMOylation has a plethora of effects (Augustine and Vierstra, 2018). This makes it experimentally challenging to undertake a thorough investigation into the role of SUMOylation on Arabidopsis HOPs. However, given the fact that some SUMOylation sites are located within the TPR1 and TPR2B modules, and these domains are involved in HSP70 interaction, the study of the relevance of these putative SUMOylation sites in HSP70 binding could be a starting point in this study.
HOP function in the folding of specific HSP90 clients and in proteasome assembly
HOP was initially considered a core co-chaperone of HSP90 and, based on this, it was speculated that HOP may participate in the folding of the majority of HSP90 clients. However, different studies in mammals and yeast have recently established that, in contrast to this general assumption, the folding of the vast majority of the HSP90 clients in these specific eukaryotes may be driven by a HSP70–HSP90 binary complex lacking HOP (Bhattacharya et al., 2020). Interestingly, the existence of such a complex has been confirmed in hop/sti1 mutant cells in the two cited model systems. This complex seems able to maintain the accumulation of a large part of the HSP90 client proteins in hop knockout (KO) cells and has been proposed to be more efficient in the folding of the general bulk of the HSP90 clients than the complex containing HOP (Xu et al., 2019; Bhattacharya et al., 2020). Notably, there are some specific HSP90 clients, such as GR and v-Src, whose accumulation is reduced when overexpressed in the absence of HOP (Bhattacharya et al., 2020). These data suggest that, although HOP may be dispensable for the folding of the general bulk of the HSP90 targets in mammals and yeast, HOP function could be essential for the folding of specific proteins, which make up the specific set of HOP targets. Unfortunately, the client determinants involved in HOP recognition or the special advantages that impose the HOP-dependent folding compared with the binary complex are currently unknown. Moreover, direct evidence of the existence of such complex in the presence of HOP is currently lacking in eukaryotes (Kravats et al., 2018; Bhattacharya et al., 2020).
In addition, HOP has been recently proposed to participate in the assembly of the lid and core particles of the proteasome in non-plant eukaryotes (Bhattacharya et al., 2020; Bhattacharya and Picard, 2021) (Fig. 3B). In accordance with this role, a reduced accumulation of fully assembled (capped) proteasome particles is observed in mammalian hop cells and sti1 mutants (Bhattacharya and Picard, 2021). Surprisingly, these defects in proteasome assembly do not render mammalian KO cells hypersensitive to proteotoxic stresses, such as those produced by thapsigargin, DTT, heat shock, or the proline analog azetidine-2-carboxylic acid (AZC), since these defects seem compensated by the increased folding capacity of the binary complex lacking HOP (Bhattacharya et al., 2020).
The existence of a HOP-independent HSP70–HSP90 complex able to maintain the folding of most of the HSP90 interactome has not been investigated either in hop mutant or in wild-type plants. In this sense, it is worth mentioning that, although recent studies demonstrate that HOPs are required to maintain the protein levels and the activity of specific HSP90 targets involved in hormonal signalling in Arabidopsis, systematic studies to identify HSP90 clients are missing in plants. This makes it especially difficult to analyse the effect of HOP or its absence in the general bulk of HSP90 clients.
Due to the high conservation of the HOP structure in eukaryotes, it is possible that the function of HOP in general processes (such as proteasome assembly or folding) could be conserved in all species. Nevertheless, direct extrapolations in this regard should be avoided, since obvious differences in the phenotypical analyses of the hop mutants are observed in plants compared with other eukaryotes. For example, as stated before, human hop KO cells are not hypersensitive to agents causing accumulation of misfolded proteins (Bhattacharya et al., 2020; Bhattacharya and Picard, 2021). In contrast, different Arabidopsis hop mutants are clearly sensitive to DTT, tunicamycin, and heat stress (Fernandez-Bautista et al., 2017, 2018). This makes the analyses of whether these proteotoxic defects of the hop mutant plants are due to alterations in the assembly of the proteasomal subunits or to the reduced folding and accumulation of specific sets of proteins especially relevant. In addition, it would be highly interesting to study the existence of a binary complex in plants and, if present, to understand why the complex, in contrast to the situation in mammals, is unable to compensate the proteotoxic defects in the model plant.
HOP proteins: master assistants of hormonal signaling and response to stress in plants
In comparison with the amount of information in other organisms, knowledge about HOP clients in plants is quite limited. Nonetheless, quite recently the F-box proteins COI1 [involved in jasmonic acid (JA) signalling], TIR1 [involved in auxin signalling], and SNE [involved in gibberellin (GA) signalling] have been proposed as possible clients of HOP in Arabidopsis (Muñoz et al., 2021, 2022; Mangano et al., 2023). In accordance with this, HOP3 interacts with COI1, and COI1 activity is reduced in the hop3-1 mutant. In a similar way, it has been reported that members of the Arabidopsis HOP family interact with TIR1 and SNE, and the accumulation of these two F-box proteins is highly reduced in the hop1 hop2 hop3 triple mutant. Consistently, the corresponding hop mutants show a reduced sensitivity to JA, auxins, and GAs which impinges on alterations of physiological processes governed by these hormonal pathways. In this sense, in agreement with the reduced activity of COI1, the hop3 mutant displays defects related to the JA network (such as a reduced defense against the acarus Tetranychus urticae and the necrotrophic fungus Botrytis cinerea). In addition, according to the reduced accumulation of TIR1, the hop1 hop2 hop3 mutant shows defects associated with the auxin pathway (such as a reduced rate of lateral root production). Moreover, most probably due to the reduced accumulation of SNE, the hop triple mutant shows defects related to the GA pathway (including a delay in seed germination and bolting) (Muñoz et al., 2021, 2022; Mangano et al., 2023). Interestingly, Arabidopsis hop mutants also show a reduced hypocotyl elongation in response to moderate increases of temperature, a process that depends on multiple hormonal pathways, including auxin, GAs, or brassinosteroids (BRs), and specific transcription factors (Quint et al., 2016).
Remarkably, TIR1 and SNE are not the only F-box proteins involved in the signalling of auxin and GAs, respectively. Certainly, auxin signalling also depends on the F-box AFB proteins, including AFB2, which shows a similar structure to TIR1 (Dharmasiri et al., 2005). A similar situation is observed in the case of GAs, whose signalling depends on the F-box proteins SNE and SLY1 (Ariizumi et al., 2011). For this reason, one fascinating aspect regarding these HOP clients is that, while TIR1 and SNE have been identified as possible targets of HOPs in Arabidopsis, the folding of AFB2 and SLY1 does not seem to have a clear dependence on HOP function. This points out, as observed for HSP90 in other eukaryotes, that HOP proteins are also highly selective in the choice of clients in plants (Boczek et al., 2015). Interestingly, HOPs have also been shown to be involved in BR signalling under salt stress (Fig. 4). In this specific case, HOPs seem to form a complex with HSP90 and the BR-related kinase BIN2. This complex facilitates the BIN2 nucleocytoplasmic transport and, therefore, the boost of BR synthesis needed to promote growth under high salt conditions (Zhang et al., 2022). Unexpectedly, despite BR being involved in multiple developmental processes, no obvious phenotypes related to BR defects were observed in the hop mutants under control conditions (Zhang et al., 2022). This observation suggests that, in contrast to the role of HOP in the auxin and GA pathway, which impinges not only on plant response to moderate temperatures but also on development (Muñoz et al., 2022; Mangano et al., 2023), the role of HOP in the BR pathway could be circumscribed to stress.
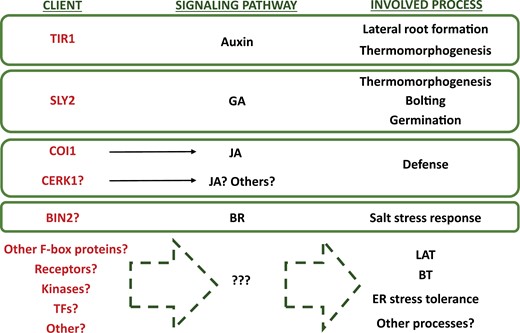
Possible clients and roles of HOP in plants. HOPs are involved in multiple developmental and stress responses in plants. In some cases, particular clients of HOP have been identified and, among them, the signalling pathways in which HOPs participate. In other cases, the involvement of HOPs in certain physiological processes has been described; however, we still lack essential information about the molecular role of HOP in these processes. Based on the information gathered from the hop mutants, it is quite possible that new HOP clients and functions will be discovered in plants. At this stage, it should not be ruled out that there could be different HOP clients modulating the output of a specific pathway or, vice versa, that a single HOP client may be involved in multiple pathways. TF, transcription factor; LAT, long-term acquired thermotolerance; BT, basal thermotolerance.
The role of HOP in stress response in plants clearly exceeds the response to salt stress (Zhang et al., 2022). Actually, different members of the Arabidopsis and the wheat HOP family have been shown to be involved in acquired and basal thermotolerance, ER stress, and the thermomorphogenesis adaptative process (Fernandez-Bautista et al., 2017, 2018; Meena et al., 2020; Toribio et al., 2020; Muñoz et al., 2022; Mangano et al., 2023) (Fig. 4). In addition, HOP has been demonstrated to be involved in innate immunity in rice (Fig. 4). In this organism, HOP interacts with two immune-related proteins, the Rac/Rop small GTPase RAC1 and the pattern recognition receptor (PRR) CERK1. This interaction seems to favor the maturation and transport to the plasma membrane of the chitin receptor CERK1 and to promote the formation in the plasma membrane of a complex called the defensome (Chen et al., 2010). Finally, HOP was described as a cellular determinant of PVY symptom development in tobacco (Lamm et al., 2017).
A major challenge: the identification of the main HOP clients in plants
Even though the analysis of hop mutants is shedding light on the prominent role of HOPs in plant adaptation to different adverse conditions, we still need to understand how HOPs participate in these processes. For this, it will be important to identify the clients of HOP. Remarkably, with the exception of thermomorphogenesis [where the defects observed in the hop mutants could be due to a reduced accumulation of TIR1 and SNE (Muñoz et al., 2022; Mangano et al., 2023)], the possible clients of HOP in the other stress responses are unknown. Based on the multiple phenotypes observed in the hop mutant, it is possible to predict that TIR1 and SNE, and probably COI1, are not the only clients of HOP in plants. Furthermore, it also seems plausible to speculate that there could be other HOP clients involved in other processes different from hormonal signalling (Fig. 4).
Interestingly, a study of translation of chloroplast pre-proteins in wheat germ extract allowed the identification of HOP in high molecular weight HSP90 pre-protein complexes, suggesting that HOP along with HSP90 binds to specific chloroplast pre-proteins to maintain them in a competent state until they are translocated to the chloroplast (Fellerer et al., 2011). If these data are corroborated in vivo, these proteins will greatly increase the number of already known plant-specific HSP90 and HOP clients.
In our opinion, systematic studies that allow the identification of HSP90 and HOP clients under different conditions are needed to have a wider picture of the role of HOP co-chaperones in plants. This seems especially challenging when considering that the genome of many plant species codes for multiple HOPs and that these proteins could perform specialized functions. Remarkably, only one member of the Arabidopsis HOP family, AtHOP3, has been shown to date to be involved in ER stress and plant defense associated with the JA pathway (Fernandez-Bautista et al., 2017; Muñoz et al., 2021). In addition, it has been described that Arabidopsis HOP2 and HOP3 display a higher involvement than HOP1 in GA signalling and in GA-related processes (Mangano et al., 2023). Remarkably, Arabidopsis HOP2 and HOP3, but not HOP1, interact with SNE (or as least HOP1 does not interact with the same affinity with SNE as with HOP2 and HOP3) (Mangano et al., 2023). This selectivity for client binding may explain why HOP2 and HOP3 play a more important role in GA signalling. Although the presence of multiple HOP genes in plant species and their selectivity complicates the analysis of the role of the HOP proteins in plants, these facts make the understanding of the molecular bases of such specificity extremely interesting.
Finally, it should be noted that in non-plant eukaryotes, HOP has also been shown to be involved in functions not related to HSP90 (Gebauer et al., 1998; Odunuga et al., 2004). Hopefully, more in-depth studies on the role of HOP in plants will also decipher new HSP90-independent functions of HOP in the plant kingdom.
Conclusions
Although HOP was initially described in the 1990s, novel studies are discovering and re-defining new aspects of HOP function in eukaryotes. Despite the effort, the knowledge of HOP function in plants is still scarce. We already know that HOP is involved in the proper signalling of important hormonal networks in plants, and participates in multiple processes associated with plant development and response to stress. Nevertheless, this seems to be only the tip of the iceberg, since, based on the existence of multiple phenotypes in the hop mutants, it is highly probable that HOP could be involved in the folding and maturation of many other clients, some of them plant specific. Furthermore, very little is known about HOP regulation in plants or the exact impact of HOP in plant protein folding and degradation. These studies, along with the identification of HOP’s clients under different conditions, will help to understand the role of these important co-chaperones in plants.
Acknowledgements
Dedicated to MMC’s beloved father, Alfonso Castellano. RIP.
Author contributions
MMC and AM: contributed equally to the majority of the writting and discussion; ENU and ICO: contributed equally to gathering information on post-transcriptional regulation and localization, and generated the related figures; RT and SM: equally supervised the writing and the discussion, and generated most figures.
Conflict of interest
The authors declare no conflict of interest.
Funding
This work is supported by the projects PID2021-126956OB-I00 from Ministerio de Ciencia, Innovación y Universidades (MICIU), 2020-T1/BIO19900 from Comunidad de Madrid (CAM), the ‘Severo Ochoa Programme for Centers of Excellence in R&D’ from the Agencia Estatal de Investigación of Spain (grant CEX2020-000999-S to the CBGP), and the CEPEI Program (grant SEV-2016-0672). In the frame of these programs, ENU is a recipient of an ‘Atracción de Talento’ contract, and RT, SM, and IO were supported with post-doctoral or pre-doctoral contracts.
Data availability
This review contains no experimental data.
References
Author notes
M. Mar Castellano and Alfonso Muñoz These authors contributed equally to this work.
Isabel C. Okeke and Esther Novo-Uzal These authors contributed equally to this work.
René Toribio and Silvina Mangano These authors contributed equally to this work.
Comments