-
PDF
- Split View
-
Views
-
Cite
Cite
Hao Li, Yajuan Ou, Kui Huang, Zhongming Zhang, Yangrong Cao, Hui Zhu, A pathogenesis-related protein, PRP1, negatively regulates root nodule symbiosis in Lotus japonicus, Journal of Experimental Botany, Volume 75, Issue 11, 7 June 2024, Pages 3542–3556, https://doi.org/10.1093/jxb/erae103
- Share Icon Share
Abstract
The legume-rhizobium symbiosis represents a unique model within the realm of plant-microbe interactions. Unlike typical cases of pathogenic invasion, the infection of rhizobia and their residence within symbiotic cells do not elicit a noticeable immune response in plants. Nevertheless, there is still much to uncover regarding the mechanisms through which plant immunity influences rhizobial symbiosis. In this study, we identify an important player in this intricate interplay: Lotus japonicus PRP1, which serves as a positive regulator of plant immunity but also exhibits the capacity to decrease rhizobial colonization and nitrogen fixation within nodules. The PRP1 gene encodes an uncharacterized protein and is named Pathogenesis-Related Protein1, owing to its orthologue in Arabidopsis thaliana, a pathogenesis-related family protein (At1g78780). The PRP1 gene displays high expression levels in nodules compared to other tissues. We observed an increase in rhizobium infection in the L. japonicus prp1 mutants, whereas PRP1-overexpressing plants exhibited a reduction in rhizobium infection compared to control plants. Intriguingly, L. japonicus prp1 mutants produced nodules with a pinker colour compared to wild-type controls, accompanied by elevated levels of leghaemoglobin and an increased proportion of infected cells within the prp1 nodules. The transcription factor Nodule Inception (NIN) can directly bind to the PRP1 promoter, activating PRP1 gene expression. Furthermore, we found that PRP1 is a positive mediator of innate immunity in plants. In summary, our study provides clear evidence of the intricate relationship between plant immunity and symbiosis. PRP1, acting as a positive regulator of plant immunity, simultaneously exerts suppressive effects on rhizobial infection and colonization within nodules.
Introduction
In nature, most leguminous plants have evolved abilities to engage in symbiotic interactions with rhizobia (Oldroyd et al., 2011). This partnership involves the formation of a special organ known as a nodule, within which rhizobia reside and reduce nitrogen gas into ammonia for host plants (Oldroyd et al., 2011; Yang et al., 2021). The process of nitrogen reduction within nodules is remarkably efficient compared to other biological nitrogen-reduction pathways (Zhu et al., 2023). Significantly, root nodules are considered nitrogen reservoirs, providing a substantial source of nitrogen essential for plant growth and development (Oldroyd and Leyser, 2020). The intimacy of symbiotic interactions between plants and rhizobia raises an intriguing question regarding how symbiotic cells accommodate rhizobia without triggering an apparent plant immunity. Although approximately 200 genes from host plants have been characterized to be involved in regulating root nodule symbiosis (Oldroyd, 2013; Long, 2016; Roy et al., 2020; Wang et al., 2022; Yang et al., 2022), the mechanisms of plant immunity regulating rhizobial symbiosis is still largely unclear.
Haemoglobins are prevalent across all biological kingdoms and serve diverse roles, including the transport, delivery, and scavenging of oxygen (O2) and nitric oxide (NO) (Gell, 2018). In legumes, gene duplication events have given rise to symbiotic haemoglobins, known as leghaemoglobins (Lbs), which exert distinct regulatory effects in nodule symbiosis when compared to non-symbiotic haemoglobins (Hbs) (Larrainzar et al., 2020). Hbs modulate NO concentration during the early stages of symbiosis to prevent activation of the plant immune response, whereas Lbs act as O2 buffers, safeguarding nitrogenase activity within nodules (Ott et al., 2005; Larrainzar et al., 2020). In Lotus japonicus, there are three Lbs members, whereas Medicago truncatula has 12 (Larrainzar et al., 2020). Knockout of Lbs in plants demonstrated no significant differences from wild-type (WT) plants when fertilized with mineral nitrogen, underscoring the vital role of Lbs in symbiosis rather than general plant growth and development (Wang et al., 2019). Furthermore, triple mutants exhibit increased production of superoxide radicals and hydrogen peroxide, leading to premature nodule senescence (Wang et al., 2019). A specific cis-element within the Lbs promoter, the nitrate-responsive element (NRE), plays a critical role in driving high Lbs expression in nodules (Jiang et al., 2021). Knockout of this NRE element through CRISPR technology resulted in significantly reduced Lbs expression (Feng et al., 2021a; Jiang et al., 2021). The transcription factors Nodule Inception (NIN) and NIN-like protein2 (NLP2) directly activate Lbs expression through binding to the NRE motif (Feng et al., 2021a; Jiang et al., 2021). These findings underscore the critical role of Lbs in nodule symbiosis.
In response to environmental microbes, plants have evolved inducible defence systems that activate when specific receptors recognize microbial signal molecules, such as bacterial flg22 (a conserved 22 a.a. from N-terminus of flagellin) or chitin from fungal cell walls (Couto and Zipfel, 2016; DeFalco and Zipfel, 2021; Lee et al., 2021). In the case of symbiotic rhizobia, a plant’s defence system is in a passive state to establish endosymbiosis (Toth and Stacey, 2015; Cao et al., 2017; Gourion and Ratet, 2021; Berrabah et al., 2023). The introduction of purified nodulation factors (NFs) induces the expression of some defence-related genes within 1 h in L. japonicus roots, but these defence genes return to their baseline levels after 7 h of treatment (Nakagawa et al., 2011). Activation of defence responses by flg22 leads to the suppression of subsequent rhizobial infection and delays in nodule development (Lopez-Gomez et al., 2012). However, once symbiosis is established, flg22 treatment seems to have no discernible effect on the formation of new nodules (Lopez-Gomez et al., 2012). Recent research indicates that SymRK (Symbiosis Receptor-like Kinase) promotes rhizobial infection by suppressing LjBAK1 (Brassinosteroid insensitive1-Associated receptor Kinase 1)-mediated plant immunity in L. japonicus (Feng et al., 2021b). Additionally, NIN, an indispensable transcription factor for rhizobial symbiosis, has a role in suppressing defence responses in M. truncatula nodules (Liu et al., 2021). These findings suggest that symbiotic signalling takes precedence over defence systems in nodule symbiosis. Several genes in M. truncatula have been identified as essential for maintaining rhizobia in symbiotic cells. For instance, Defective in Nitrogen Fixation2 (DNF2), Cysteine-rich Receptor-like Kinase (SymCRK), Regulator of Symbiosome Differentiation (RSD), and Nodules with Activated Defense1 (NAD1) mutants all exhibit increased accumulation of phenolic compounds and induction of defence-related genes in M. truncatula, indicating their roles in preserving bacteroides by suppressing defence responses (Bourcy et al., 2013; Sinharoy et al., 2013; Berrabah et al., 2014, 2015, 2018; Wang et al., 2016; Domonkos et al., 2017). However, there is limited knowledge regarding genes in L. japonicus that suppress immunity during rhizobial maintenance within symbiotic cells.
In our current study, we identify LjPRP1, a pathogenesis-related family protein from L. japonicus, as an important regulator of both rhizobial symbiosis in nodules as well as the plant immune response. The PRP1 gene is induced by rhizobial treatment and exhibits high expression levels in nodules. Rhizobial infection and nodule nitrogen fixation are significantly enhanced in the prp1 mutants, whereas PRP1 overexpression results in a reduced number of infection threads and decreased nitrogenase activity. Corresponding to the increased pink colouration observed in nodules of the prp1 mutants, the protein levels of Lbs and the proportion of infected cells are notably elevated in prp1 nodules. Furthermore, PRP1 also positively regulates pattern-triggered immunity in plants. The prp1 mutant plants are more susceptible to both Pseudomonas syringae and Botrytis cinerea. These data strongly suggest that PRP1 serves as an important intersection point between the immune pathway and symbiotic signalling by preventing excessive rhizobial colonization within nodules.
Materials and methods
Plant materials and growth conditions
Lotus japonicus ecotypes Miyakojima MG-20 and Gifu-129 were utilized in this study. The LORE1-inserted prp1 mutant line (30037066) was acquired from Lotus Base (https://lotus.au.dk/) (Malolepszy et al., 2016; Mun et al., 2016). We generated the transgenic lines pPRP1:PRP1-GFP and pUbi-PRP1-FLAG (PRP1-OE) in L. japonicus MG20 using the stable transformation method.
Seeds were surface-sterilized for 6–8 min, rinsed four to six times with cold sterilized water, followed by a 5 min surface sterilization in a 2% sodium hypochlorite solution, and a final rinse of four to six times with sterilized water. These seeds were then synchronized at 4 °C in a dark growth room for 2 d and subsequently germinated on Murashige and Skoog (MS) medium-containing agar plates (0.8%) at 22 °C for 2 d in darkness. Young seedlings were then transferred to a growth chamber with conditions set at 22 °C, under a 16 h light/8 h dark photoperiod. These seedlings were eventually moved to pots with sterile vermiculite and perlite at a 2:1 volume ratio and supplied with nitrogen-free 1/2 Broughton and Dilworth (B&D) medium (Broughton and Dilworth, 1971). Lotus japonicus seedlings were cultivated under 16 h light/8 h dark conditions in a growth room with 200 µmol m–2 s–1 light irradiance at 22–23 °C, with a relative humidity range of 40–60%. Nicotiana benthamiana plants were grown in a growth room with 200 µmol m–2 s–1 light irradiance at 22 °C under a 16 h light/8 h dark photoperiod with 40–60% relative humidity for 3–4 weeks before conducting experiments.
Rhizobial infection and nodulation phenotyping
Lotus japonicus wild type Gifu or MG20, the prp1 mutant, and pUbi-PRP1-FLAG transgenic seedlings were transplanted to pots containing sterile vermiculite and perlite. Rhizobia strain Mesorhizobium loti MAFF303099 constitutively expressing DsRed (M. loti-DsRed) and M. loti NZP2235 harbouring pXLGD4 conferring constitutive β-galactosidase (LacZ) expression (M. loti-LacZ) were used in this study. Plants were inoculated with M. loti-DsRed or M. loti-LacZ at a final OD600 of 0.02. To visualize infection threads, plants were harvested at 5 d post-inoculation. Nodule phenotypes were evaluated at 14 or 21 d post-inoculation for nodulation assay.
Plasmid construction
To generate PRP1-OE transgenic lines, the corresponding coding sequences were PCR-amplified from wild-type cDNA and cloned into pUbi-Hyg-3×HA (Human influenza hemagglutinin, Maekawa et al., 2008) after digestion with XbaI and StuI. For PRP1 expression driven by the native promoter, the hygromycin resistance gene was excised from pCAMBIA1300 after XhoI digestion, and replaced with green fluorescent protein (GFP) to generate pCAMBIA1300-GFP. The DNA fragment containing the PRP1 promoter (2022 bp upstream of the start codon) was amplified from L. japonicus genomic DNA and ligated into pCAMBIA1300-GFP at the SmaI restriction site, creating pCAMBIA1300-GFP-pPRP1. Subsequently, PRP1 fused with 3×HA and the nopaline synthase (NOS) terminator fragment was inserted into pCAMBIA1300-GFP-pPRP1. These constructs were introduced into the prp1 mutant plants via hairy root transformation. To generate pPRP1:PRP1-GFP transgenic lines, the PRP1 promoter fused with the PRP1 gene was cloned into the pCAMBIA1302 vector using XbaI and SpeI digestion. For yeast two-hybrid assays, the coding sequence of PRP1 and its variants were cloned into pGBKT7 [Binding Domain (BD) vector] using EcoRI and SalI and into pGADT7 [Activation Domain (AD) vector] using EcoRI and XhoI, respectively. The constructs pGBKT7-p53 (BD-p53), pGBKT7-Lam (BD-Lam), and pGADT7-SV40 (AD-SV40) were described in a previous publication (Duan et al., 2019). For the split-luciferase assay, HA-tagged nLuc and Myc-tagged cLuc were cloned into pGWB514 between the KpnI and SacI sites, creating pGWB514-35S-HA-nLuc and pGWB514-35S-Myc-cLuc. The coding sequences (CDS) of PRP1 and its variants were PCR-amplified and cloned into pGWB514-35S-HA-nLuc using XbaI and KpnI or pGWB514-35S-Myc-cLuc using XbaI and KpnI, respectively. For DNA-protein pull-down assays, the C-terminus of NIN (520–878 a.a.) was cloned into pMAL-c2x using EcoRI and SalI. All the primers used for cloning are listed in Supplementary Table S1, and all constructs were verified by DNA sequencing.
Quantitative polymerase chain reaction (qPCR)
Total RNA was extracted from roots using the EASYspin Plant RNA Kit (Aidlab). The Primescript RT Reagent Kit (TaKaRa) was used to remove gDNA from RNA samples and reverse transcribe cDNAs. qPCR was performed in a 10 μl reaction mixture containing 5 μl 2× Universal SYBR Green Fast qPCR Mix (ABclonal), 25 ng DNA template, 0.4 μM forward primer, and 0.4 μM reverse primer. qPCR was performed using a QuantStudioTM Real-Time PCR System (Thermo Fisher Scientific, USA) using the following cycles: 95 °C for 2 min, followed by 40 cycles of 95 °C for 10 s, 58 °C for 30 s, and 72 °C for 30 s. Lotus japonicus ATP synthase (GenBank ID: AW719841) and Ubiquitin (GenBank ID: AW720576) were employed as reference genes. Standard errors and statistical significance based on three or four biological replicates were calculated using the ΔCt method. The primers for qPCR are listed in Supplementary Table S1.
Hairy-root and stable transformation
For hairy root transformation, the constructs were introduced into Agrobacterium rhizogenes strain LBA1334 to infect L. japonicus seedlings following the protocol in the L. japonicus handbook (Diaz et al., 2005). For stable transformation, constructs were transferred into A. tumefaciens strain EHA105 to infect L. japonicus seedlings using a previously described protocol (Tirichine et al., 2005).
Immunostaining assay
The immunostaining assay was performed following previously established protocols (Liu et al., 2020). In brief, nodules were fixed in a solution containing 4% w/v paraformaldehyde, 60 mM sucrose, and 50 mM sodium cacodylate (pH 7.4) for 2 h before being sectioned into about 4 µm thick slices using a microtome (Leica HistoCore AUTOCUT, Germany). Sections were subjected to a 2 h incubation with 0.3% (v/v) Triton X-100, followed by overnight incubation with primary antibodies and a 2 h incubation with secondary antibodies. Anti-GFP (1:1000; CST) and Alexa Fluor 488 goat anti-rabbit immunoglobulin IgG (1:2000; Molecular Probes) were used as primary and secondary antibodies, respectively. Fluorescence was observed using a confocal laser microscope (Nikon Eclipse Ti, Japan) using the following excitation and emission wavelengths: 405 nm and 410–450 nm for cell walls; 488 nm and 507–532 nm for GFP-labelled proteins; and 561 nm and 600–650 nm for DsRed-tagged rhizobia.
Acetylene reduction assay
Nitrogenase activity was measured using the acetylene reduction assay. All the roots containing nodules at 21 d post-inoculation were placed in sealed glass vials (20 ml). Two millilitres of air were removed from the vials, and 2 ml of acetylene was injected. After incubation for 2 h at 28 °C, the vials were cooled on ice to stop the reaction. Gas from each vial (100 µl) was analysed using a GC-4000A gas chromatograph (Dongxi Company, China).
X-Gal staining
For visualizing infection events, plants were inoculated with M. loti-LacZ and harvested for analysis at 5 or 7 d post-inoculation. Roots were vacuum-infiltrated for 3 min in fix solution (1.25% glutaraldehyde dissolved in 0.1 M potassium phosphate buffer, pH 7.4) and incubated at 25 °C for 40 min. Subsequently, roots were washed twice for 10 min in 0.1 M potassium phosphate buffer. The roots were vacuum-infiltrated for 5 min with X-Gal staining solution (0.1 M phosphate buffer, 6.25 mM K4Fe(CN)6, 6.25 mM K3Fe(CN)6, and 0.75% X-Gal in dimethyl formamide) and kept overnight at 28 °C in the dark. After two washes in H2O for 10 min, the X-Gal-stained roots were examined under a fluorescence microscope (Leica DM2500, Germany).
Split-luciferase complementary assay
The corresponding constructs were transformed into A. tumefaciens strain EHA105 for co-expression in Nicotiana benthamiana leaves. A culture containing nLUC- or cLUC-fusion proteins and p19 was diluted in the infiltration buffer [10 mM 2-(N-morpholino) ethanesulfonic acid (MES, pH 6.0), 10 mM MgCl2, 200 μM acetosyringone] and mixed at a 1:1:1 ratio. The mixture was then hand-infiltrated into N. benthamiana leaves with a needle-less syringe. After incubation for 3 d, 1 mM luciferin (Promega, Madison, USA) was applied to the leaves, and images were captured with a Tanon-5200 Image Analyser (Tanon, China).
Yeast two-hybrid assay
The bait plasmid (pGBKT7) and prey plasmid (pGADT7) were transformed into yeast strains Y187 and AH109 using LiAc-mediated yeast transformation, respectively. These strains were grown for 3 d on control medium lacking Leu and Trp (SD-Leu-Trp) and selective medium that additionally lacked His and Ade (SD-Leu-Trp-His-Ade) before images were taken.
DNA-protein pull-down assay
The DNA-binding assay was conducted as described previously (Vert and Chory, 2006). Biotinylated DNA fragments corresponding to the LjPRP1 promoter were generated via PCR using biotinylated primers. The biotinylated promoter was incubated with Anti-Biotin Agarose beads (Sigma-Aldrich, USA) in TES buffer (10 mM Tris–HCl pH 7.5, 1 mM EDTA pH 8.0, 2 M NaCl) for 3 h at 4 °C and washed three times in the IP100 buffer (100 mM potassium glutamate, 50 mM Tris–HCl pH 7.5, 2 mM MgCl2, 0.05% Nonidet P-40). The proteins were combined with DNA-bound beads for 2–4 h at 4 °C. The beads were then washed three times with the IP100 buffer. Proteins were eluted in 1× SDS loading buffer by boiling for 5 min and detected by immunoblotting using an anti-maltose binding protein (anti-MBP) antibody (Yeasen, China).
Co-immunoprecipitation assay
The corresponding constructs were transformed into A. tumefaciens strain EHA105 and co-expressed in N. benthamiana. After 3 d post-inoculation, leaves were harvested to extract total proteins with plant-extracting buffer (50 mM Tris–HCl pH 7.5, 150 mM NaCl, 5 mM ethylene diamine tetraacetic acid (EDTA), 0.2% Nonidet P-40, and 1×EDTA-free protease inhibitor cocktail). Samples were centrifuged at 4 °C, 12 000 g for 20 min. Then the supernatant was incubated with 25 μl anti-FLAG M2 agarose beads (Sigma-Aldrich, USA) for 2–4 h at 4 °C. Beads were collected at 4 °C, 3000 g for 2 min and washed with ice-cold washing buffer [50 mM Tris–HCl pH 7.5, 150 mM NaCl, 5 mM EDTA, 0.2% Nonidet P-40, and 1 mM phenylmethanesulfonylfluoride (PMSF)]. Proteins were eluted by boiling in 1× SDS loading buffer and detected by immunoblotting with anti-HA and anti-FLAG antibodies (Sigma-Aldrich, USA).
MAPK3/6 phosphorylation analyses
Lotus japonicus seeds were placed upside down on B&D medium supplemented with 0.5 mM KNO3 containing 1.2% agar for hypocotyl elongation at 24 °C in the dark for 24–32 h. Ten to fourteen germinated seedlings were transferred to the B&D medium described above and kept vertical in a growth chamber at 22 °C under a 16 h light/8 h dark photoperiod for 20–30 d. Then, approximately 100 mg of plant roots were collected and incubated in sterile water overnight. The next day, the root pieces were treated with 0.5 μM flg22 or 1 μM chitin for 10 min, with sterile water as the negative control. Total root protein was isolated in the denaturing buffer [50 mM Tris–HCl pH 7.5, 150 mM NaCl, 0.1% NP-40, 4 M urea, 1×EDTA-free protease inhibitor cocktail (Sigma-Aldrich, CAT# P9599, St. Louis, MO, US)] and subjected to western blot analysis using anti-p44/42 (Cell Signaling Transduction, Danvers, MA, USA) and anti-Actin (Abclonal, Wuhan, China) antibodies.
Pathogen inoculation
For Pseudomonas syringae inoculation, WT and prp1 mutant plants were cultivated in soil for 7 d. Pseudomonas syringae pv. tomato DC3000 (Pst DC3000) was grown in King’s B medium (29 g l–1 proteose peptone, 1.5 g l–1 K2HPO4, 10 ml l–1 glycerol, 0.74 g l–1 MgSO4) for 2 d. Seedlings were infiltrated with a suspension of P. syringae DC3000 (OD600=0.0001). The stem length was documented and measured at 12 d post-infection. For Botrytis cinerea inoculation, WT and prp1 mutant plants were cultivated in soil for 28 d. Botrytis cinerea strain B05.10 was cultivated on Difco potato dextrose agar medium. Lotus japonicus leaves were placed on 0.6% water agar with spores (5 × 105 spores/ml) in the centre of the leaf discs. Lesion size was measured at 3 d post-infection.
Alignment and phylogenetic analysis
PRP1 homologous proteins were identified based on sequence similarity to L. japonicus PRP1 using the BLASTp suite at the Phytozome database (https://phytozome-next.jgi.doe.gov). Sequence alignment of PRP1 orthologues from different plant species was analysed using DNAMAN (https://www.lynnon.com/dnaman.html). Lotus japonicus PRP1 can be found at Lotus Base (https://lotus.au.dk/) under the following accession number: Lj0g3v0096089 in L. japonicus MG20 v3.0.
Quantification and statistical analysis
All experiments were repeated with at least three biological repeats with similar results. Protein band intensities and fluorescence intensities were quantified using ImageJ (https://imagej.nih.gov/ij). Statistical data were analysed using two-tailed Student’s t-tests and one-way ANOVAs.
Results
The PRP1 gene is induced by rhizobia inoculation and highly expressed in nodules
The LjPRP1 gene encodes a 242 amino acid protein with a nuclear transport factor 2 (NTF2)-like domain. Its nomenclature as Pathogenesis-Related Protein1 derives from its orthologue in Arabidopsis thaliana, a pathogenesis-related family protein (At1g78780) (Supplementary Fig. S1). Transcript analysis from the L. japonicus Gene Expression Atlas (ExpAt) indicated significantly elevated PRP1 expression levels in nodules compared to roots (Mun et al., 2016) (Supplementary Fig. S2). However, the expression level of PRP1 was not induced after arbuscular mycorrhizal (AM) fungi treatment (Supplementary Fig. S2), implying that the expression pattern of PRP1 might be specifically associated with nodule symbiosis. To further assess PRP1 expression, we employed quantitative polymerase chain reaction (qPCR) and found that PRP1 transcripts were prominently induced in nodules at 21 d post-inoculation compared to roots and other tissues (Fig. 1A). We also observed PRP1 expression dynamics from 3 to 8 weeks post-inoculation (wpi), revealing an induction of PRP1 expression upon rhizobia inoculation, peaking at 4 wpi and gradually declining by 6 wpi (Fig. 1B–C). Notably, PRP1 induction upon rhizobia inoculation was absent in the L. japonicus nfr1-1 mutant, which is unable to respond to rhizobium nodulation factors (NFs) (Radutoiu et al., 2003), and in the cyclops-1 mutant, which specifically affects rhizobia infection but not nodule organogenesis (Yano et al., 2008) (Fig. 1D). The special induction of expression by rhizobium suggests that PRP1 plays an important role regulating rhizobia infection.
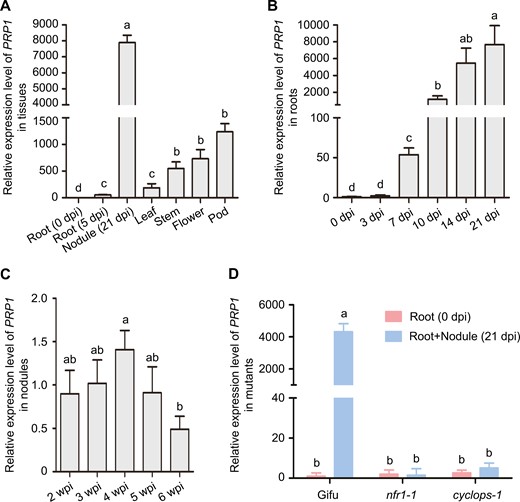
Expression patterns of PRP1 gene in L. japonicus. (A) Expression of the PRP1 gene in non-inoculated roots, roots inoculated with rhizobia at 5 d post-inoculation, nodules at 21 d post-inoculation, leaves, stems, flowers, and pods. (B) Expression of PRP1 in roots inoculated with M. loti MAF303099 at 0, 3, 7, 10, 14, and 21 d post-inoculation. (C) PRP1 expression in nodules at 2–6 weeks post-inoculation after inoculation with M. loti MAF303099. (D) PRP1 expression in the roots of Gifu, nfr1-1, and cyclops-1 at 21 d post-inoculation with M. loti MAF303099. For (A) to (D), gene expression levels were normalized to those of ATPase and Ubiquitin. Values are means ±SD (n=3). Data were analysed using a one-way ANOVA. Different letters represent classes with significant differences (P<0.05).
To validate tissue and cell specificity of PRP1 expression, we created stable transgenic L. japonicus plants expressing a PRP1-GFP fusion under its native promoter (pPRP1:PRP1-GFP). GFP signals were detected in roots and nodule primordia at 0, 3, and 7 d post-inoculation, intensifying in mature nodules at 10 d post-inoculation (Fig. 2A–G), confirming PRP1 induction in nodules following rhizobium treatment.
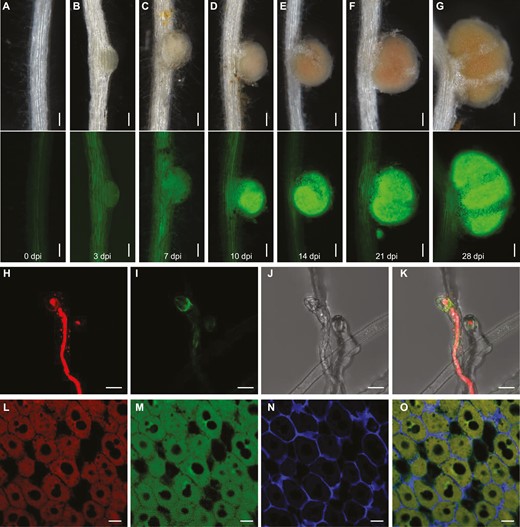
Tissue-specific expression and subcellular localization of PRP1 in L. japonicus. (A–G) Images of the pPRP1:PRP1-GFP reporter in stable transgenic plants after inoculation with rhizobia at the indicated times. Green shows PRP1-GFP signals with an excitation wavelength of 488 nm. Scale bars, 0.1 mm. (H–K) Confocal microscopy of PRP1-GFP in root hairs of pPRP1:PRP1-GFP stable transgenic plants. Images of root hairs at 5 d post-inoculation with M. loti expressing DsRed. Red shows DsRed-tagged rhizobia with an excitation wavelength of 561 nm. Green indicates PRP1-GFP signals with an excitation wavelength of 488 nm. Images are maximum intensity z projections. Scale bars, 10 μm. (L–O) Subcellular localization of PRP1 in nodules. Immunostaining of pPRP1:PRP1-GFP transgenic nodules at 21 d post-inoculation with M. loti expressing DsRed. Red shows DsRed-tagged rhizobia with an excitation wavelength of 561 nm. Green shows anti-GFP signals with an excitation wavelength of 488 nm. Blue shows signals from cell walls with an excitation wavelength of 405 nm. Scale bars, 20 μm.
PRP1 localizes in infected root hairs and symbiotic cells
To determine the subcellular localization of PRP1, we employed pPRP1:PRP1-GFP stable transgenic plants and inoculated their roots with DsRed-labelled Mesorhizobium loti to visualize infection events. Our observations revealed that the PRP1-GFP fluorescence signal was exclusive to infected root hair cells (Fig. 2H–K). To further elucidate the subcellular localization of PRP1 in nodules, we conducted in situ immunostaining in pPRP1:PRP1-GFP transgenic nodules inoculated with DsRed-labelled M. loti at 21 d post-inoculation. The GFP antibody signals were primarily concentrated in cells colonized by rhizobia (Fig. 2L–O), indicating PRP1 localization in infected cells within nodules.
NIN activates PRP1 expression via directly binding to its promoter
Considering that NIN is a master transcription factor of symbiosis and has a rhizobium treatment-induced expression pattern during nodule symbiosis (Schauser et al., 1999; Madsen et al., 2010; Soyano et al., 2014; Vernie et al., 2015), we hypothesized that NIN might regulate PRP1 expression. To test this hypothesis, we conducted transcriptional activation analyses of the PRP1 promoter co-expressed with or without NIN in N. benthamiana leaves. Leaves overexpressing NIN exhibited about four times the luciferase (LUC) activity compared to controls (Fig. 3A–B), indicating that NIN can induce PRP1 expression. To further investigate this, we assessed PRP1 gene expression in L. japonicus roots overexpressing the NIN gene. Indeed, NIN overexpression increased PRP1 expression levels at 10-fold that of the control, even without rhizobia treatment (Fig. 3C). To examine if NIN directly binds to the PRP1 promoter, we performed a DNA-protein pull-down assay. The carboxy-terminus of NIN (NINC), containing the RWP-RK domain responsible for DNA binding (Soyano et al., 2014), could pull down the biotin-labelled PRP1 promoter, as detected by immunoblotting (Fig. 3D). These results suggest that NIN directly binds to the PRP1 promoter to activate its expression.
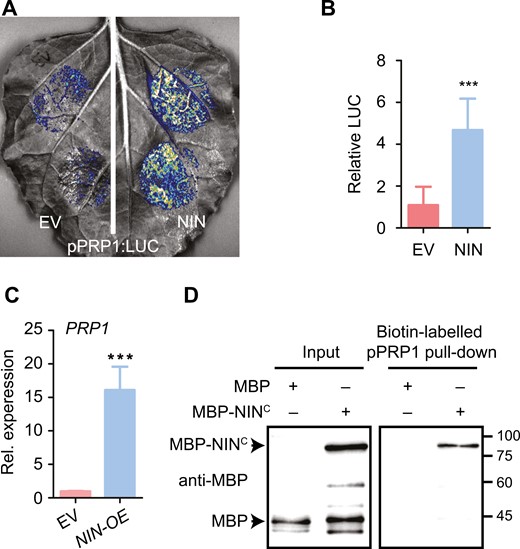
NIN binds to the promoter of PRP1 to activate its expression. (A) PRP1 promoter activity was enhanced by NIN overexpression in N. benthamiana leaves. The LUC reporter driven by the PRP1 promoter (–2022 to –1) was co-expressed with empty vector (EV) or NIN in N. benthamiana, respectively. (B) Relative luciferase (LUC) activity was estimated in N. benthamiana leaves in (A). Data are means ±SD. Asterisks indicate significant differences according to a two-tailed Student’s t-test. ***P<0.001. (C) The expression of PRP1 was analysed in the L. japonicus roots overexpressing NIN (NIN-OE) or the control (EV, empty vector) in the absence of rhizobia. Expression levels were normalized against the reference genes ATPase and ubiquitin. Values are means ±SD (n=4). Data were analysed using a two-tailed Student’s t-test. ***P<0.001. (D) NIN associates with the PRP1 promoter in a DNA-protein pull-down assay. The promoter region (–2022 to –1) of PRP1 was used. The biotin-labelled DNA fragment was generated by PCR with biotin-labelled primers. Biotin-labelled DNA fragment was incubated with MBP or MBP-NINC (520–878). MBP serves as a negative control, and inputs serve as loading controls.
PRP1 functions in rhizobial infection and nitrogen fixation
To gain insights into the function of PRP1 in nodulation, we isolated a PRP1 mutant in L. japonicus, bearing a LORE1 retrotransposon insertion in its first exon, resulting in the absence of detectable PRP1 expression (Supplementary Fig. S3A–C). Upon inoculation with M. loti strain NZP2235 containing a LacZ reporter, we observed a significantly increased number of infection threads in the prp1 mutant compared to the wild type Gifu (WT) at 5 d post-inoculation (Fig. 4A–C). Although the number of nodules in the prp1 mutant was similar to that in the WT at 21 d post-inoculation, prp1 mutant nodules exhibited a more pronounced pink colour (Fig. 4D; Supplementary Fig. S3D). Additionally, the transcript abundance of Leghaemoglobin (Lbs) genes, essential for oxygen buffering in nodules and optimal bacterial nitrogenase function, increased to about 50% in the prp1 mutants compared with WT plants (Fig. 4E). This was further confirmed at the protein level, as Lbs protein abundance was increased in the prp1 mutant nodules (Fig. 4F–G). Accordingly, the prp1 mutant nodules exhibited significantly higher nitrogen fixation capabilities, as measured by the acetylene reduction assay (ARA), compared to WT plants (Fig. 4H; Supplementary Fig. S3E–G). The plant biomass level in the prp1 mutants was similar to that in WT plants from germination to 21-day-old seedling (Supplementary Fig. S3H–I). Due to the fact that only one knockout mutant line of prp1 plant was used for nodulation phenotyping, we performed genetic complementation experiments to confirm the role of PRP1 in nodulation. PRP1 was expressed under its native promoter in the prp1 mutant roots through hairy root transformation. As shown in Fig. 4I–K, infection thread number, nitrogenase activity, and nodule colour were restored to WT levels. The data confirmed the important role of PRP1 in regulating rhizobial symbiosis in L. japonicus.
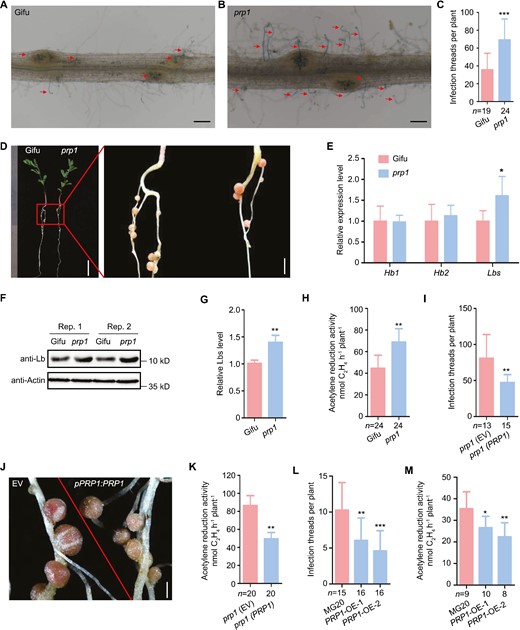
PRP1 negatively regulates nodule symbiosis. (A–C) Infection thread phenotype of wild-type Gifu (A) and the prp1 mutants (B) inoculated with M. loti MAFF303099 containing a LacZ report gene at 5 d post-inoculation. Numbers of infection threads in WT and prp1 mutants at 5 d post-inoculation (C). Scale bars, 0.2 mm in (A–B). (D) Nodule phenotypes of WT and the prp1 mutant at 21 d post-inoculation. Scale bars, 1 cm (left) and 2 mm (right). (E) Relative expression of Hb1, Hb2, and Lbs in WT and prp1 mutant nodules at 21 d post-inoculation. Expression levels were normalized against the reference genes ATPase and ubiquitin. (F–G) Lbs levels of WT and prp1 mutant nodules at 21 d post-inoculation with M. loti MAFF303099 determined by western blotting using an anti-Lb antibody. Actin was used as the loading control. Representative pictures are shown in (F) and relative protein levels are shown in (G). (H) Acetylene reduction activity of nodules in WT and prp1 mutants at 21 d post-inoculation with rhizobium. (I–K) Complementation of prp1 mutants. Transgenic roots of the prp1 mutant expressing empty vector (EV) or PRP1 under the control of the endogenous promoter, were inoculated with M. loti MAFF303099. Numbers of infection threads at 7 d post-inoculation (I), images of nodules at 21 d post-inoculation (J), and acetylene reduction activity of nodules at 21 d post-inoculation (K) were measured. Scale bars, 0.5 mm (J). (L) Numbers of infection threads in the roots of WT MG20 and two independent PRP1 overexpression lines (PRP1-OE-1 and PRP1-OE-2) at 5 d post-inoculation with M. loti MAFF303099 containing a LacZ reporter. (M) Acetylene reduction activity of nodules at 21 d post-inoculation in MG20 and PRP1 overexpression plants. All values are means ±SD. Data were analysed using a two-tailed Student’s t-test. *P<0.05, **P<0.01, ***P<0.001. ‘n’ indicates the number of plants analysed.
Additionally, we generated stable transgenic L. japonicus plants overexpressing PRP1 (PRP1-OE). Two independent lines exhibited a reduction in infection threads at 5 d post-inoculation compared to WT plants but had a similar nodule number at 21 d post-inoculation (Supplementary Fig. S4B–C). PRP1-OE plants displayed a reduction in nitrogenase activity of nodules at 21 d post-inoculation (Fig. 4M). There were no differences in plant biomass levels observed between WT and PRP1-OE plants at 21 d post-inoculation (Supplementary Fig. S4D–E). Together with the results from the prp1 mutant plants, we conclude that PRP1 plays a negative role regulating infection thread formation and nitrogenase activity in L. japonicus.
PRP1 negatively regulates intracellular rhizobia accommodation in nodules
To delve deeper into the mechanisms underlying the increased formation of pink nodules and elevated nitrogen fixation rates in the prp1 mutants, we examined nodules from prp1 and WT plants at 21 d post-inoculation by sectioning and staining with toluidine blue dye for microscopic analysis. Our observations revealed that the proportion of infected cells (stained blue in colour) per nodule was increased in the prp1 nodules compared to the WT nodules (Fig. 5A–C). The increased proportion of infected cells per nodule suggest more rhizobium colonization in nodules. We then conducted qPCR analyses of M. loti 16S ribosomal RNA and 30S ribosomal gene transcripts to monitor relative rhizobium abundance, normalized against two L. japonicus housekeeping gene transcripts. The quantification revealed that the abundance of 16S ribosomal RNA and 30S ribosomal protein transcripts was significantly higher (approximately double) in the prp1 nodules compared to the WT nodules (Fig. 5D). We also assessed intracellular rhizobium accommodation in PRP1-OE and WT nodules. The proportion of infected cells decreased in PRP1-OE nodules compared to WT nodules (Supplementary Fig. S5A, B). Hence, these data indicate that PRP1 negatively regulates the accommodation of rhizobium in nodules.
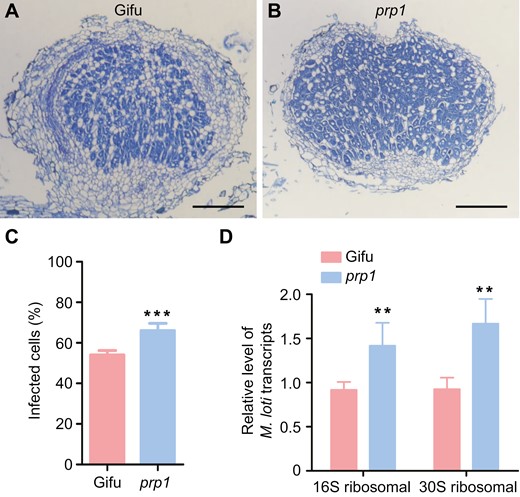
PRP1 negatively regulates rhizobium colonization in nodules. (A and B) Sections of nodules from wild-type Gifu (A) and prp1 mutant (B) plants at 21 d post-inoculation stained with toluidine blue. Scale bars, 0.2 mm. (C) Percentage of the infected cells (stained blue) in nitrogen-fixing zone of the WT(A) and the prp1 mutant (B). Values are means ±SD (n>10). Data were analysed using a two-tailed Student’s t-test. ***P<0.001. (D) Quantification of transcription of genes encoding M. loti 16S ribosomal RNA and 30S ribosomal protein in wild-type (Gifu) and prp1 mutant nodules at 21 d post-inoculation. Expression levels were normalized against the reference genes ATPase and Ubiquitin. Values are means ±SD (n=3). Data were analysed using a two-tailed Student’s t-test. **P<0.01.
PRP1 exists as a dimer during regulation of nodule symbiosis
Immunoblot analysis of the PRP1 protein in transgenic roots revealed the presence of two bands (Fig. 6A): the upper band locates at about 75 kDa, whereas the lower band locates at about 35 kDa, shown on an SDS-PAGE gel after immunoblotting, implying that PRP1 might form a homodimer. To investigate this possibility, we employed split-luciferase complementation (Fig. 6B), yeast two-hybrid (Fig. 6C), and co-immunoprecipitation (co-IP) assays (Fig. 6D) to test the PRP1-PRP1 association. Notably, PRP1-PRP1 interaction is independent of its nuclear transport factor2 (NTF2-like) domain (Fig. 6C). Since protein dimers often rely on disulfide bonds mediated by cysteine residues, we examined whether cysteine residues were necessary for PRP1 dimerization. To address this, we substituted the sole cysteine residue, Cys-238, with serine (S), resulting in PRP1C238S. Using split-luciferase complementation (Supplementary Fig. S6A) and yeast two-hybrid assays (Supplementary Fig. S6B), we discovered that mutation at C238 does not affect the PRP-PRP1 interaction. Additionally, expression of PRP1C238S was able to rescue the formation of pink nodules in the prp1 mutants (Supplementary Fig. S6C), indicating that Cys-238 in PRP1 might not be essential for its role in regulating nodulation.
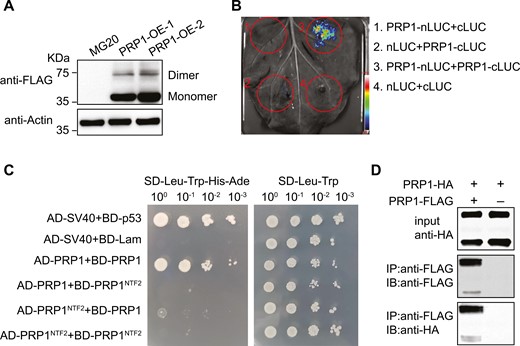
PRP1 is present as a dimer. (A) PRP1 forms as a dimer in L. japonicus. The PRP1 protein in PRP1-transgenic plants was analysed by western blotting using an anti-FLAG antibody. Actin protein was used as a loading control. Molecular weight standards are indicated. (B) Split-luciferase (Split-LUC) complementation assay showing self-interaction of PRP1 in N. benthamiana. (C) Yeast two-hybrid (Y2H) assay showing self-interaction of PRP1. Control medium lacking leucine and tryptophan (SD-Leu-Trp) and selective medium additionally lacking histidine and adenine (SD-Leu-Trp-His-Ade). BD-p53/AD-SV40 and BD-Lam/AD-SV40 were used as the positive and negative controls, respectively. (D) Co-immunoprecipitation (Co-IP) experiments showing self-interaction of PRP1. Protein immunoprecipitation was performed using anti-FLAG agarose and detected by immunoblotting using anti-HA and anti-FLAG antibodies.
PRP1 positively regulates pattern-triggered immunity
Considering that the PRP1 orthologue in Arabidopsis thaliana encodes an uncharacterized protein but is involved in plant pathogenesis, we investigated whether LjPRP1 participates in plant immune responses in L. japonicus. We observed clear reductions in MAPK3/6 phosphorylation in the prp1 mutants compared to WT roots treated with flg22 or chitin (Fig. 7A, B). To confirm the role of PRP1 mediating defence responses, we examined the expression levels of several defence-related genes, including pathogenesis-related protein 27 (PRp27-like), respiratory burst oxidase homolog protein B (RbohB-like), and CP450 in L. japonicus in response to flg22 and chitin treatments (Yoshioka et al., 2001; Lopez-Gomez et al., 2012; Shi et al., 2012; Bozsoki et al., 2017). After flg22 or chitin treatment, up-regulation of the expression of these genes was significantly reduced in the prp1 roots compared to the WT controls (Fig. 7C–H). Furthermore, prp1 mutants exhibited shorter stem lengths when infected with the bacterial pathogen P. syringae pv. tomato (Pst DC 3000) at 12 d post-infection and increased lesion sizes on prp1 leaves infected with the fungal pathogen B. cinerea strain B05.10 at 3 d post-infection (Fig. 7I–L). These results suggest that PRP1 plays a positive role in mediating plant immunity.
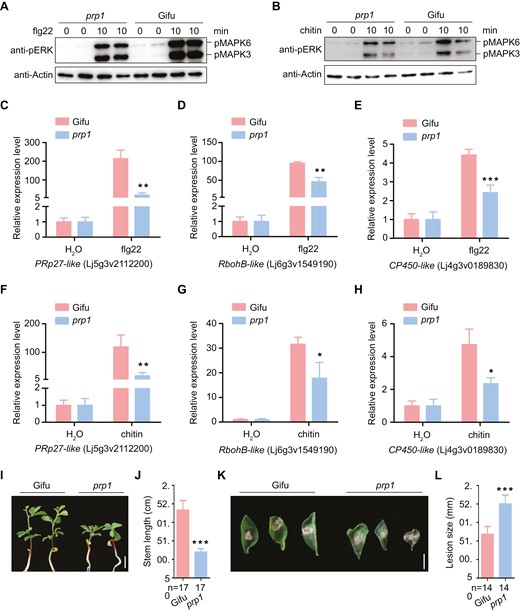
PRP1 positively regulates pattern-triggered immunity. (A–B) Flg22 and chitin induced MAPK3/6 phosphorylation in the wild type (Gifu) and the prp1 mutant, determined by immunoblotting using an α-pERK antibody (upper panel), and equal loading was verified with α-Actin antibody (bottom panel). (C–H) Gene expression in the wild-type (Gifu) and prp1 mutant plants was monitored using qPCR: expression of PRp27-like (C), RbohB-like (D), and CP450-like (E) under treatment with 0.5 μM flg22; expression of PRp27-like (F), RbohB-like (G), and CP450-like (H) under treatment with 1 μM chitin. ATPase and ubiquitin were used as controls. Values are means ±SD (n=3). Data were analysed using a two-tailed Student’s t-test. *P<0.01, **P<0.01, ***P<0.001. (I–L) Physiological responses of prp1 mutant plants challenged with Pseudomonas syringae pv. tomato DC3000 and Botrytis cinerea strain B05.10. Phenotypes (I) and stem length (J) of wild type and prp1 mutant plants inoculated with Pst DC3000 at 12 d post-infection. Phenotypes (K) and lesion size (L) of wild type and prp1 plants inoculated with B. cinerea at 3 d post-infection. Data are means ±SD and analysed using a two-tailed Student’s t-test. ***P<0.001. Scale bars, 5 mm in (I) and 2 mm in (K).
Discussion
In this study, we have demonstrated that PRP1, a pathogenesis-related family protein, plays an important role in regulating nodule symbiosis and plant immunity in L. japonicus. Remarkably, we observed a strong up-regulation of PRP1 expression in roots inoculated with rhizobium and nodules. Moreover, PRP1 exerts a negative regulatory influence on rhizobial infection in the early stages of symbiosis, as well as on the accommodation of intracellular rhizobia in the later stages of symbiosis. NIN, a pivotal transcription factor governing both rhizobia infection and nodule organogenesis (Schauser et al., 1999; Madsen et al., 2010; Liu et al., 2019; Feng et al., 2021a), transactivates the PRP1 promoter, thus orchestrating high expression levels of the PRP1 gene within nodules. Additionally, PRP1 plays a positive regulatory role in pattern-triggered immunity. Consequently, we propose that PRP1 represents a novel component that bridges the symbiotic and immune signalling pathways within nodule symbiosis.
The evolution of legume-rhizobium symbiosis involves sophisticated regulations between hosts and symbionts. Genetic studies in the past two decades have identified about 200 plant genes regulating rhizobial symbiosis (Roy et al., 2020). However, almost all the genes have homologues in non-legumes, suggesting the recruitment and/or modification of other signalling pathways from host plants for accommodating rhizobial endosymbiosis. Indeed, several proteins from other pathways have been shown to regulate nodule symbiosis in legumes. For instance, Asymmetric Leaves2-like18/Lateral Organ Boundaries Domain16a (ASL18/LBD16a), the orthologue in non-legumes essentially required for lateral root development (Goh et al., 2012), has been reported to be a target of NIN and to regulate nodule development in response to rhizobia inoculation (Schiessl et al., 2019; Soyano et al., 2019). However, the NBS (NIN-binding nucleotide sequences) targeted by NIN locates within the intron of ASL18/LBD16a. The special regulation model might play a pivotal role in incorporating this lateral root regulator into the symbiotic signalling pathway in legumes (Schiessl et al., 2019; Soyano et al., 2019). Another example came from the finding that SHORTROOT-SCARECROW (SHR-SCR), two essential proteins for controlling the root stem cell programme, are required for initiating legume-specific cortical cell division to facilitate de novo nodule organogenesis in response to rhizobia treatment (Dong et al., 2021). Here, we identify L. japonicus PRP1, the orthologue of which in Arabidopsis is a pathogenesis-related family protein with uncharacterized function, serves as a positive regulator of plant immunity, but negatively regulates nitrogen fixation and the accommodation of intracellular rhizobia in nodules. Notably, successful rhizobial infection requires the suppression of the plant defence response that resists foreign microbial invasion (Cao et al., 2017). This study demonstrated that PRP1, a component in the plant immunity pathway, is recruited into the symbiotic pathway as a direct target gene of NIN to regulate rhizobial symbiosis. PRP1 is a negative regulator of rhizobial infection and rhizobial accommodation in symbiotic cells; however, the expression of the PRP1 gene is induced after rhizobium inoculation. Therefore, the precise function of PRP1, recruited from the plant immunity pathway, might serve as a negative feedback to limit the excessive invasion and accommodation by rhizobia in symbiotic cells.
To elucidate the function of PRP1 in nodule symbiosis, we conducted a yeast two-hybrid screen using PRP1 as bait to identify its interacting partners. As shown in Supplementary Fig. S7, two proteins were identified: TNL-like (TIR-NBS-LRR resistance protein) and SCR-like protein (SCL). Previous studies have highlighted the importance of R genes in rhizobial symbiosis, exemplified by Rj2/Rfg1 and NNL1 (Nodule Number Locus1) (Yang et al., 2010; Faruque et al., 2015; Zhang et al., 2021). Additionally, several SCR and SCL proteins are essential for infection thread progression and nodule organogenesis. Examples include SCR and SCL23 in M. truncatula, SCL6-1 in Glycine max, and SIN1 (Scarecrow-like 13 involved in nodulation) in Phaseolus vulgaris (Battaglia et al., 2014; Hossain et al., 2019; Dong et al., 2021). These findings suggest that the negative regulatory role of PRP1 in nodule symbiosis may be attributable to its positive function in immunity.
The broad goal of this study is to provide new insights into the role of plant immunity in regulating legume-rhizobial symbiosis. We identified L. japonicus PRP1 as another component in the intersection of the plant immunity pathway and the symbiotic pathway. Although PRP1 plays a negative role in rhizobial infection and accommodation in symbiotic cells, the transcription of PRP1 is induced after rhizobial inoculation and is directly regulated by NIN. Therefore, the recruitment of PRP1 from the plant immunity pathway into the symbiotic pathway might serve as a negative feedback regulation of rhizobial symbiosis, because legume-rhizobial symbiosis only allows a certain number of rhizobium for infection and accommodation.
Supplementary data
The following supplementary data are available at JXB online.
Fig. S1. Phylogenetic tree of PRP1 orthologues from different species.
Fig. S2. Expression patterns of PRP1 in different tissues analysed from the Lotus Expression Atlas (ExpAt).
Fig. S3. Identification of the prp1 mutant plants.
Fig. S4. Identification of the PRP1 overexpression transgenic lines.
Fig. S5. PRP1 negatively regulates rhizobium colonization in nodules.
Fig. S6. C238 is not required for PRP1 dimerization and nodule symbiosis.
Fig. S7. PRP1 interacts with TNL-like and SCARECROW-like proteins in yeast cells.
Table S1. Primers used in this work.
Acknowledgements
We thank Dr Jens Stougaard for kindly providing cyclops-1 mutant seeds, Dr Fang Xie for kindly providing nfr1-1 mutant seeds, and Dr Tao Chen for kindly providing B. cinerea strain B05.10. We are grateful to the Centre for Carbohydrate Recognition and Signaling for providing LORE1 insertion mutant seeds. The qPCR and microscopy data were acquired from the Core Facility Center run by the National Lab of Agricultural Microbiology in Wuhan, China.
Author contributions
HL, ZZ, YC, and HZ conceived the idea and directed the research; HL, YO, and KH performed most of the experiments and analysed the data; and HL, YO, HZ, and YC wrote the manuscript.
Conflict of interest
The authors have no conflicts to declare.
Funding
This work was supported by the National Key R&D Program of China (2019YFA0904700), the National Natural Science Foundation of China (32200207 and 32090063), and the China Postdoctoral Science Foundation (2019M662652).
Data availability
Data supporting the findings of this study are available within the paper and supplementary data published online. Further information may be obtained from the corresponding author.
References
Author notes
Hao Li and Yajuan Ou contributed equally to this work.
Comments