-
PDF
- Split View
-
Views
-
Cite
Cite
Luisa M Sandalio, Jesús Espinosa, Sergey Shabala, José León, María C Romero-Puertas, Reactive oxygen species- and nitric oxide-dependent regulation of ion and metal homeostasis in plants, Journal of Experimental Botany, Volume 74, Issue 19, 13 October 2023, Pages 5970–5988, https://doi.org/10.1093/jxb/erad349
- Share Icon Share
Abstract
Deterioration and impoverishment of soil, caused by environmental pollution and climate change, result in reduced crop productivity. To adapt to hostile soils, plants have developed a complex network of factors involved in stress sensing, signal transduction, and adaptive responses. The chemical properties of reactive oxygen species (ROS) and reactive nitrogen species (RNS) allow them to participate in integrating the perception of external signals by fine-tuning protein redox regulation and signal transduction, triggering specific gene expression. Here, we update and summarize progress in understanding the mechanistic basis of ROS and RNS production at the subcellular level in plants and their role in the regulation of ion channels/transporters at both transcriptional and post-translational levels. We have also carried out an in silico analysis of different redox-dependent modifications of ion channels/transporters and identified cysteine and tyrosine targets of nitric oxide in metal transporters. Further, we summarize possible ROS- and RNS-dependent sensors involved in metal stress sensing, such as kinases and phosphatases, as well as some ROS/RNS-regulated transcription factors that could be involved in metal homeostasis. Understanding ROS- and RNS-dependent signaling events is crucial to designing new strategies to fortify crops and improve plant tolerance of nutritional imbalance and metal toxicity.
Introduction
Currently, more than a billion people suffer from malnourishment, while a similar number lack basic micronutrients, such as Zn, Fe, and Cu, in their diet. These deficiencies have a major impact on human health, and forecasts predict that this impact will become worse in the future (Semba et al., 2022). At the same time, one of the consequences of anthropogenic action is the accumulation of heavy metals, which are very harmful to all types of organisms (Huang et al., 2020). Soil pollution has become a major issue worldwide, with an increase of polluted areas in China, Australia, the USA, and Europe in particular (Yang et al., 2022). Some of these contaminated farmlands are still used to cultivate crops, posing a high risk to human health (Yang et al., 2022).
Another major contaminant in the soil is high amounts of salts (predominantly NaCl), which accumulate as a result of either natural causes (e.g. rock weathering) or inappropriate agricultural practices such as the use of low-quality water for irrigation. Globally, the area of soil affected by salinity is increasing at an alarming rate of 2–3 ha min–1 (Shabala et al., 2014), and soil salinity is expected to affect over 50% of the world population in the future (Liu et al., 2020). Salinity stress tolerance was present in the ancestors of crops but has been significantly weakened or lost during domestication (Palmgren et al., 2015; Lopez-Marques et al., 2020; Chen et al., 2021). As a result, all major staple crops (rice, wheat, maize) are highly sensitive to soil salinity.
An efficient way to deal with heavy metal contamination and salinity would be to develop crops that are able to take up significant quantities of heavy metals or salts from the soil without a yield penalty and, at the same time, prevent their accumulation in the edible plant parts. To achieve this aim, a deep understanding of the mechanisms that regulate the uptake, translocation, and sequestration of salt and heavy metals is required.
When plants are exposed to hostile soil conditions (e.g. nutritional deficiencies, salinity, or the presence of heavy metals), they increase their production of reactive oxygen species (ROS) such as ·OH, H2O2, O2·–, and 1O2. These ROS originate as by-products of aerobic metabolism and their accumulation is determined by the balance between their production and their elimination by antioxidant systems (Sandalio et al., 2012; Halliwell and Gutteridge, 2015). Uncontrolled levels of ROS are toxic: they cause oxidative stress and result in damage to various macromolecules (lipids, proteins, and DNA). However, ROS (mainly H2O2) also have an important signaling role in the control of processes such as growth, development, or the response to different biotic and abiotic stress conditions (Sandalio et al., 2012; Peláez-Vico et al., 2022). Transcriptomic studies have shown the existence of specificity in ROS signaling and responses induced by different stimuli (Gadjev et al., 2006; Vaahtera et al., 2014). The mechanisms involved are not well known but require the intervention of Ca2+ signals and other molecules such as nitric oxide (NO) and various hormones (Peláez-Vico et al., 2022; Shikha et al., 2022).
In the past years, an important role of ROS and NO in the regulation of ion channels and transporters of macro- and micronutrients as well as heavy metals has emerged (Zepeda-Jazo et al., 2011; Hafsi et al., 2022), involving post-translational and transcriptional regulation, as well as hormone balance (Cui et al., 2018; Nieves-Cordones, et al., 2019; Shikha et al., 2022). However, the molecular mechanisms responsible for this regulation remain elusive. In this review, we summarize the current standing in the field and discuss the progress made in understanding the mechanistic basis of ROS and RNS production and their role in the regulation of ion transporters and channels, at both the transcriptional and post-translational levels. This information could be of interest in designing new strategies to develop fortified crops, improve plant tolerance of salinity, and devise new phytoremediation methodologies based on redox biochemistry governed by ROS and RNS.
Production of reactive oxygen and nitrogen species at the subcellular level
Reactive oxygen species production and metabolism
The term ROS includes reduced oxygen species such as H2O2, radicals such as ·OH and O2·–, and excited forms of oxygen, the singlet oxygen 1O2 (Halliwell and Gutteridge, 2015; Sandalio et al., 2021). The chemical reactivity and biological functions of ROS differ considerably: H2O2 is the most stable form, which can even move between organelles and cells through aquaporins (Smirnoff and Arnaud, 2019; Peláez-Vico et al., 2022), whereas ·OH is the most reactive and short-lived of all ROS (Demidchik, 2014). ROS occur as a normal attribute of aerobic life, and their production and removal needs to be balanced by specific antioxidant defenses (Halliwell and Gutteridge, 2015). ROS such as ·OH can be very reactive and oxidize almost all kinds of molecules, including proteins, lipids, and DNA, promoting oxidative damage that can even give rise to cell death (Halliwell and Gutteridge, 2015; Sandalio et al., 2023). This situation can be triggered by changes in the environment that alter ROS homeostasis, such as nutritional disturbances, drought, salinity, high or low temperatures, or the presence of different pollutants (Nieves-Cordones et al., 2019; Cejudo et al., 2021). Cells have developed complex mechanisms to detect and regulate these changes to maintain metabolic functionality. ROS are also used as secondary messengers, operating in the detection of environmental changes and triggering specific changes at the transcriptional and post-translational levels (Mhamdi and Van Breusegem, 2018; Sandalio et al., 2019; Romero-Puertas et al., 2022).
ROS production and redox compartmentalization in organelles is an effective evolutionary strategy to regulate physiological process and the cellular response to stress conditions through site-specific ROS footprinting (Jones and Go, 2010; Romero-Puertas et al., 2022). The subcellular redox network facilitates rapid responses to changes in the intracellular redox equilibrium, which, in turn, regulates signaling processes and cell responses (Sandalio et al., 2021; Zoccarato et al., 2022). ROS production takes place in different cell organelles, such as chloroplasts, mitochondria, and peroxisomes, as a consequence of electron transport chains in these organelles, with the production of O2·– and further dismutation to H2O2 (Smirnoff and Arnaud, 2019; Phua et al., 2021; Sandalio et al., 2021) (Fig. 1). However, ROS production is also associated with metabolic pathways such as photorespiration (glycolate oxidase, GOX), polyamine metabolism (polyamine oxidases, PAO; copper amine oxidase, CuAO), catabolism of ureides (xanthine oxidoreductase, XOR; urate oxidase, UO), and β-oxidation of fatty acids in peroxisomes (Acyl-CoA oxidase, ACX) (Sandalio et al., 2021) (Fig. 1). In the apoplast, ROS are mainly produced in the plasma membrane by the NADPH oxidases (also called respiratory burst oxidase homologs, RBOHs; Pei et al., 2000; Peláez-Vico et al., 2022) and peroxidases (Lüthje and Martinez-Cortes, 2018; Smirnoff and Arnaud, 2019). Singlet oxygen is mainly produced in chloroplasts (Dogra and Kim, 2020). ·OH is produced by Fenton-type reactions requiring the participation of O2·–, H2O2, and Fe or Cu, and therefore could be produced in any organelle (Demidchik, 2014; Halliwell and Gutteridge, 2015). ROS levels in plants are tightly regulated by a range of enzymatic and non-enzymatic antioxidants (Smirnoff and Arnaud, 2019; Phua et al., 2021; Sandalio et al., 2021) (Fig. 1).
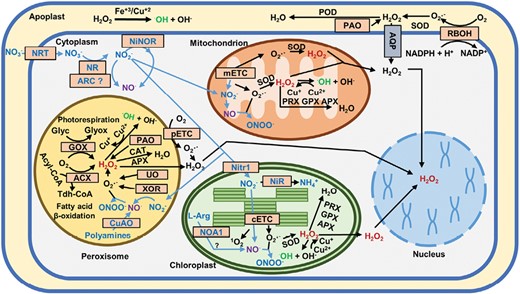
Reactive oxygen and nitrogen species metabolism at the subcellular level in plant cells. ACX, acyl-CoA oxidase; APX, ascorbate peroxidase; AQP, aquaporin; ARC, amidoxime reducing component; CAT, catalase; CuAO, copper-containing amine oxidases; ETC, electron transport chain (p, peroxisomal; c, chloroplastidial; m, mitochondrial); Glyc, glycolate; Glyox, glyoxylate; GOX, glycolate oxidase; GPX, glutathione peroxidase; NiNOR, nitrite:NO-reductase; NiR, nitrite reductase; Nitr1, nitrite transporter; NOA1, NO-associated 1 protein; NR, nitrate reductase; NRT, nitrate transporter; PAO, polyamine oxidase; POD, peroxidase; PRX, peroxiredoxin; RBOH, respiratory burst oxidase homolog; SOD, superoxide dismutase; Tdh-CoA, trans-2,3-dehydroacyl-CoA; UO, urate oxidase; XOR, xanthine oxidoreductase.
Signaling by H2O2 occurs through the reversible oxidation of specific cysteine residues from proteins to sulfenic acid (Young et al., 2019; Sies et al., 2022). Owing to their transient nature, these sulfur modifications are considered as redox switches (Young et al., 2019). Redox post-translational modification (PTM) of proteins, such as methionine oxidation, sulfenylation, and sulfinylation, as well as intra- and inter-molecular disulfide bond formation, are rapid and reversible mechanisms that regulate protein function in living cells in response to changing redox states (Fig. 2) (Sandalio et al., 2019; Young et al., 2019), while other modifications, such as sulfonylation and carbonylation, give rise to irreversible oxidation, inactivation, and further degradation of proteins (Sandalio et al., 2023). The reversible modifications can fine-tune protein function, localization, stability, and interactions in response to redox changes, to adapt the cell to environmental changes and mitigate potential damage (Young et al., 2019). Protein PTMs also trigger cell signaling pathways and cross-talk among complex interconnected signaling pathways by affecting protein–protein interactions that underpin plant stress responses. These signaling events are coupled with ROS-activated MAP kinase cascades and transcriptional regulation in both plant and animal cells (Li et al., 2022; Sies et al., 2022).
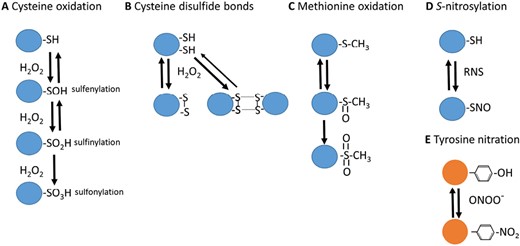
ROS- and RNS-dependent PTMs. Some of the most relevant redox-dependent PTMs associated with ROS and RNS are shown. These modifications include (A) cysteine oxidation (sulfenylation, sulfinylation, and sulfonylation), (B) cysteine disulphide bonds, (C) methionine oxidation, (D) cysteine S-nitrosylation, and (E) tyrosine nitration.
Nitric oxide production and metabolism
The way NO is synthesized and sensed in plants as well as the signaling mechanisms underlying its regulatory functions is complex and remains controversial (León and Costa-Broseta, 2020). NO can be synthesized from either oxidized or reduced N-containing precursors, with nitrate being the most abundant and relevant source that is reduced by the cytosolic nitrate reductases (NRs) through nitrite as an intermediate (Yamasaki and Sakihama, 2000; Rockel et al., 2002; León and Costa-Broseta, 2020) (Fig. 1). Other molybdoenzymes, such as amidoxime reducing component (ARC) in the cytoplasm in Chlamydomonas (Chamizo-Ampudia et al., 2016), xanthine oxidoreductases/dehydrogenases (XORs/XDHs) in the cytoplasm (Cantu-Medellin and Kelley, 2013), and peroxisomes (Sandalio et al., 2021, 2023), can also catalyze the biosynthesis of NO from nitrite. Other oxidized sources, such as xanthine, can be used under specific conditions in different photosynthetic organisms (Godber et al., 2000; Maia and Moura, 2015) (Fig. 1). Although an oxidative pathway involving NOS has also been proposed to be operational in plants (Kolbert et al., 2019), no NOS orthologs have been identified in plants (Jeandroz et al., 2016). Peroxisomes have, however, been reported to be a source of NOS-like activity in plants (Sandalio et al., 2023), although this needs further experimental support and the identification of the enzymes involved. Other oxidative pathways from polyamines or hydroxylamine, such as CuAOs, localized in the apoplast and peroxisomes have been proposed as alternative sources of NO in plants (Tun et al., 2006; Rümer et al., 2009; Wimalasekera et al., 2011; Planas-Portell et al., 2013; Zhou et al., 2016; Groß et al., 2017; Sandalio et al., 2023) (Fig. 1). The only NOS identified in the marine green alga Ostreococcus taurii (Foresi et al., 2010) was predicted to be a cytosolic enzyme (Gaudet et al., 2011), but experimental support is still needed. Under conditions of limited oxygen availability, mitochondria are an important source for NO production, with nitrite being an efficient electron acceptor (Fig. 1) (Planchet et al., 2005). The excess of NO produced in mitochondria in hypoxic conditions is modulated by the action of the phytoglobin–NO cycle (Igamberdiev et al., 2005).
NO, as a free radical, tends to react with other molecules, such as ROS, although the resulting molecules, such as hydroxyl radicals (·OH), are centers of molecular damage (Nappi and Vass, 1998; Bright et al., 2006; Sandalio et al., 2019). Some of these reactions have a relevance for NO-triggered signaling, such as the reaction of NO with superoxide-yielding peroxynitrite (ONOO–) (Radi et al., 2001; Arasimowicz-Jelonek and Floryszak-Wieczorek, 2011; Vandelle and Delledonne, 2011) (Fig.1), which is involved in the PTM of proteins by nitration of tyrosine residues (Lozano-Juste et al., 2011; León, 2022). On the other hand, the metabolic reaction of NO with the redox regulator glutathione also has high relevance in NO-triggered signaling, as the resulting S-nitrosoglutathione (GSNO) (Fig.1) is an excellent NO donor in reactions of transnitrosylation, including the S-nitrosylation of the cysteine residues of proteins (Astier et al., 2012; Zaffagnini et al., 2016) (Fig. 2). The master regulator of protein S-nitrosylation is the enzyme S-nitrosoglutathione reductase (GSNOR) (Fig.1) (Jahnová et al., 2019), which regulates GSNO levels (Feechan et al., 2005) and, at the same time, is regulated by the S-nitrosylation of key cysteine residues (Guerra et al., 2016; Zhan et al., 2018). Both NO-derived PTMs (Fig. 2) of proteins have a deep impact on the regulation of growth and development as well as responses to stress (Lindermayr et al., 2005; Astier and Lindermayr, 2012; Romero-Puertas et al., 2013; Sami et al., 2018; Sandalio et al., 2019). In fact, PTMs are the mechanism of action of NO known to date, and the recent identification of NO-regulated chromatin-modifying histone deacetylases and transcription factors may explain the role of NO in epigenetic mechanisms and modulation of gene transcription (Wurm and Lindermayr, 2021). Furthermore, NO-mediated PTMs control the redox state of cells by acting on the enzymes of the most prominent antioxidant systems and NO biosynthetic and metabolic enzymes (Pietraforte et al., 2003; Romero-Puertas and Sandalio, 2016; Begara-Morales et al., 2016; Sandalio et al., 2019; Costa-Broseta et al., 2021).
Reactive oxygen- and nitrogen-dependent regulation of metal transporters and ion channels
The transport and accumulation of essential macronutrients (N, P, K, S, Ca, Mg) and micronutrients (e.g. Fe, Cu, Zn, Mn) is mediated by transporters and channels located at the plasma membrane and tonoplast. Some of these are highly selective for specific ions whereas others may allow the passage of a large number of ions. In addition, some of them may share transcription factors or certain essential components of the signaling network that regulates their expression (Ohkama-Ohtsu and Wasaki, 2010). The above scenario is also applicable to non-essential heavy metals. For example, cadmium (Cd) does not use specific transporters and accumulates in plants through transporters of other elements, such as Fe (IRT1, NRAMP) or Zn (ZIP, ZRT), among others (Tao and Lu, 2022). ROS seem to play an important role in the root response to nutrient deprivation (Nieves-Cordones et al., 2019) and excess of heavy metals (Hafsi et al., 2022). Furthermore, changes in the plant transcriptome in NO-related mutants or in response to NO donors also involve the transport category, where the ATP-binding cassette (ABC) transporter family is usually well represented (Parani et al., 2004; Besson-Bard et al., 2009; Gibbs et al., 2014; Hussain et al., 2016) (Table 1). Only a few reports regarding the regulation of metal transporters by NO- and ROS-dependent PTMs are available, and information regarding their functionality is even scarcer. A search in the Plant PTM Viewer database (https://www.psb.ugent.be/webtools/ptm-viewer/index.php) resulted in 1317 proteins in Arabidopsis thaliana being the target of methionine oxidation, of which only five were identified as transporters; 3438 proteins that are targeted for reversible cysteine oxidation, five of which were identified as transporters; and 6836 proteins that can potentially experience sulfenylation, of which only 11 were identified as transporters and only two were related to metal transporters (ABC19 and ABCF1/GCN1; Huang et al., 2019). Among 1833 Arabidopsis proteins identified as possible targets of S-nitrosylation, only one of the three transporters identified in the gsnor1-3 mutant is associated with metal transport (Hu et al., 2015) (Table 1). To get an insight into the potential relevance of post-translational regulation by NO-derived PTMs of the metal transporters, we performed an in silico analysis of the potential nitration and S-nitrosylation of Arabidopsis metal transporters. We took advantage of the available tools for computational prediction of NO-dependent PTMs by using GPS-SNO (https://sno.biocuckoo.org/; Xue et al., 2010) and GPS-YNO2 (https://yno2.biocuckoo.org/; Liu et al., 2011) for the prediction of S-nitrosylation and tyrosine nitration sites, respectively, and DeepNitro (http://deepnitro.renlab.org; Xie et al., 2018), which allows both predictions simultaneously. We analyzed 91 Arabidopsis metal transporters belonging to the ABC, COPT, FRO, HMA, IRT, MRS2, NRAMP, OPT, POT, YSL, and ZIP families. Supplementary Table S1 summarizes the predicted NO-dependent PTMs with either S-nitrosylation or tyrosine nitration sites, although their functionality needs to be tested in direct experiments. Among the transporters predicted to be modified by both tools, ABCB25 is potentially S-nitrosylated at C639, HMA3A and HMA3B at C384, HMA5 at C10, POT5 at C762, and ZIP8 at C41. We also identified with high confidence tyrosine nitration sites for several transporters, including ABCC6 at Y695, ABCG36 at Y933, ABCG40 at Y891, HMA2 at Y10, MRS2-1 at Y259, and the high-affinity potassium transporter POT5 at Y783. Other transporters, such as the vacuolar Fe exporters NRAMP3 and NRAMP4, were identified as nitrated in our in silico analysis by only one of the prediction tools. Unfortunately, there is no information available on the functional role of the tyrosine residues that are potentially modified, so the relevance of these predictions should be experimentally confirmed and their functional implications analyzed.
PTM, post-translational modification; TR, transcriptional regulation. Up and down arrows indicate up- and down-regulation, respectively. ✓ indicates PTM by ROS or NO.
PTM, post-translational modification; TR, transcriptional regulation. Up and down arrows indicate up- and down-regulation, respectively. ✓ indicates PTM by ROS or NO.
Natural resistance-associated macrophage proteins
Natural resistance-associated macrophage proteins (NRAMPs) are an evolutionarily conserved family of proteins that function as proton-coupled metal ion transporters that can transport Mn2+, Fe2+, Zn2+, Cu2+, Cd2+, Al3+, Co2+, and Ni2+ in prokaryotic and eukaryotic organisms (Nevo and Nelson, 2006; Banerjee and Datta, 2020; Yang et al., 2022). In Arabidopsis, six genes encode members of the NRAMP transporter family (NRAMP1–NRAMP6). AtNRAMP1 is located in the plasma membrane of root cells (Fig. 3) and functions as a high-affinity Mn2+ transporter under Mn deficiency (Cailliatte et al., 2010). AtNRAMP2 is a Mn2+ transporter localized in the trans-Golgi network; knock down of AtNRAMP2 promotes a reduction of cellular redox homeostasis under Mn deficiency (Alejandro et al., 2017; Gao et al., 2018). AtNRAMP3 and AtNRAMP4 are functionally redundant and are involved in the release of metals from vacuoles; they are important for the retrieval of Fe2+ stores in seeds during germination, for the supply of Mn2+ to photosystem II in leaves, and for the response to Cd2+ stress (Thomine et al., 2003; Lanquar et al., 2004; Lanquar et al., 2010; Molins et al., 2013). AtNRAMP6 is localized to the Golgi/trans-Golgi network (Fig. 3), like AtNRAMP2, and plays an important role in intracellular Fe2+ homeostasis (Li et al., 2019) and Cd2+ distribution within the cell (Tao and Lu, 2022). Several pieces of evidence demonstrate that ROS can regulate the expression and content of some NRAMPs in Arabidopsis plants. Molins et al. (2013) showed that AtNRAMP3 protein levels were increased in roots when plants were grown in media supplemented with Cd2+ and 1 mM H2O2 for 1 week, in agreement with a survey of the publicly available microarray data indicating that AtNRAMP3 gene expression is up-regulated upon oxidative stress induced by H2O2, paraquat, and Fe excess (Gagnot et al., 2008). In fact, nramp3nramp4 Arabidopsis double mutants showed a hypersensitive phenotype when growing on media supplemented with 0.5 mM H2O2, suggesting that these transporters may be regulated by H2O2 (Molins et al., 2013). Furthermore, Arabidopsis mutants lacking RBOH C, D and F (rbohC, rbohD, rbohF), the most important sources of ROS associated with the plasma membrane (Mittler, 2017), showed up-regulation of AtNRAMP3 and AtNRAMP6 after 24 h of 50 µM Cd2+ treatment, whereas no significant changes were observed in the wild-type (WT) genotype (Hafsi et al., 2022). These results could explain the higher influx and accumulation of Cd observed in roots in response to 24 h of Cd treatment in Atrboh mutants, as well as the accumulation of Fe and Zn in roots, while translocation was inhibited for all three metals (Gupta et al., 2017; Hafsi et al., 2022). These results indicate the existence of an RBOH-dependent H2O2 regulation of AtNRAMP3 and AtNRAMP6 expression under Cd stress conditions. In rice, OsNRAMP5 has been shown to be induced by NO donors and modulated by NO in the plant response to arsenic (As) (Singh et al., 2016; A.P. Singh et al., 2017) (Table 1). Additionally, NRAMP3 is up-regulated in the triple mutant nia1nia2noa1-2 (Table 1), and NRAMP3 and NRAMP4 were predicted to be nitrated in our in silico analysis (Supplementary Table S1).
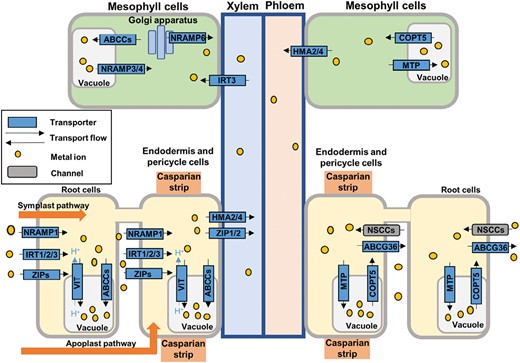
Scheme of the uptake, accumulation, and translocation of metals in plants. Metals are absorbed by the roots through the apoplastic and symplastic pathways. They are transported into the cytosol by different transporters associated with the plasma membrane and can be further transported to the vacuole through tonoplast-associated transporters. In addition, metals are translocated to mesophyll cells through the xylem, where can they accumulate in the vacuole.
ZIP family
The zinc regulated transporter and iron regulated transporter-like protein (ZIP) family (also known as ZRT, IRT-like protein) belongs to the IRT family (Yang et al., 2022). The ZIP transporters mediate the uptake of Zn, Fe, and Mn into the cytosol from the extracellular space (Fig. 3). Zn deficiency up-regulates six ZIP family genes in Hordeum vulgare (Tiong et al., 2015), and it has been suggested that ZIP transporters have an important role in plant adaptation to low and fluctuating Zn in soil in wheat (Niazkhani et al., 2021). Iron deficiency is one of the most important factors limiting crop production in the world, and IRT1 is the most important root transporter for the uptake of ferrous Fe from the soil (Zhang et al., 2019). IRT1 is highly expressed in Fe-deficient root cells to improve Fe absorption and therefore promote growth and development (Zhang et al., 2019). However, it can also mediate the uptake of other cations, such as Zn, Mn, Co, or Cd (Hu, 2021; Abuzeineh et al., 2022; Assunção, 2022; Tao and Lu, 2022). Overexpression of IRTI in Arabidopsis and rice increased their sensitivity to Zn and Cd (reviewed in Tao and Lu, 2022). Bahmani et al. (2019) have reported the up-regulation of AtIRT1 expression in Arabidopsis plants treated with H2O2 and NO donors for 24 h. In turn, the expression of the tobacco haemoglobin gene NtHb1, which acts as an NO scavenger, in Arabidopsis plants led to the down-regulation of IRT1, due to a reduction of NO and H2O2 in response to Cd exposure. Hafsi et al. (2022) showed differential expression of IRT1 in Arabidopsis WT plants and rboh mutant plants deficient in RBOH C, D, and F after 24 h of 50 µM Cd treatment. This suggests the existence of an RBOH isoform-dependent H2O2 regulation of IRT1 expression under Cd stress conditions. Other studies showed the NO-dependent induction of LeIRT1 in tomato roots grown under Fe deficiency under normal and elevated CO2 levels (Graziano and Lamattina, 2007; Jin et al., 2009), and in pear (Liu et al., 2022). By contrast, a repression of IRT1 in Arabidopsis roots under Cd stress to avoid Cd accumulation has been described (Connolly et al., 2002, 2003; Hafsi et al., 2022) (Table 1). IRT1 repression was increased in the presence of a NOS-l inhibitor, also suggesting a role for NO in the regulation of IRT1 under Cd stress (Besson-Bard et al., 2009). Similar results were described in tomato roots grown under an excess of Cd, where the NO-dependent up-regulation of IRT1 was responsible for nitrate-facilitated Cd accumulation in plants (Luo et al., 2012). GSNO also induced Arabidopsis IRT1 (García et al., 2010). Transcriptomic analysis of the NO-deficient triple mutant nia1nia2noa1-2 (Gibbs et al., 2014), showed several transporters that were differentially up- or down-regulated compared with WT plants (Table 1). In accordance with previous results, IRT1 is down-regulated in the NO-deficient mutant, while IRT3 is up-regulated (Table 1), probably to compensate for IRT1 down-regulation as overexpression of IRT3 in irt1-1 mutants recovers the irt1-1 iron-deficient phenotype (Shanmugam et al., 2011). Interestingly, ZIP5 and ZIP11 are up-regulated whereas ZIP9 is down-regulated in the triple mutant nia1nia2noa1-2 (Gibbs et al., 2014) (Table 1). On the other hand, an iTRAQ-based proteomic analysis of plasma membrane-associated proteins in rice plants exposed to Cd stress after NO treatment allowed the identification of several differentially regulated metal transporters, including the Fe transporter IRT2 (Yang et al., 2016). In addition, ZIP8, which is involved in metal transport in the rhizosphere and antioxidant activity (Wu et al., 2016), is potentially S-nitrosylated at C41 based on our in silico analysis (Supplementary Table S1).
Vacuolar iron transporters
The vacuolar iron transporter (VIT) family are tonoplast-localized transporters. They probably function as H+-dependent antiporters and are involved in fungi and plants in preventing the negative effects of Fe2+ excess (Yang et al., 2022) (Fig. 3). In rice plants, OsVIT1 and OsVIT2 can transport Fe2+ and Zn2+ into the vacuole (Zhang et al., 2012), while in wheat TaVIT2 may transport Fe2+ and Mn2+ (Connorton et al., 2017). The Ca2+-sensitive cross-complementer 1 (Ccc1) is the VIT1 ortholog in yeast, and it has been suggested that ROS can increase the activity of Ccc1 transporter (Li et al., 2010; Sorribes-Dauden et al., 2020). Similarly, ROS increase the activity of the vacuolar Ccc1 transporter in fungi (Sorribes-Dauden et al., 2020), although the underlying mechanism has not been established in any organism. Therefore, similar regulation could be applied to the VIT proteins of plants. In fact, Arabidopsis VACUOLAR IRON TRANSPORTER1-LIKE1 (VTL1) has been identified as a target of methionine oxidation by a functional protein-bound methionine oxidation proteomic analysis using Atcat2-2 mutants, and Arabidopsis VIT2 was identified as a target of oxidized cysteine that could be reversibly reduced (Jacques et al., 2015). The VIT1 gene, which is down-regulated under Fe deficiency in Arabidopsis, encodes a transporter involved in vacuolar Fe loading, while NRAMP4, a vacuolar Fe exporter, is up-regulated. Therefore, Fe homeostasis is regulated by different ROS- and NO-modulated transporters. Many examples of ROS and Fe signaling cross-talk have been observed in photosynthetic organisms, with ROS being an important signal to regulate Fe homeostasis and vice versa in plants (Thi Tuyet Le et al., 2019). Together with NRAMPs, IRTs, and ZIPs, two VIT family proteins are also differentially expressed in the triple mutant nia1nia2noa1-2 (Gibbs et al., 2014) (Table 1). All these data suggest the existence of an NO-dependent fine-tuned Fe and Zn homeostasis mechanism to achieve optimum levels of these metals, probably not only in the triple mutant nia1nia2noa1-2 but in general.
Cu transporters
The COPT/Ctr Cu transporters play an important role in Cu2+ uptake and homeostasis, and are localized in the plasma membrane of root tip cells (Shin et al., 2012). Arabidopsis COPT5 has been identified as a target of reversible cysteine oxidation by using OxiTRAQ, a quantitative redox proteomics approach (Liu et al., 2014), and therefore its activity could be regulated by ROS-dependent redox changes. Yang et al. (2016) also identified OPT3 as differentially regulated in an iTRAQ proteomic analysis of plasma membrane-associated proteins in rice plants exposed to Cd stress after NO treatment.
Heavy metal transport/detoxification superfamily proteins
Heavy metal transport/detoxification superfamily proteins (HMPs) play key roles in heavy metal transport and detoxification in plant cells. HMPs are metalloproteins or metallochaperone-like proteins containing heavy metal-associated (HMA) domains with two cysteine residues that bind and transfer Cu, Cd, Co, Zn, and other heavy metal ions (Li et al., 2020). Plant proteins containing HMA domains fall into several groups: HPPs (heavy metal-associated plant proteins), HIPPs (heavy metal-associated isoprenylated plant proteins), ATX1-like and P1B-ATPase (heavy metal ATPases; HMAs). HMAs have been widely studied in different species (Li et al., 2020; He et al., 2020) and are mainly located at the plasma membrane, where they are involved in long-distance transport of ion metals such as Cd, Zn, and/or Cu (Fig. 3). In Arabidopsis, HMA5 is induced by high Cu levels and causes the efflux of excess Cu from the cytosol to the plasma membrane (Li et al., 2020); however, different HMPs can differ in their metal specificity and the organ in which they function (Li et al., 2020). Information on the regulation of HMPs by ROS is scarce; however, a transcriptomic analysis of Arabidopsis WT and acx1 mutant plants (Romero-Puertas et al., 2022) revealed ACX1-dependent differential regulation of HMP43 and HMP20, thus suggesting a possible regulation by H2O2 produced during β-oxidation in the cell. Interestingly, the analysis on the Arabidopsis gox2 mutant transcriptome (Terrón-Camero et al., 2022) did not show any change in the expression of these genes, suggesting a differential regulation of HMPs depending on the ROS source. Less information is available on ROS-dependent PTMs of this group of proteins. HIPP34 has been identified as a target of reversible cysteine oxidation in a functional OxiTRAQ analysis of Arabidopsis cultured cells exposed to H2O2 (Liu et al., 2014). However, the functionality of this PTM requires further analysis. Interestingly, genes encoding the HMPs and HIPPs superfamily proteins are highly represented among the down-regulated genes in the nia1nia2noa1-2 triple mutant (Table 1). On the other hand, in our in silico analysis the Cd/Zn-transporting ATPases HMA3A and HMA3B were predicted to be S-nitrosylated in a cytoplasmic loop at C384, which is close to the D397 that seems to act as a binding site in the 4-aspartylphosphate intermediate. The probable Cu-transporting ATPase HMA5, which is involved in Cu detoxification in roots (Andrés-Colás et al., 2006), was predicted to be S-nitrosylated at C10, which in the AlphaFold three-dimensional structure is far from any of the C62, C65, C140, and C143 residues involved in Cu binding. Only C65 was also predicted to be S-nitrosylated, but by only one of the predictive tools (Supplementary Table S1). In turn, the nitration of HMA5 at C10, and of HMA2 at Y10, was also predicted with high confidence (Supplementary Table S1). Additionally, among the 926 endogenous S-nitrosylated proteins identified in Arabidopsis, the chloroplastic Cu-transporting ATPase PAA1 (HMA6, Q9SZC9) was identified (Hu et al., 2015).
ABC transporters
ABC transporters are a multimeric family of proteins that use ATP to transport a variety of substances (including carbohydrates, ions, lipids, xenobiotics, antibiotics, heavy metals, and drugs) to and from cells, mainly into the vacuole (Do et al., 2021). ABC transporters are classified into eight subfamilies based on their structure and function (Do et al., 2021). Only C-type ABC transporters (ABCC; classically referred to as multidrug resistance-associated proteins, MRPs) are found on the tonoplast, where they play a role in plant metal tolerance, maintaining the transport of metals into vacuoles to prevent their harmful effects (Jogawat et al., 2021) (Fig. 3). As an example, AtABCC3 takes part in transporting phytochelatin and its complexes with Cd (Brunetti et al., 2015), as well as other metals such as Mn and Zn, to the vacuole (Jogawat et al., 2021), and ABCC4 transports Cd from the cytoplasm to the vacuole in Ophiopogon japonicus (Zhao et al., 2022). Moreover, AtABCC6, which is also involved in metal tolerance, is induced in Arabidopsis plants exposed to Cd treatment (Gaillard et al., 2008). Some members of the ABC family can be transcriptionally regulated by ROS. The up-regulation of AtABCC6 in response to Cd treatment has been found to be GOX2-dependent in Atgox2 mutants (Terrón-Camero et al., 2022), thus suggesting an H2O2-dependent regulation of this transporter associated with photorespiration (Table 1). In wheat seedlings exposed to 10 µM H2O2, Bhati et al. (2015) showed up-regulation of the expression of TaABCC3, TaABCC4, TaABCC6, and TaABCC13, while TaABCC9, TaABCC14, and TaABCC16 were down-regulated, suggesting that these transporters can be differentially regulated by H2O2. ABCG is the largest ABC transporter subfamily in plants and plays a critical role in heavy metal tolerance. AtABCG36/AtPDR8 is located at the plasma membrane of root cells and plays a role as a Cd extrusion pump (Kim et al., 2007). Bahmani et al. (2019) showed that PDR8 expression increased in response to Cd but decreased in plants treated with H2O2 and NO, and the opposite results were obtained in Arabidopsis plants expressing tobacco Hb1, with reduced production of ROS and NO. Wu et al. (2019) proposed a model in which Cd stress inhibits the expression of mitochondrial MMDH2 (malate dehydrogenase 2), reducing ROS levels, in turn leading to increased expression of ABCG36, which finally reduces Cd accumulation. Furthermore, Arabidopsis AtABCG40/PDR12 is located at the plasma membrane and is strongly induced by Pb2+ treatment, and its overexpression leads to plants being more Pb2+ tolerant due to Pb2+ efflux (Lee et al., 2005). Transcriptomic analysis of WT and Atgox2 mutant Arabidopsis after Cd stress for 24 h (Terrón-Camero et al., 2022) allowed the identification of GOX2-dependent differential expression of AtABCG40, AtABCG16, and ABCB12/PGP12, suggesting a possible regulation of these transporters by peroxisomal H2O2. ABCB1 activity in rat brain capillaries is apparently regulated by NO produced by inducible NO synthase in combination with protein kinase C (PKC), with NO reducing the activity, although the underlying mechanism is not well known (Crawford et al., 2018). Interestingly, transporters from the ABC family have been shown to be modulated by NO in plant response to As (P.K. Singh et al., 2017), and regulation of Cd transport through the activation of ABC transporters was shown to be one of the major mechanisms involved in NO-dependent Cd detoxification in tall fescue after an integrated transcriptomic and metabolomic analysis (Zhu et al., 2020). The mitochondrial ABC transporter B family member 25 (ABCB25), which seems to be essential for exporting Fe/S cluster precursors from mitochondria into the cytoplasm (Bernard et al., 2009) and mediates glutathione-dependent resistance to heavy metals (Kim et al., 2006), was predicted in our in silico analysis to be S-nitrosylated at C639, close to the C-terminus and not located in a transmembrane domain (Supplementary Table S1). As mentioned before, we also identified with high confidence tyrosine nitration sites for several ABC transporters, including ABCC6 at Y695, ABCG36 at Y933, and ABCG40 at Y891 (Supplementary Table S1). Further analysis would be required to stablish the role of these tyrosine nitration sites in the regulation of those ABC transporters.
Cation/proton exchanger (CAX) family
The cation/proton exchanger (CAX) family comprises vacuole-localized transmembrane antiporters that use secondary active transport to exchange cations from the cytoplasm with protons, transporting cations to the vacuole to maintain ion homeostasis in guard cells (Pittman and Hirschi, 2016; Yang et al., 2022). Some CAX isoforms have broad substrate specificity, providing the ability to transport trace metal ions such as Mn2+ and Cd2+. AtCAX3 has been shown to be involved in the efflux of Ca2+, Zn2+, and Cd2+ in Arabidopsis plants (Yang et al., 2022). In halophytic plants, CAXs have been reported to play a role in salt tolerance (Pittman and Hirschi, 2016). Bahmani et al. (2019) showed that the transcription of AtCAX3 was increased in response to Cd in Arabidopsis WT plants, whereas it was decreased in plants treated with H2O2 and NO for 24 h, and increased in NtHb1-expressing Arabidopsis plants, thus demonstrating a redox regulation of CAX3 expression (Table 1). The expression of NtHb1 in Arabidopsis regulates Cd transporter expression by decreasing NO and ROS levels, down-regulating IRT1 and PDR8, while up-regulating CAX3, giving rise to a reduction in the Cd levels in roots and shoots (Bahmani et al., 2019). Additionally, CAX1 is among the genes differentially regulated in the NO-deficient triple mutant nia1nia2noa1-2 (Table 1).
Non-selective cation channels
Ca2+-permeable channels have been shown to be activated by H2O2 in many plant systems, such as root epidermal cells (Demidchik et al., 2003, 2007), guard cells (Pei et al., 2000), and pollen tubes (Breygina et al., 2016). In intact plants, this activation leads to an increase in cytosolic free Ca2+, which occurs in a dose-dependent manner (Leshem et al., 2007; Ma and Berkowitz, 2011). However, in patch-clamp experiments H2O2 did not activate whole-cell currents in protoplasts isolated from the Arabidopsis mature root epidermis (Demidchik et al., 2007), suggesting that the above stimulatory effects of H2O2 on the rapid rise in cytosolic free Ca2+ may be indirect and mediated by ·OH produced in the cell walls (Demidchik, 2015). In addition, H2O2-induced activation of Ca2+ currents was observed only when H2O2 was applied to the cytosolic side of the membrane (Demidchik and Maathuis, 2007), implying a need for its transport (through aquaporins) across the plasma membrane for in planta operation. In mitochondria from mammalian cardiac muscle, the Ca2+ release channels/ryanodine receptors (RyR2s), which are cation-selective channels that have a high ion conductance for both monovalent (K+) and divalent (Ca2+) cations, can be regulated by oxidation and S-nitrosylation (Meissner, 2004). Twenty-one cysteine residues per RyR2 subunit were reported to be in a reduced state and could be potential targets for redox modifications including S-nitrosylation and disulfide cross-linking (Nikolaienko et al., 2018). In mammalian tissues, SR/ER Ca2+-ATPase (SERCA) can also be modified by cysteine oxidation or tyrosine nitration, while plasma membrane Ca2+ ATPase (PMCA) is inhibited either by its direct oxidation or by methionine oxidation in its binding partner calmodulin (O-Uchi et al., 2014).
While the above reports referred to Ca2+-permeable ion channels, no specific Ca2+-selective channels have been reported so far in plants (Demidchik et al., 2018), and Ca2+ uptake across cellular membranes is mediated by non-selective cation channels (NSCCs). These NSCCs are permeable to a wide range of cations, including essential macronutrients (K+, Ca2+, Mg2+, NH4+) and micronutrients (Zn2+, Fe2+) (Demidchik and Maathuis, 2007), as well as toxic nutrients such as Na+, Cd2+, or Al3+. The Arabidopsis genome contains 40 NSCCs in total, divided into two main families: cyclic nucleotide gated channels (CNGCs; 20 genes in Arabidopsis) and ionotropic glutamate receptors (GLRs; 20 genes) (Maathuis, 2006; Demidchik and Maathuis, 2007). In addition, tonoplast-based TPC (Two-Pore Cation) channels and several types of mechanosensitive channels, such as MSL (MscS-Like), MCA (Mid1-Complementing Activity), and OSCA (hyperosmolality-gated Ca2+-permeable) are also classified as NSCCs (Basu and Haswell, 2017; Liu et al., 2018).
Activation of NSCCs has been reported for several types of plant systems, both for H2O2 and for ·OH. In root epidermis, ·OH activates NSCCs, triggering a simultaneous Ca2+ influx and K+ efflux (following the electrochemical gradient for these ions); this activation has been observed in both the mature root zone and the root apex of a large number of plants (Demidchik et al., 2003; Zepeda-Jazo et al., 2011; Velarde-Buendía et al., 2012). In patch-clamp experiments, ·OH-induced activation of Ca2+ influx and K+ efflux conductances was reported in Arabidopsis roots (Demidchik et al., 2003), although the mechanism has not been elucidated. The extent of ·OH-induced activation of NSCCs has often been negatively correlated with abiotic stress tolerance in plants, specifically with plants’ ability to adapt to soil salinity (Bose et al., 2014; Wang et al., 2018; Liu et al., 2019). This is hardly surprising, as NSCCs are also permeable to Na+ (Demidchik and Tester, 2002) and salinity-stress induced accumulation of ROS in root tissues may form a positive feedback loop exacerbating Na+ uptake into root epidermis. Exogenous H2O2 activates Ca2+-permeable NSCCs in protoplasts isolated from Arabidopsis guard cells (Pei et al., 2000) and in the outside-out mode from the cytoplasmic side in root epidermis (Demidchik et al., 2003, 2007).
NO is also able to modulate Na and K nutrition in plants under salinity stress, as the Na transporter CNGC1 appears to be regulated by NO in Brassica napus (Huang et al., 2022). Moreover, the function of the AKT1 channel, via overproduction of an active form of vitamin B6 (pyridoxal 5ʹ-phosphate), is repressed by NO in Arabidopsis (Xia et al., 2014) (Table 1). The sodium exchanger-encoding gene NHX2 has also been identified as one of the genes that are differentially regulated in the NO-deficient triple mutant nia1nia2noa1-2 (Table 1). NO also selectively regulates abscisic acid-dependent Ca2+-sensitive K+ and Cl– channels of Vicia faba guard cells, inducing Ca2+ release from intracellular stocks (García-Mata et al., 2003) (Table 1). Ciorba et al (1999), using ShC/B voltage-dependent K+ channels expressed in Xenopus oocytes as a model system, demonstrated that NO slows down the time course of K+ channel inactivation by oxidizing a critical methionine residue in the inactivation ball domain of the channel protein. Additionally, the channel protein was protected from methionine oxidation by methionine sulfoxide reductase and vitamin C (Ciorba et al., 1999).
K+-selective efflux channels
Shaker-type depolarization-activated outward-rectifying K+-efflux GORK channels are present in both guard cells (hence their name—Guard Cell Outward Rectifying K+ channel) and root epidermis, and are known to be activated by ·OH. Discovered first in Arabidopsis (Demidchik et al., 2003, 2010), ·OH-activated GORK channels have since been reported in pea root epidermis (Zepeda-Jazo et al., 2011) and in barley root cells, where their activation correlated with salt sensitivity (Velarde-Buendía et al., 2012); more recently, ROS-activated GORK channels were found to be essential for Arabidopsis responses to hypoxia stress (Wang et al., 2017). ·OH-induced K+ efflux was much stronger in the elongation zone than in mature epidermis (Shabala et al., 2016), and it was causally associated with cell fate determination under stress conditions (Demidchik et al., 2010). A similar scenario may be envisaged for plants exposed to toxic metals such as Cu2+ or Fe3+, as interaction between these transition metals and H2O2 may lead to the formation of ·OH through the Fenton reaction in the cell walls (Demidchik, 2015), triggering K+ loss through GORK. Cd triggers the down-regulation of GORK transcripts in WT Arabidopsis plants, whereas no significant changes were observed in RBOH C, D, and F mutants (Hafsi et al., 2022). Interestingly, the basal level of GORK transcripts in control conditions was reduced significantly in AtrbohC, and to a lesser extent in AtrbohD and AtrbohF, thus suggesting an RBOH-dependent regulation of GORK at the transcriptional level and possibly at the post-translational level (Hafsi et al., 2022).
Tran et al. (2013) also reported that, in addition to a rapid activation of GORK at the single-channel level, ROS-dependent post-transcriptional regulation of GORK channels may occur. The abundance of GORK channel transcripts increases in a time-dependent manner after ozone (O3) treatment, and Tran et al. (2013) attributed this effect to pre-mRNA GORK splicing. It should also be noted that GORK transcript levels are increased in plants exposed to abiotic stresses, specifically salinity (Adem et al., 2014).
Some reports on guard cells have shown that both inward- and outward-rectifying K+-selective channels in guard cells may be inhibited by H2O2 (e.g. in V. faba; Zhang et al., 2001; Köhler et al., 2003). At the same time, Laohavisit et al. (2012) have demonstrated the presence of an additional K+ efflux pathway that is catalyzed by annexins, as an Arabidopsis loss-of-function mutant for annexin1 (Atann1) lacked ·OH- activated Ca2+- and K+-permeable conductance in root epidermis. Thus, at least two mechanisms seem to coexist and act in concert to amplify ·OH-induced K+ efflux.
Other ROS-regulated channels and transporters
Exogenous H2O2 stimulates anion efflux in cultured Arabidopsis cells (Trouverie et al., 2008); however, this effect appears to be indirect and related to the activation of Ca2+ conductance, which in turn activates Cl– currents (Demidchik, 2015). Kadono et al. (2010) demonstrated that 3 min of O3 exposure was enough to activate anion currents in cell suspensions and depolarize the plasma membrane. However, as the authors used a voltage-clamp approach on intact cells, it cannot be excluded that the reported effect was indirect and also mediated by O3-induced changes in cytosolic free Ca2+. More direct experiments on protoplasts are therefore needed.
Organelle-based ion channels also appear to be a target for ROS regulation. Pastore et al. (2007) showed that ROS can quickly stimulate the ATP-sensitive plant mitochondrial K+ channel (PmitoKATP) in wheat. The activity of mitochondrial K-ATP+ in pea was also modulated by NO and H2O (Chiandussi et al., 2002), leading to a release of cytochrome c and consequent programmed cell death. Activation of tonoplast-based Ca2+-, K+-, and Na+-permeable SV (slow vacuolar) channels encoded by the TPC1 gene by physiologically relevant concentrations of H2O2 has also been demonstrated in direct patch-clamp experiments (Pottosin et al., 2009).
To the best of our knowledge, no direct reports of ROS-induced activation of members of the HAK/KUP family of high-affinity K+ transporters has been reported in plants, although many papers have reported an apparent correlation between changes in the expression levels of these transporters and ROS metabolism in plants. Overexpression of MiHAK14 from Mangifera indica in Arabidopsis enhanced plant tolerance to K+ depletion and NaCl stress by improving ROS scavenging ability (Zhang et al., 2022). Similar findings were reported for Casuarina equisetifolia, where CeqHAK6 and CeqHAK11 increased antioxidative defences (Wang et al., 2023). In turn, ROS accumulation may affect the transcript levels of HAK/KUP family genes. In Arabidopsis, plants overexpressing RCI3, a member of a family of peroxidases, showed higher ROS production and increased AtHAK5 expression levels (Kim et al., 2010). AtHAK5 and AtKUP8 have been also reported to be regulated by RBOH-dependent H2O2 (Wang et al., 2021; Hafsi et al., 2022). We have also identified C762 from HAK5 as a target for S-nitrosylation (Supplementary Table S1). Arabidopsis Atkup8-2 mutant plants showed lower accumulation of H2O2 compared with WT plants when grown in the presence of heavy metals (Sanz-Fernández et al., 2021), and plants overexpressing PvHAK16 from seashore paspalum (Paspalum vaginatum) showed increased accumulation of ROS under salt stress (Dai et al., 2022). Members of the KUP family, such as KUP5 and KUP6 and the antiporter KEA1 for potassium transport, are differentially regulated in nia1nia2noa1-2 triple mutants compared with the WT (Table 1), implying a role for NO in their operation.
Additionally, some proteins can change their functionality after oxidation. This is the case for the heme transporter HmuR in the bacterium Burkholderia thailandensis T6SS4. HmuR is a redox-regulated dual-functional transporter that under normal conditions transports heme iron but can transport zinc under oxidative stress, following the formation of an intramolecular disulfide bond in the protein (Si et al., 2017).
ROS- and NO-sensing mechanisms involved in the regulation of ion channels and transporters
One of the intriguing questions in plant redox biology is the identification of ROS/redox sensors (Sierla et al., 2016). In general, ROS have been shown to change the activity of a large number of regulatory enzymes, such as various kinases (e.g. MAP and other Ser/Thr kinases) and phosphatases (Apel and Hirt, 2004; Van Breusegem et al., 2008; Pitzschke and Hirt, 2009).
Owing to its low redox buffering capacity, the apoplast is an excellent medium for ROS signal propagation, and it harbors a large number of cysteine-rich kinases that could possibly participate in ROS-sensing mechanisms (Bourdais et al., 2015). For example, cysteine-rich receptor-like kinases (CRKs) represent one of the largest subgroups of receptor-like kinases and are ideally suited for the role of ROS sensors (Wrzaczek et al., 2010; Bourdais et al., 2015). The CRKs possess two cysteine-rich DUF26 domains (C-X8-C-X2-C-motifs) and, upon ROS binding, could undergo redox modifications leading to conformational changes and downstream signaling. In stomata guard cells, apoplastic ROS signals may be perceived by GHR1 (GUARD CELL HYDROGENPEROXIDE-RESISTANT1), an atypical plasma membrane-associated leucine-rich repeat receptor-like kinase (Hua et al., 2012). Another possible candidate sensor is CPK21 (Ueoka-Nakanishi et al., 2013), which can also activate guard cell-expressed anion channels (Geiger et al., 2010). Furthermore, the expression of IRT1 in Arabidopsis mutants lacking MAPK3 and MAPK6 (mpk3 and mpk6, respectively) was shown to be down-regulated under Fe deficiency (Ye et al., 2015). MAPK3 and MAPK6 participate in a MAPK pathway downstream of ROS, contributing to both abiotic and biotic stress signaling (Opdenakker et al., 2012; Jalmi et al., 2018), suggesting the existence of H2O2-dependent regulation of IRT1 via the MAPK pathway.
Plant transcription factors may also potentially assume the role of ROS sensors, as mentioned before (Hong et al., 2013; Demidchik, 2015). Known examples include the transcription factor TGA1, which possesses two specific cysteine residues (C260 and C266) that could be oxidized (Després et al., 2003). Another example is the heat shock transcription factors, which could also be involved in direct ROS sensing (Hong et al., 2013). Brassica juncea BjCdR15, a bZIP transcription factor orthologue of Arabidopsis TGA3, is a regulator of Cd uptake, translocation, and accumulation in shoots, and confers Cd tolerance in transgenic plants by regulating the expression of AtHMA4, AtNRAMP3, AtABCC3/AtMRP3, and AtABCG36/AtPDR8 (Farinati et al., 2010). TGA3 transcript levels were up-regulated by Cd exposure in WT and AtrbohC plants, whereas no significant changes were observed in AtrbohD and AtrbohF, suggesting that H2O2 from RBOHD and RBOHF could regulate TGA3 (Hafsi et al., 2022). Herrera-Vásquez et al. (2021) have provided evidence to support the idea that TGA class II (TGA2/5/6) transcription factors represent a redox regulatory node in biotic and abiotic stress responses. Additionally, TGA2/5/6 impact on the cellular redox state by controlling the expression of genes responsible for restraining ROS accumulation (Herrera-Vásquez et al., 2021).
Transcriptomic analyses of genes induced by increasing intracellular H2O2 levels in cat2 Arabidopsis mutants and Arabidopsis mutants with altered ROS production have allowed the identification of several transcription factors in the WRKY, AP2/ERF, MYB, NAC, HSF, and ZAT families (He et al., 2018; Terrón-Camero et al., 2022). WRKY46 plays an important role in the control of root-to-shoot Fe translocation under Fe deficiency conditions via the direct regulation of VTL1 transcript levels (Yang et al., 2016). It should be also borne in mind that ABC transporters are targets of nuclear factor erythroid 2-related factor 2 (Nrf2) in mice (Aleksunes and Klaassen, 2012) and Nrf2 is activated by ROS in animal cells (Marinho et al., 2014). Other transcription factors regulated by ROS in animal cells include CREB, TP53, NOTCH, NF-kB, SP1, HIF-1, SREBP-1, and HSF1, which have been considered as H2O2 sensors (Marinho et al., 2014). Moreover, in plant cells, the transcription factor WRKY13 activates AtABCG36 expression to positively regulate Cd tolerance (Sheng et al., 2019; Li et al., 2022). WRKY transcription factors have been identified as potential downstream targets of MAPKs (Jalmi et al., 2018). The fact that ROS can lead to the activation of MAPK kinases (Li et al., 2022; Torres and Forman, 2003) may suggest that H2O2 can regulate ABCG36 expression via MAPK-dependent activation of WRKY13. The zinc finger transcription factor ZAT12 has been reported to be regulated at the transcriptional and post-translational levels by ROS in Arabidopsis, and to be up-regulated by prolonged Fe deficiency. Thus, ZAT12 could be involved in the cross-talk between ROS and Fe uptake regulation (Gratz et al., 2021).
While the operation of the above sensory molecules is plausible and supported by numerous pieces of evidence, none of these models can explain the rapid activation of ion channels reported in patch experiments using a single-channel mode (e.g. Demidchik et al., 2007), suggesting direct ROS sensing by ion channel(s) per se. Garcia-Mata et al. (2010) demonstrated that the SKOR K+ efflux channel was activated by H2O2 when heterologously expressed in HEK293 cells. Moreover, the substitution of the C168 residue on the S3 α-helix of the voltage sensor complex by another amino acid led to the loss of sensitivity of SKOR to H2O2 (Garcia-Mata et al., 2010). As the above cysteine residue also exists in GORK (Demidchik et al., 2014), this could explain a direct activation of the GORK channels by ROS, discussed above.
As for the NSCC channels, the bioinformatics analysis revealed the presence of two candidates among the CNGCs, namely CNGC19 and CNGC20, that possess putative Cu/Fe-binding sites that could represent the cysteine metal pockets situated in the first cytosolic domain of CNGC (Demidchik et al., 2014). Such cysteine residues have been shown to be responsible for ·OH-mediated activation of Ca2+-permeable channels in animal cells (Simon et al., 2004).
Regarding NO signaling, NRAMP3 and NRAMP4 have been reported recently to be transcriptionally regulated by S-nitrosylated bHLH29, bHLH38, and bHLH101 transcription factors (Shee et al., 2022). An NO-sensing mechanism based on the N-degron pathway-mediated degradation of clade VII ethylene response factor (ERF) transcription factors (ERFVIIs) has been reported to control a wide array of plant developmental and stress-related responses (Gibbs et al., 2014; Abbas et al., 2015). The ERFVIIs group comprises three constitutively expressed genes, RAP2.2, RAP2.3/EBP, and RAP2.12, as well as two hypoxia-inducible genes, HRE1 and HRE2, which have been demonstrated to be substrates of the E3 ubiquitin ligase PROTEOLYSIS6 (PRT6), a key regulator of the Cys/Arg branch of the N-degron pathway (Gibbs et al., 2015). RAP2.3/EBP/ERF72 could directly bind to the promoter regions of Fe-deficiency response genes including the Fe transporter IRT1, HA2, and CLH1 to exert negative regulation on responses to Fe deficiency (Liu et al., 2017). In woody apple plants, MbERF72 suppress Fe uptake by modulating an H+-ATPase and, consequently, the rhizosphere pH (Zhang et al., 2020). The expression of RAP2.2 is controlled by two WRKY family transcription factors, WRKY33 and WRKY12, during hypoxia-triggered responses (Tang et al., 2021). The WRKY33-ATL31-IRT1 module has been recently reported to play a crucial role in blocking Cd absorption to prevent metal toxicity in Arabidopsis (Zhang et al., 2023). Extensive work will be needed to assess whether ERFVII signaling and WRKY-related regulation of metal homeostasis are linked and, if so, to what extent.
Conclusions and perspectives
Plants have developed an evolutionary strategy to regulate their response to environmental changes, including nutritional imbalance and toxic heavy metals and salinity, through a site-specific ROS and NO footprint (Gibbs et al., 2014; Zhu et al., 2020; Romero-Puertas et al., 2022). Some of the targets of ROS- and NO-dependent transcriptional regulation are ion/metal transporters, with major implications for plants’ capacity to sense and adapt to biotic and abiotic stresses.H2O2 and NO modify reversibly specific cysteine residues (or tyrosine residues in the case of ONOO–) in proteins. ROS and NO interact both with each other and with proteins involved in their production and metabolism, regulating their own levels and the cellular redox equilibrium. The identification of redox-sensitive nutrient sensors in plant cells, their specificity, and the mechanisms involved in decoding these signals is one of the challenges for the field. A number of metal transporters, ion channels, MAPKs, and transcription factors appear to be regulated transcriptionally by ROS and NO, although the mechanisms underlying the network involved in this regulation is far from being completely known. Further methodological improvements will allow better identification of membrane proteins to more completely address the relevance of redox changes in the functionality of metal and ion transporters. However, the ·OH-dependent activation of Ca2+-permeable NSCC and SKOR has been well established. Here, we have also implemented our knowledge of NO-dependent regulation of metal transporters by the identification of cysteine and tyrosine targets of NO by an in silico analysis, which requires confirmation by further proteomic approaches. Interestingly, some transporters are targets of both ROS- and NO-dependent PTMs, suggesting that these reactive species are an important hub in the regulation of their functionality. Additionally, the specific subcellular sites of production of ROS and NO could trigger specific patterns of PTM and signaling, which require further deep analysis. All this information could be of interest in designing new strategies to fortify crops, improving plant resilience against nutritional imbalance and salinity, and designing new phytoremediation methodologies based on redox biochemistry governed by ROS and NO.
Supplementary data
The following supplementary data are available at JXB online.
Table S1. Prediction of S-nitrosylation and Y nitration sites of Arabidopsis metal transporters.
Conflict of interest
The authors declare no conflict of interest.
Funding
LMS, JE, and MCRP were supported by grants P20_00364 from the Junta de Andalucía, and PID2021-122280NB-I00 and RED2018-102407-T from the Ministry of Science, and Innovation and Universities, the ‘Agencia Estatal de Investigación’ and the European Regional Development Fund co-funding (MCIU/AEI/ERDF). JE was supported by a fellowship for academic staff from the Junta de Andalucía (PREDOC_00917). JL was supported by grant PID2020-112618GB-I00 from the Spanish Ministry of Economy and Competitiveness and ‘Agencia Estatal de Investigación’/FEDER/European Union. SS acknowledges support from Australian Research Council and grant 31961143001 for joint research projects between the Pakistan Science Foundation and National Natural Science Foundation of China.
Comments