-
PDF
- Split View
-
Views
-
Cite
Cite
Feng Peng, Chenyang Li, Chengkai Lu, Yang Li, Peng Xu, Gang Liang, IRONMAN peptide interacts with OsHRZ1 and OsHRZ2 to maintain Fe homeostasis in rice, Journal of Experimental Botany, Volume 73, Issue 18, 18 October 2022, Pages 6463–6474, https://doi.org/10.1093/jxb/erac299
- Share Icon Share
Abstract
IRONMAN (IMA) is a family of small peptides which positively regulate plant responses under Fe deficiency. However, the molecular mechanism by which OsIMA1 and OsIMA2 regulate Fe homeostasis in rice is unclear. Here, we reveal that OsIMA1 and OsIMA2 interact with the potential Fe sensors, OsHRZ1 (HAEMERYTHRIN MOTIF-CONTAINING REALLY INTERESTING NEW GENE (RING) AND ZINC-FINGER PROTEIN 1) and OsHRZ2. OsIMA1 and OsIMA2 contain a conserved 17 amino acid C-terminal region which is responsible for the interactions with OsHRZ1 and OsHRZ2. Plants overexpressing OsIMA1 (OsIMA1ox) show increased Fe concentration in seeds and reduced fertility, as observed in the hrz1-2 loss-of-function mutant plants. Moreover, the expression patterns of Fe deficiency inducible genes in the OsIMA1ox plants are the same as those in hrz1-2. Co-expression assays suggest that OsHRZ1 and OsHRZ2 promote the degradation of OsIMA1 proteins. As the interaction partners of OsHRZ1, the OsPRI (POSITIVE REGULATOR OF IRON HOMEOSTASIS) proteins also interact with OsHRZ2. The conserved C-terminal region of four OsPRIs contributes to the interactions with OsHRZ1 and OsHRZ2. An artificial IMA (aIMA) derived from the C-terminal of OsPRI1 can be also degraded by OsHRZ1. Moreover, aIMA overexpressing rice plants accumulate more Fe without reduction of fertility. This work establishes the link between OsIMAs and OsHRZs, and develops a new strategy for Fe fortification in rice.
Introduction
Iron (Fe) is one of the essential micronutrients for all living organisms, since Fe can mediate redox reactions in the electron transport chain through the conversion between ferrous and ferric forms, which takes part in various cellular biochemical processes (Hänsch and Mendel, 2009). Fe deficiency is one of the most prevalent human nutritional disorders worldwide (Mayer et al., 2008). Since humans obtain Fe mainly from plants, the production of Fe-rich crops will profit human health. Fe deficiency in plants often results in interveinal chlorosis of leaves, growth retardation and reduced crop yields (Briat et al., 2015). Despite Fe being abundant in the earth’s crust, bioavailability of Fe is low, as it is mainly present in the forms of insoluble hydroxides and oxides, especially in calcareous soils. One third of the world’s cultivated lands are calcareous soils. Thus, Fe deficiency has become one of the factors limiting plant quality and productivity around the world.
To cope with Fe deficiency stress, plants have evolved two distinct strategies for efficient Fe uptake, the reduction strategy (strategy I) and the chelation strategy (strategy II; Römheld and Marschner, 1986). Generally, strategy I, which is mainly utilized in non-graminaceous plants, involves the acidification of the rhizosphere to release Fe, the reduction of Fe (III) to Fe (II) and the transport of Fe (II) (Marschner, 1995; Eide et al., 1996; Robinson et al., 1999). Strategy I plants also secrete flavins and coumarins to promote Fe uptake (Sisó-Terraza et al., 2016; Tsai et al., 2018). Strategy II, which is employed by graminaceous plants, is mediated by the synthesis and secretion of Fe (III) chelators, the mugineic acid (MA) family, and the translocation of MA-Fe (III) into roots. Rice (Oryza sativa) is a specific graminaceous species, which preferentially grows in the waterlogged field. Rice not only possesses the strategy II-based Fe-uptake system which includes the MA synthesis-associated enzymes [e.g. OsNAS1(NICOTIANAMINE SYNTHASE 1), OsNAS2, OsNAAT1 (NICOTIANAMINE AMINOTRANSFERASE 1), OsDMAS1 (DEOXYMUGINEIC ACID SYNTHASE 1), etc.], the MA excretion protein [e.g. OsTOM1 (TRANSPORTER OF MUGINEIC ACID FAMILY PHYTOSIDEROPHORES 1)] and the MA-Fe (III) transporter [e.g. OsYSL15 (YS-Like15)], but also partial strategy I Fe uptake system which involves the Fe (II) transporter OsIRT1 (IRON TRANSPORTER 1) and OsIRT2 (Ishimaru et al., 2006; Cheng et al., 2007).
Fe deficiency directly restricts growth and development of plants, while excessive iron causes the formation of cytotoxic reactive oxygen species (ROS) and damages cellular constituents (Brumbarova and Bauer, 2005). To maintain Fe homeostasis, plants have developed a sophisticated signalling network to regulate Fe uptake and transport. The basic helix-loop-helix (bHLH) family plays a key role in the maintenance of Fe homeostasis in rice (Kobayashi, 2019). OsIRO2 (IRON-RELATED BHLH TRANSCRIPTION FACTOR 2) functions as a crucial regulator of Fe homeostasis (Ogo et al., 2007), which is in charge of the expression of strategy II associated genes OsNAS1, OsNAS2, OsNAAT1, OsTOM1 and OsYSL15 (Ogo et al., 2011; Liang et al., 2020). OsFIT (FER LIKE FE DEFICIENCY INDUCED TRANSCRIPTION FACTOR)/OsbHLH156 is an interaction partner of OsIRO2. Unlike OsIRO2, which is preferentially localized in the cytoplasm, OsFIT mainly localizes in the nucleus. In the presence of OsFIT, OsIRO2 moves to the nucleus where OsFIT and OsIRO2 form a transcription complex to activate the expression of Fe-uptake genes (Wang et al., 2019; Liang et al., 2020). The transcription of OsFIT and OsIRO2 increases under Fe-deficient conditions, but decreases under Fe-sufficient conditions. OsIRO3 is a negative regulator of Fe homeostasis (Zheng et al., 2010; F. Wang et al., 2020; W. Wang et al., 2020), and the loss-of-function of OsIRO3 causes up-regulation of OsFIT and OsIRO2 (Li et al., 2022). In contrast, OsPRI1 (POSITIVE REGULATOR OF IRON HOMEOSTASIS 1)/OsbHLH060, OsPRI2/bHLH058, and OsPRI3/OsbHLH059 positively regulate the expression of OsIRO2 and OsFIT (Zhang et al., 2017, 2020; Kobayashi et al., 2019). OsHRZ1 [HAEMERYTHRIN MOTIF-CONTAINING REALLY INTERESTING NEW GENE (RING) AND ZINC-FINGER PROTEIN 1] and OsHRZ2 have been identified as potential Fe sensors, which contain several hemerythrin domains for Fe binding and a RING domain for E3 ligase activity, playing a negative role in Fe homeostasis (Kobayashi et al., 2013). OsHRZ1 interacts with OsPRI1, OsPRI2, and OsPRI3 and mediates their degradation via the 26S proteasome pathway (Zhang et al., 2017, 2020). In addition, OsIDEF1 (IDE-BINDING FACTOR 1) and OsIDEF2 were reported to regulate the early Fe deficiency response and the internal Fe translocation, respectively (Ogo et al., 2008; Kobayashi et al., 2009).
IMA (IRONMAN), a family of small peptides, has been recently reported to play a positive role in the Fe deficiency response in Arabidopsis and rice (Grillet et al., 2018; Hirayama et al., 2018; Kobayashi et al., 2021; Li et al., 2021). Two OsIMA genes have been identified in rice (Grillet et al., 2018; Kobayashi et al., 2021). The expression of both genes is positively regulated by OsPRI2 and OsPRI3, and negatively regulated by OsIRO3 (F. Wang et al., 2020). However, it was still unclear how OsIMA1 and OsIMA2 activate the Fe deficiency response in rice.
In the present study, we show that OsIMA1 and OsIMA2 physically interact with OsHRZ1 and OsHRZ2, and their protein stability is under the control of OsHRZs. Correspondingly, OsIMA1 overexpression causes the phenotypes similar to those of hrz1-2 mutant plants. Furthermore, the overexpression of an artificial IMA derived from OsPRI1 promotes Fe accumulation in seeds, but does not lower fertility.
Materials and methods
Plant materials and growth conditions
Rice (Oryza sativa L. ‘Nipponbare’) was used as wild type in this study. Rice plants were grown in Crops Conservation and Breeding Base of XTBG, in Mengla county of Yunnan province, China. For hydroponic culture assays, half-strength Murashige and Skoog (MS) media (pH5.6–5.8) with 0.1 mM EDTA-Fe (III) was used. The nutrient solution was changed every 3 d. Rice plants were grown in a growth chamber at 28 °C during the day (14 h, light intensity of 300 μmol m-2 s-1) and at 20 °C during the night (10 h).
For paddy field growth, pre-germinated seeds were planted with a planting distance of 18 × 18 cm. The soil (pH 6.65) contained 44.93 g kg-1 total Fe. During the whole growing period, the paddy field was maintained with 5–15 cm standing water until the plants reached the late grain filling stage. Fertilizers (nitrogen, phosphorus, and potassium) were applied at normal rates for paddy rice in the region.
Nicotiana benthamiana plants were grown in a growth chamber at 22 °C during the day (14 h, light intensity of 120 μmol m-2 s-1) and at 20 °C during the night (10 h).
qRT–PCR
Total RNA was extracted from 3-week-old rice roots or shoots. RNA samples were reverse transcribed using an RT Primer Mix (oligo dT) and PrimeScript RT Enzyme Mix for qPCR (TaKaRa, Japan) following the manufacturer’s protocol. qRT–PCR was performed on a Light-Cycler 480 real-time PCR machine (Roche, Switzerland) by using PrimeScript™ RT reagent (Perfect Real Time) Kit (TaKaRa, Japan). All PCR amplifications were performed in triplicate, with OsACTIN1 and OsOBP as the internal controls to normalize the samples. Primer sequences used for qRT–PCR are listed in Supplementary Table S1.
Metal concentration measurement
Seeds from paddy field grown plants and shoots and roots from hydroponically grown plants were dried at 65 °C for one week. About 500 mg dry weight of these samples were digested with 5 ml of 11 M HNO3 for 3 h at 185 °C and 2 ml of 12 M HClO4 for 30 min at 220 °C. The concentration of Fe was measured with an Inductively Coupled Plasma Mass Spectrometry (ICP-MS, Japan). All measurements were done in three biological replicates (n =3).
Yeast two-hybrid assays
The yeast two-hybrid assays were carried out according to the manufacturer’s protocol. Root cDNA was used for the amplification of various genes or regions. The C-terminal regions of OsHRZ1 and OsHRZ2 were cloned into the pGADT7 or pGBKT7 plasmids, respectively (Clontech, Japan). The full-length or truncated OsIMAs were cloned individually into the pGBKT7 plasmid. The GAD-PRIs vectors were described previously (Zhang et al., 2020). Vectors were transformed into yeast strain Y2HGold (Clontech, Japan). Yeast transformation was performed according to the Yeastmaker Yeast Transformation System 2 User Manual (Clontech, Japan). The primers used are listed in Supplementary Table S1.
Generation of transgenic plants
Root cDNA was used for the amplification of various genes or regions. For generation of the OsIMA1 overexpression construct, the full-length CDS of OsIMA1 was cloned downstream of the maize ubiquitin promoter in the pUN1301 binary vector (Biovector, USA). The C-terminal region of OsPRI1 was designed as the aIMA and cloned downstream of the maize ubiquitin promoter in the pUN1301 binary vector. The constructs were introduced into the Agrobacterium tumefaciens strain EHA105 and then used for transformation in wild-type rice by Biogle Biotechnology Corporation (Hangzhou, China). The transgenic plants were selected with hygromycin and used for examination of transgene expression. T3 transgenic plants were used for analysis.
Tripartite split-GFP complementation assays
Tripartite split-GFP complementation assays were conducted as reported previously (Lei et al., 2020). The sfGFP was divided into three parts, GFP1-9, GFP10 and GFP11. The N-end of OsIMAs was fused with GFP10, and the C-end of OsHRZs with GFP11. A. tumefaciens strain EHA105 was used in the transient expression experiments. Each of the above constructs was transformed into Agrobacterium cells, and various combinations of Agrobacterium cells were infiltrated into 3-week-old Nicotiana benthamiana leaves by an infiltration buffer (0.2 mM acetosyringone, 10 mM MgCl2, and 10 mM MES, pH 5.6). The abaxial sides of leaves were injected with 20 μM β-estradiol 24 h before observation. GFP fluorescence was photographed on an OLYMPUS confocal microscope (OLYMPUS, Japan).
Firefly luciferase (LUC)-based bimolecular fluorescence complementation (BiFC) assays
The N-end of OsIMAs was fused with cLUC, and the C-end of OsHRZs with nLUC. The combinations indicated were introduced into Agrobacterium and co-expressed in N. benthamiana leaves. The various combinations of Agrobacterium cells were infiltrated into 3-week-old Nicotiana benthamiana leaves by an infiltration buffer (0.2 mM acetosyringone, 10 mM MgCl2, and 10 mM MES, pH 5.6). The plants were placed in the dark for 48 h. Then, the infiltrated leaves were sprayed with luciferin (100 mM) and kept in the dark for 10 min, and photographed by a low-light cooled CCD imaging apparatus (Tanon-5200, Tannon, China).
Protein transient expression in tobacco leaves
OsHRZ1 and OsHRZ2 were fused with the C-end of GFP, and driven by the CaMV 35S promoter in the binary vector pOCA30. OsIMA1, OsIMA2, and OsIMA1Ala54Val were fused with the N-terminal of mCherry, and driven by the CaMV 35S promoter in the binary vector pOCA30. These plasmids were transformed into Agrobacterium cells individually. Various combinations of Agrobacterium cells were infiltrated into N. benthamiana leaves, and kept in the dark for 48 h. Then, the infiltrated leaves were used for protein extraction with RIPA buffer [50 mM Tris, 150 mM NaCl, 1% NP-40, 0.5% Sodium deoxycholate, 0.1% SDS, 1 mM PMSF, 1 × protease inhibitor cocktail, pH 8.0] and immunoblotting was conducted.
Immunoblotting
Proteins were electrophoresed using 12% SDS-PAGE and transferred to nitrocellulose membranes. Target proteins on the membrane were detected using immunodetection and chemiluminescence. Signals on the membrane were recorded using a chemiluminescence detection machine (Tanon-5200). The antibodies used for western blotting were as follows, mouse monoclonal anti-GFP (Abmart, China, 1:5000), anti-mCherry (Abmart, China, 1:5000)) and goat anti-rabbit IgG horseradish peroxidase (Affinity Biosciences, USA, 1:10 000).
Results
OsIMA1 and OsIMA2 interact with OsHRZ1 and OsHRZ2
OsIMA1 and OsIMA2 play positive roles in Fe homeostasis since their overexpression promotes the expression of Fe deficiency-inducible genes (Kobayashi et al., 2021). It was reported that Arabidopsis IMAs physically interact with the C-terminal region of BRUTUS (BTS; Li et al., 2021) which is an ortholog of OsHRZ1 (Kobayashi et al., 2013). OsHRZ2 is a paralog of OsHRZ1 in rice. To verify whether OsIMA1 and OsIMA2 interact with OsHRZ1 and OsHRZ2, we carried out yeast-two-hybrid assays. OsIMA1 and OsIMA2 were fused with the GAL4 DNA binding domain (BD) in the pGBKT7 vector as the baits, and the C-terminal regions of OsHRZ1 and OsHRZ2 with the GAL4 activation domain (AD) in the pGADT7 vector as the preys. As shown in the growth of yeast, both OsIMA1 and OsIMA2 interact with the C-terminal regions of OsHRZ1 and OsHRZ2 (Fig. 1A).
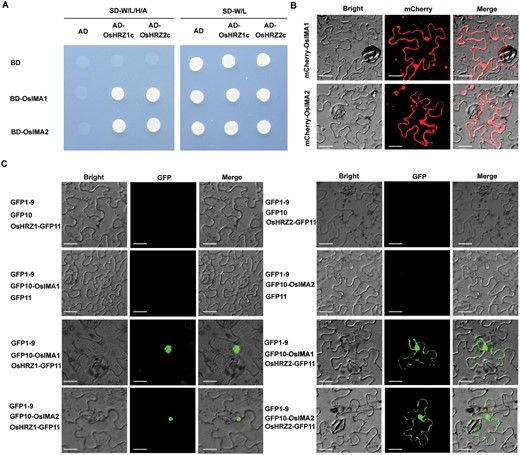
OsIMA1 and OsIMA2 interact with OsHRZ1 and OsHRZ2. (A) OsIMAs interact with the C-terminal regions of OsHRZs in yeast. The full-length OsIMAs were fused with BD, and the C-terminal regions of OsHRZs fused with AD. Yeast co-transformed with different BD and AD plasmid combinations was spotted. Growth on selective plates lacking leucine, tryptophan, adenine, and histidine or lacking leucine and tryptophan is shown. (B) Sub-cellular localization of OsIMA1 and OsIMA2. mCherry was fused with the N-end of OsIMAs. Transient expression assays were performed in N. benthamiana leaves. Scale bars=20 μm. (C) Interaction of OsIMAs and OsHRZs in plant cells. Tripartite split-sfGFP complementation assays were performed. OsIMAs were fused with GFP10, and OsHRZs with GFP11. The combinations indicated were introduced into Agrobacterium and co-expressed in N. benthamiana leaves. Scale bars=20 μm.
OsHRZ1 protein localizes in the nucleus, and OsHRZ2 in both the nucleus and cytoplasm (Kobayashi et al., 2013). To investigate the sub-cellular localization of OsIMA1 and OsIMA2, mCherry was tagged to the N-end of OsIMA1 and OsIMA2, and expressed transiently in tobacco leaves. As shown in Fig. 1B, both OsIMA1 and OsIMA2 were present in the nucleus and cytoplasm. To further confirm the location where the protein interactions occur, we employed the tripartite split-GFP system monitoring the localization of protein complexes. The GFP10 fragment was fused with OsIMA proteins in their N-end (GFP10-OsIMAs), and GFP11 with OsHRZs in their C-end (OsHRZs-GFP11). When GFP10-OsIMA1/2 and OsHRZ1-GFP11 were transiently co-expressed with GFP1-9 in tobacco leaves, the GFP signal was only visible in the nucleus of transformed cells (Fig. 1C). By contrast, when GFP10-OsIMA1/2 and OsHRZ2-GFP11 were transiently co-expressed with GFP1-9, the GFP signal was visible in both the nucleus and cytoplasm. We also employed the LUC-based BiFC assay to confirm the protein interactions (Supplementary Fig. S1). Taken together, our data suggest that OsIMAs physically interact with OsHRZs in plant cells.
The C-terminal region of OsIMAs accounts for the interactions with OsHRZ1 and OsHRZ2
The IMAs feature a conserved C-terminal region (Fig. 2A; Grillet et al., 2018). In order to investigate whether the C-terminal region is responsible for their interactions with OsHRZs, we performed yeast-two-hybrid assays. OsIMA peptides were divided into two parts, the N-terminal region and the C-terminal 17 aa region, and individually fused to BD in the pGBK-T7 vector as baits. Yeast growth assays indicated that their C-terminal regions, but not the N-terminal regions, interact with OsHRZs (Fig. 2B).
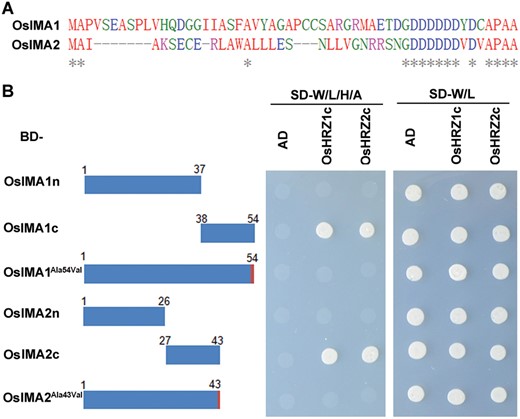
The last amino acid of OsIMA1 and OsIMA2 is crucial for interactions with OsHRZ1 and OsHRZ2. (A) Alignment of amino acid sequences of OsIMAs. The full-length amino acid sequences of OsIMAs were aligned using Clustal Omega online (https://www.ebi.ac.uk/Tools/msa/clustalo/). The asterisks indicate identical amino acids. (B) Yeast-two-hybrid assays. The left panel shows a schematic diagram of various versions of OsIMA. OsIMA1/2n, the N-terminal of OsIMA1/2; OsIMA1/2c, the C-terminal of OsIMA1/2; OsIMA1Ala54Val and OsIMA2Ala43Val, the last amino acid Ala of OsIMA1/2 was changed to Val. The truncated or mutated OsIMAs were fused with BD, and the C-terminal regions of OsHRZs with AD. Yeast co-transformed with different BD and AD plasmid combinations was spotted. Growth on selective plates lacking leucine, tryptophan, adenine, and histidine or lacking leucine and tryptophan is shown.
The last amino acid Ala of Arabidopsis IMAs is crucial for their interactions with BTS (Li et al., 2021). The last amino acid of OsIMAs is also Ala. We questioned whether the same case occurs in rice. We generated the full-length OsIMAs with their last amino acid changed from Ala to Val and fused them with the BD as baits. Yeast growth indicated that the mutation of the last amino acid Ala disrupted the interactions between OsIMAs and OsHRZs (Fig. 2B). These data suggest that the C-terminal regions of OsIMAs contribute to their interactions with OsHRZs, and that the last amino acid Ala is necessary.
OsIMA1ox plants mimic the hrz1-2 mutant plants
To further investigate how OsIMAs regulate the Fe deficiency response, we generated OsIMA1 overexpressing transgenic plants (OsIMAox) in which expression of the OsIMA1 gene was driven by the maize ubiquitin promoter (Supplementary Fig. S2). The OsIMA1 transgenic plants displayed reduced fertility compared with the wild type, which was also observed in the hrz1-2 loss-of-function mutant plants (Fig. 3A; Supplementary Fig. S3A). In contrast, thousand-kernel weight of OsIMAox plants was similar to that of wild-type and hrz1-2 plants (Supplementary Fig. S3B). Measurement of metal concentration indicated that the OsIMA1ox plants accumulated much more Fe in the seeds than the wild-type plants, as did the hrz1-2 mutant plants (Fig. 3B). There was no difference for seed Zn and Mn concentration amongst OsIMA1ox, hrz1-2 and wild type plants (Supplementary Fig. S3C, D).
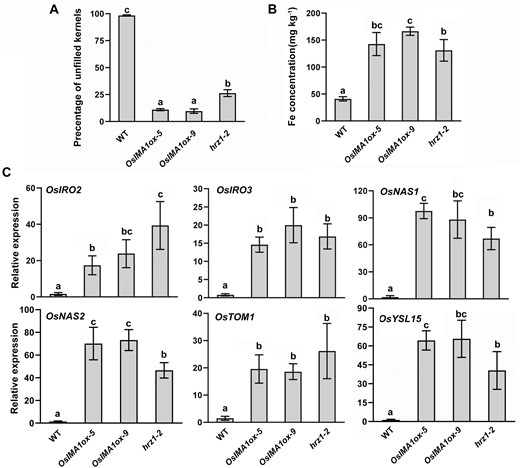
OsIMA1ox plants mimic the hrz1-2 loss-of-function mutant. (A) Seed setting (%) of wild type, OsIMAox and hrz1-2. (B) Fe concentration in seeds of wild type, OsIMAox and hrz1-2. Unpolished seeds were used for Fe measurement. (C) Expression of Fe deficiency-inducible genes in roots of wild type, OsIMAox and hrz1-2. Rice plants were grown in Fe-sufficient solution for 2 weeks, and roots were used for RNA extraction and qRT–PCR. Data represent means ±SD (n=3). Different letters above each bar indicate statistically significant differences (ANOVA, P<0.05).
We then determined their growth in response to Fe deficiency. Under Fe sufficient conditions, OsIMAox and hrz1-2 plants grew as well as wild type. However, under Fe-deficient conditions, OsIMAox and hrz1-2 plants developed higher shoots than wild type (Supplementary Fig. S4A, B). We further measured the metal concentration in the roots and shoots, and found that OsIMAox and hrz1-2 accumulated more Fe, but not Mn or Zn, than wild type (Supplementary Fig. S4C, D). Considering that the OsIMA1ox plants phenocopied the hrz1-2 mutant plants, we then compared the expression of several Fe deficiency-inducible genes. In the Fe deficiency response signalling pathway, OsIRO3 and OsIRO2 are genes encoding two major transcription factors which are considerably up-regulated under Fe-deficient conditions (Ogo et al., 2007; Zheng et al., 2010). Additionally, the strategy II associated genes, such OsNAS1, OsNAS2, OsTOM1, and OsYSL15, are strongly induced by Fe deficiency. Rice plants were grown in Fe-sufficient solution for 2 weeks, and then their roots were separated and used for RNA extraction. Examination of transcript abundance indicated that the expression of these Fe deficiency-inducible genes was significantly up-regulated (P<0.05) in both the OsIMA1ox plants and hrz1-2 mutant plants, compared with the wild-type plants (Fig. 3C).
OsHRZ1 and OsHRZ2 promote the degradation of OsIMAs
OsHRZ1 and OsHRZ2 have a RING domain which possesses E3 ligase activity, and several proteins have been reported to be degraded by OsHRZ1 and OsHRZ2 (Zhang et al., 2017, 2020; Guo et al., 2022). To investigate whether OsHRZ1 and OsHRZ2 also facilitate the degradation of OsIMAs, we used OsIMA1 as a representative and performed the transient expression assays in tobacco leaves. The C-end of OsHRZ1 and OsHRZ2 was fused with the GFP tag. mCherry-OsIMA1 was co-expressed with GFP, OsHRZ1-GFP, and OsHRZ2-GFP separately, in tobacco leaves. Immunoblot analysis indicated that the abundance of mCherry-OsIMA1 protein was markedly lower in the presence of OsHRZ1-GFP or OsHRZ2-GFP, compared with that in the presence of myc-GFP (Fig. 4). These data suggest that OsHRZ1 and OsHRZ2 accelerate the degradation of OsIMA1 and OsIMA2.
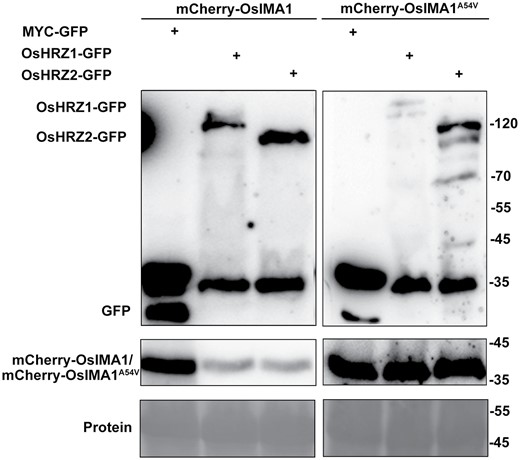
Both OsHRZ1 and OsHRZ2 promote the degradation of OsIMA1. mCherry-OsIMA1 or mCheryy-OsIMA1Ala54Val was co-expressed with MYC-GFP, OsHRZ1-GFP, and OsHRZ2-GFP. Transient expression assays were performed in tobacco leaves. After infiltration with agrobacteria, plants were kept in dark for 2 d, and then infiltrated leaves were harvested. Total protein was extracted and immunoblotted with anti-GFP antibody or anti-mCherry antibodies. Ponceau staining shows equal loading. Protein molecular weights (in kDa) are indicated.
Given that the last amino acid of OsIMA1 and OsIMA2 is responsible for their interactions with OsHRZ1 and OsHRZ2 (Fig. 2B), we speculated that the protein stability of OsIMA1Ala54Val would not be affected by OsHRZ1 and OsHRZ2. The mCherry tag was linked with the N-end of OsIMA1Ala4Val, and then used for co-expression assays. As expected, OsHRZ1 and OsHRZ2 could not degrade OsIMA1Ala54Val (Fig. 4). These data suggest that OsHRZ1 and OsHRZ2 degrade OsIMAs via protein interactions.
The C-terminal region of OsPRI1 interacts with OsHRZ1 and OsHRZ2
The rice bHLH IVc sub-group consists of four members, and three of them (OsPRI1, OsPRI2, and OsPRI3) interact with OsHRZ1 (Zhang et al., 2017, 2020). To verify if the fourth member, OsPRI4, also interacts with OsHRZ1, we tested their interaction using the yeast-two-hybrid system. Yeast growth indicated that OsPRI4 and OsHRZ1 interact with each other. Because OsHRZ2 is a paralog of OsHRZ1, we examined whether OsHRZ2 is also an interaction partner of these four OsPRI proteins. Protein interaction tests indicated that these four OsPRI proteins also interact with OsHRZ2 (Fig. 5A).

The C-terminal region of OsPRIs interacts with OsHRZs. (A) All four OsPRI proteins interact with both OsHRZ1 and OsHRZ2. The full-length OsPRIs were fused with AD, and the C-terminal regions of OsHRZs with BD. Yeast co-transformed with different BD and AD plasmid combinations was spotted. Growth on selective plates lacking leucine, tryptophan, adenine, and histidine or lacking leucine and tryptophan is shown. (B) The C-terminal regions of bHLH IVc proteins from different plants. bHLH IVc proteins in Arabidopsis thaliana, Oryza sativa, Zea mays, and Sorghum bicolor, were used for analysis. Their C-terminal regions were aligned using Clustal Omega online (https://www.ebi.ac.uk/Tools/msa/clustalo/). AtbHLH34 (AT3G23210), AtbHLH104 (AT4G14410), AtbHLH105 (AT5G54680), AtbHLH115 (AT1G51070), OsPRI1 (LOC_Os08g04390), OsPRI2 (LOC_Os05g38140), OsPRI3 (LOC_Os02g02480), OsPRI4 (LOC_Os07g35870), ZmIVc-1 (ZmPHJ40.04G070000), ZmIVc-2 (ZmPHJ40.07G193300), ZmIVc-3 (ZmPHJ40.04G350300), ZmIVc-4 (ZmPHJ40.05G151400), ZmIVc-5 (ZmPHJ40.06G183000), SbIVc-1 (SbiSC187.07G031300), SbIVc-2(SbiSC187.02G307200), SbIVc-3(SbiSC187.04G009900), SbIVc-4 (SbiSC187.09G150000). The asterisks indicate the identical amino acids. (C) The C-terminal region of OsPRI1 interacts with OsHRZ1 and OsHRZ2. The left panel shows a schematic diagram of various versions of OsIMA. The truncated or mutated OsPRI1 were fused with AD, and the C-terminal regions of OsHRZs with BD. OsPRI1n, the N-terminal of OsPRI1; OsPRI1m, the bHLH domain of OsPRI1; OsPRI1c, the C-terminal of OsPRI1; OsPRI1Ala253Val, the last amino acid Ala of OsPRI1 was changed to Val. Yeast co-transformed with different BD and AD plasmid combinations was spotted. Growth on selective plates lacking leucine, tryptophan, adenine, and histidine or lacking leucine and tryptophan is shown.
The alignment of four OsPRI proteins shows that they share a conserved C-terminal region (Fig. 5B). We then investigated whether their interactions with OsHRZ1 and OsHRZ2 depend on their C-terminal regions. Considering the high similarity of their C-terminal regions of bHLH IVc proteins across different plant species (Fig. 5B), OsPRI1 was chosen as a representative for further analysis. OsPRI1 was divided into three parts, the N-terminal (OsPRI1n), the bHLH domain (OsPRI1m), and the C-terminal (OsPRI1c), and fused with the AD. OsHRZ1c and OsHRZ2c were fused with the BD. Interaction tests indicated that only OsPRI1c could interact with OsHRZ1c and OsHRZ2c (Fig. 5C). To further investigate whether the last amino acid Ala of OsPRI1 is crucial for the interactions, Ala was substituted for Val in the full-length construct of OsPRI1. The results demonstrated that the substitution disrupted the interactions with OsHRZ1c and OsHRZ2c (Fig. 5C). These data suggest that the C-terminal region of OsPRIs is required for the interactions with OsHRZ1 and OsHRZ2.
Generation of Fe-fortified rice grains by manipulating an artificial IMA derived from OsPRI1
The overexpression of OsIMA1 caused Fe overaccumulation in grains, but reduced fertility (Fig. 3A, B). Because the reduction of fertility is a disadvantageous factor for yield, we attempted to develop an artificial IMA which would increase seed Fe concentrations, but not reduce fertility. Given that the C-terminal region of OsPRIs also interacts with OsHRZs, the C-terminal region of OsPRI1 was designed as an artificial IMA (aIMA; Fig. 6A). Having confirmed that aIMA interacts with OsHRZ1 and OsHRZ2 (Fig. 5C), we investigated whether aIMA could be degraded by OsHRZ1 and OsHRZ2. The mCherry tag was fused to the N-end of aIMA and used for transient co-expression assays in tobacco leaves. Compared with the GFP, both OsHRZ1-GFP and OsHRZ2-GFP caused the degradation of mCherry-aIMA proteins, suggesting that OsHRZ1 and OsHRZ2 favour the degradation of aIMA (Fig. 6B). To further investigate whether aIMA can increase Fe accumulation in seeds, we generated transgenic rice overexpressing aIMA (aIMAox; Supplementary Fig. S5A, B). We did not observe visible fertility differences between wild-type and aIMAox plants (Fig. 6C; Supplementary Fig. S6A). Thousand-kernel weight of aIMAox plants was comparable with that of wild type (Supplementary Fig. S6B). Then, we determined the metal concentration of unpolished seeds, finding that the Fe concentration was around 2-fold higher in the aIMAox plants than in the wild type (Fig. 6D). In contrast, the seed Zn and Mn concentration of aIMAox plants was similar to wild type (Supplementary Fig. S6C, D). When grown in Fe-deficient liquid medium, the shoots of aIMAox plants were taller than that of wild type (Supplementary Fig. S7A, B). Metal concentration analysis indicated that Fe concentrations in shoots of aIMAox plants were higher than in the wild type under Fe-sufficient conditions (Supplementary Fig. S7C), whereas Fe concentrations were higher in roots under Fe-deficient conditions (Supplementary Fig. S7D). Taken together, our data suggest that the artificial IMA strategy is effective in improving Fe concentrations of rice grains.
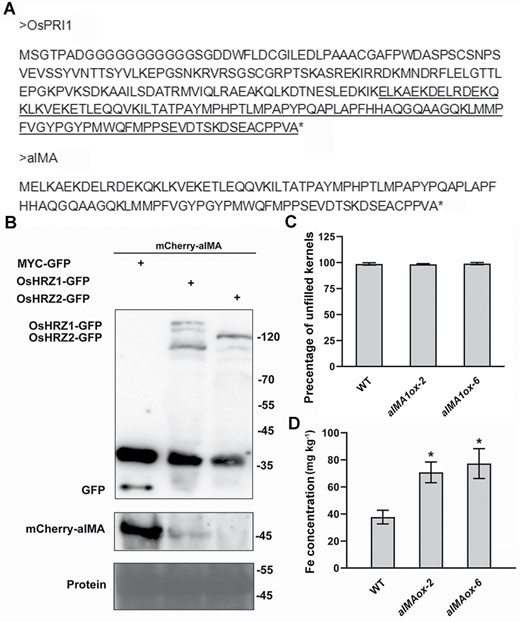
Overexpression of aIMA causes Fe overaccumulation in seeds. (A) Protein sequence of aIMA. The C-terminal region of OsPRI1 (the underlined sequence) was used to generate aIMA. A translation start site (M) was added. (B) Degradation of aIMA by OsHRZs. mCherry-aIMA was co-expressed with MYC-GFP, OsHRZ1-GFP, and OsHRZ2-GFP. Transient expression assays were performed in tobacco leaves. After infiltration with agrobacteria, plants were kept in dark for 2 d, and then infiltrated leaves were harvested. Total protein was extracted and immunoblotted with anti-GFP antibody or anti-mCherry antibody. Ponceau staining shows equal loading. Protein molecular weights (in kDa) are indicated. (C) Percentage of unfilled kernels of wild type and aIMAox. Data represent means ±SD (n=3). (D) Fe concentration. Unpolished seeds were used for Fe measurement. Data represent means ±SD (n=3). The asterisks indicate significant differences from the wild type (WT) as determined by Student’s t-test (P<0.05).
Discussion
Recently, tremendous progress has been made in the Fe deficiency response signalling pathway in rice. Many transcription factors, especially bHLH proteins, play key roles in Fe signal transduction (Kobayashi, 2019). OsHRZ1 and OsHRZ2 are two potential Fe sensors which negatively regulate Fe homeostasis (Kobayashi et al., 2013). IMAs are a class of small peptides which are conserved across angiosperms (Grillet et al., 2018). Two OsIMAs exist in rice, and their overexpression causes the constitutive activation of Fe deficiency-inducible genes (Kobayashi et al., 2021). However, the underlying molecular mechanism by which OsIMA1 and OsIMA2 mediate Fe signalling remains to be clarified. Here, we suggest that OsIMAs and OsHRZs antagonistically regulate the Fe deficiency response. Based on the regulatory mechanism of OsIMAs, we developed a strategy for generation of Fe fortified rice by manipulating an artificial IMA peptide.
OsHRZ1 and OsHRZ2 contain 1-3 haemerythrin domains and one RING domain, which endow them the Fe binding ability and E3 ligase activity, respectively (Kobayashi et al., 2013). Here, we revealed that OsHRZ1 and OsHRZ2 interact with OsIMAs and accelerate the degradation of OsIMAs. Recently, it was reported that OsHRZ1 interacts with, and mediates the degradation of three bHLH IVc proteins, OsPRI1, OsPRI2, and OsPRI3 (Zhang et al., 2017, 2020). Here, we further confirmed that all four bHLH IVc members interact with both OsHRZ1 and OsHRZ2 (Fig. 5A). Given that both OsHRZ1 and OsHRZ2 have E3 ligase activity and degrade their substrates (Kobayashi et al., 2013; Zhang et al., 2017; Guo et al., 2022), it is very likely that OsHRZ2 also mediates the degradation of bHLH IVc proteins. Although bHLH IVc proteins are positive regulators of the Fe deficiency response, their transcripts are barely induced by Fe deficiency (Zhang et al., 2017, 2020; Kobayashi et al., 2019). Thus, it is expected that their protein levels increase in response to Fe deficiency. It is noteworthy that the transcript abundance of OsHRZ1 also increased under Fe deficiency (Kobayashi et al., 2013). In order to maintain stable levels of bHLH IVc proteins, the protein stability or activity of OsHRZ1 must be reduced under Fe-deficient conditions. Although OsHRZ1 and OsHRZ2 can bind Fe ions, their protein stability seems to not respond to Fe status, according to the cell free degradation assays in vitro (Kobayashi et al., 2013). A recent study found that OsHRZ2 protein increases in response to Fe deficiency, as shown in the OsHRZ2-GFP overexpressing transgenic plants (Guo et al., 2022). BTS is a homolog of OsHRZ1 in Arabidopsis, and its protein stability is enhanced in the absence of Fe, as shown in the protein translation in wheat germ extracts (Selote et al., 2015). Thus, the protein stability of OsHRZ1 and OsHRZ2 is either irrespective to the Fe status, or increased under Fe-deficient conditions. It was an open question as to how rice plants promote the accumulation of bHLH IVc proteins to activate the Fe deficiency response. In Arabidopsis, IMAs interfere with the interactions between bHLH IVc members and BTS, since IMAs and bHLH IVc share a similar C-terminal region which contributes to the interactions with BTS (Li et al., 2021). We indicated that the C-terminal regions of both OsIMAs and OsPRIs contain an OsHRZ-interacting domain, which makes it possible that OsIMAs compete with OsPRIs for interacting with OsHRZs. This hypothesis was further supported by the fact that the OsIMA1ox plants phenocopy the hrz1-2 mutant plants (Fig. 3). Thus, it is very likely that OsIMAs interfere with the interactions between OsPRIs and OsHRZs, hence stabilizing the OsPRI proteins and activating the Fe deficiency response.
The reciprocal regulation between OsIMAs and OsHRZs is crucial for the maintenance of Fe homeostasis. Under Fe-deficient conditions, the transcription of both OsIMAs and OsHRZs is enhanced (Kobayashi et al., 2013, 2021). We show that OsHRZ1 and OsHRZ2 facilitate the degradation of OsIMA1 (Fig. 4). On the other hand, OsIMA1 overexpression enhances the transcription of OsHRZ1, and the RNA interference of OsHRZs promotes the transcription of OsIMA1 and OsIMA2 (Kobayashi et al., 2021). Both the overexpression of OsIMA1 and the loss-of-function of OsHRZ1 cause Fe overaccumulation and reduced fertility in rice (Fig. 3A). The disruption of balance between OsIMA1 and OsHRZ1 results in the disorder of Fe homeostasis and Fe toxicity. The transcript abundance of OsIMAs is relatively low under Fe-sufficient conditions, but extremely high under Fe-deficient conditions (Kobayashi et al., 2021). When OsIMA1 was overexpressed under Fe-sufficient conditions, rice plants accumulated excessive Fe (Fig. 3B; Kobayashi et al., 2021). Therefore, appropriate levels of OsIMA are required for the maintenance of Fe homeostasis. In addition to degradation by OsHRZs, the transcription of OsIMAs is positively regulated by OsPRIs (Kobayashi et al., 2021). The balance between the positive regulators (OsPRIs) and the negative regulators (OsHRZs) maintains the appropriate level of OsIMAs.
Fe-deficiency anaemia is one of the most prevalent human micronutrient deficiencies around the world. Breeding staple crops with abundant Fe is an ideal way to cope with Fe-deficiency anaemia. Fe fortification in rice grains has been accomplished by introducing Fe-homeostasis associated genes (Masuda et al., 2013). In the OsIMA1ox plants, the expression of Fe uptake associated genes increases irrespective of Fe status, which explains the increased Fe accumulation in grains when plants are grown in Fe-sufficient soil. It was reported that Fe overaccumulation is related to the embryo lethality of various bts mutants (Selote et al., 2015). In contrast to the complete infertility of bts null mutants, the bts-1 mutant with the reduced induction of BTS has slight embryo lethality (Selote et al., 2015). It implies that the increased Fe accumulation causes Fe toxicity to embryos, hence reducing fertility. Although the increased Fe accumulation is advantageous for Fe fortification, the reduced fertility is disadvantageous for rice yield. The inhibitory effect of OsIMAs on OsHRZ1 provides an alternative approach to increasing Fe accumulation without reducing yield. We developed an artificial small peptide, aIMA, which possesses the ability to interact with OsHRZs and can be degraded by OsHRZs. Indeed, the increased Fe accumulation and normal fertility were achieved in the transgenic plants overexpressing aIMA peptides (Fig. 6). Unlike the strong increase of Fe concentration in the OsIMAox plants, the moderate Fe increase was detected in the aIMAox plants. The major difference between OsIMAs and aMIA is that OsIMAs are rich in aspartic acid. Grillet et al. (2018) revealed that the aspartic acid stretch contributes to the affinity of IMAs for Fe ions. Since the stability of haemerythrin domain-containing proteins is affected when Fe ions are present (Salahudeen et al., 2009; Vashisht et al., 2009; Selote et al., 2015), it is plausible that OsIMAs deliver Fe ions to OsHRZs and then result in the inactivity of OsHRZs. It might be the reason why OsIMA1 has stronger activation to Fe deficiency-inducible genes than aIMA. This also raises the possibility of further improving Fe accumulation by optimizing the amino acids of aIMA. We noted that the bHLH IVc proteins share the conserved C-terminal region across different plant species (Fig. 5B). Thus, the artificial IMA strategy can be applied to other plant species beyond rice. Our exploration of an artificial IMA peptide provides a new strategy for Fe fortification in crops.
Supplementary data
The following supplementary data are available at JXB online.
Fig. S1. Interaction of OsIMAs and OsHRZs in plant cells.
Fig. S2. Identification of OsIMA1 overexpressing plants.
Fig. S3. Analysis of OsIMAox seeds.
Fig. S4. Analysis of OsIMAox plants grown in liquid medium.
Fig. S5. Generation of aIMA overexpressing plants.
Fig. S6. Analysis of aIMAox seeds.
Fig. S7. Analysis of aIMAox plants grown in liquid medium.
Table S1. Primer sequences used for qRT–PCR.
Acknowledgements
We thank the Institutional Center for Shared Technologies and Facilities of Xishuangbanna Tropical Botanical Garden, CAS, China, for assistance in the determination of metal content. We also thank the Germplasm Bank of Wild Species in Southwest China for confocal laser scanning microscopy, and Crops Conservation and Breeding Base of XTBG, CAS, China, for rice planting.
Author contributions
GL designed the experiments; FP, CYL, CKL, and YL performed the experiments; FP, CYL, CKL, YL, PX, and GL analysed the data; FP and GL wrote the manuscript; all the authors read and approved the final manuscript.
Conflict of interest
The authors have no conflicts to declare.
Funding
This work was supported by the Special Plan for Key Laboratory of ‘Western Light Western cross team’ (xbzg-zdsys-202111) and the Applied Basic Research Project of Yunnan Province (202001AT070131 and 202003AD150007).
Data availability
The data supporting the findings of this study are available from the corresponding author (GL) upon request.
References
Author notes
These authors contribute equally to this work.
Comments