-
PDF
- Split View
-
Views
-
Cite
Cite
Marjorie Pervent, Ilana Lambert, Marc Tauzin, Alicia Karouani, Martha Nigg, Marie-Françoise Jardinaud, Dany Severac, Stefano Colella, Marie-Laure Martin-Magniette, Marc Lepetit, Systemic control of nodule formation by plant nitrogen demand requires autoregulation-dependent and independent mechanisms, Journal of Experimental Botany, Volume 72, Issue 22, 4 December 2021, Pages 7942–7956, https://doi.org/10.1093/jxb/erab374
- Share Icon Share
Abstract
In legumes interacting with rhizobia, the formation of symbiotic organs involved in the acquisition of atmospheric nitrogen gas (N2) is dependent on the plant nitrogen (N) demand. We used Medicago truncatula plants cultivated in split-root systems to discriminate between responses to local and systemic N signaling. We evidenced a strong control of nodule formation by systemic N signaling but obtained no clear evidence of a local control by mineral nitrogen. Systemic signaling of the plant N demand controls numerous transcripts involved in root transcriptome reprogramming associated with early rhizobia interaction and nodule formation. SUPER NUMERIC NODULES (SUNN) has an important role in this control, but we found that major systemic N signaling responses remained active in the sunn mutant. Genes involved in the activation of nitrogen fixation are regulated by systemic N signaling in the mutant, explaining why its hypernodulation phenotype is not associated with higher nitrogen fixation of the whole plant. We show that the control of transcriptome reprogramming of nodule formation by systemic N signaling requires other pathway(s) that parallel the SUNN/CLE (CLAVATA3/EMBRYO SURROUNDING REGION-LIKE PEPTIDES) pathway.
Introduction
Soil mineral nitrogen (N) availability is a major limiting factor of plant growth and crop productivity. Legume holobionts associated with rhizobia may escape from mineral N limitation because of their unique capacity to acquire the unlimited N source of atmospheric N2. The symbiotic interaction of a N-limited legume with compatible rhizobia results in the formation of organs called nodules generally formed in roots. In the symbiotic organs, the enzyme nitrogenase, expressed in differentiated bacteroids, is responsible for the reduction of N2 to NH4+, which is subsequently exported into the cytosol of the infected plant cells. However, symbiotic N fixation (SNF) is functionally highly dependent on the plant (Oldroyd et al., 2011). The allocation of sucrose from the shoot to the symbiotic organs is the source of carbon and energy, fueling the bacteroids. Ammonium assimilation by bacteroids is repressed, making them dependent on amino acids supplied by the plant (Prell et al., 2009). Expression of leghemoglobins by the plant allows the low-oxygen environment required for the nitrogenase activity in bacteroids (Ott et al., 2005; Larrainzar et al., 2020). In the last decade, mechanisms behind infection of roots by rhizobia and nodule formation have begun to be unraveled, notably in the model legumes Medicago truncatula and Lotus japonicus (Oldroyd et al., 2011; Mergaert et al., 2020). Recognition of compatible rhizobia by the plant allows infection and the activation of a complex pathway that finally results in the development of the symbiotic organs. The large transcriptome reprogramming associated with this process has been described in great detail (reviewed by Mergaert et al., 2020). It involves early and late up-regulation of hundreds of genes involved in early signaling responses, bacterial infection, hormones responses, organogenesis, rhizobial differentiation and activation of SNF in the roots.
Studies in many non-symbiotic plant species (including legumes not associated with their symbionts) showed that NO3- acts locally as a signal stimulating root development as well as NO3- acquisition and assimilation. These regulations contribute to root NO3- foraging, enabling the sessile plant to preferentially explore NO3-–rich soil patches (Drew, 1975; Forde and Lorenzo, 2001; Li et al., 2014; Gent and Forde, 2017). Genes involved in NO3- sensing and the large transcriptome and hormonal reprogramming associated with the response to NO3- have been discovered (Krouk et al., 2010; Vidal et al., 2020). However, mineral N also assimilates into downstream N metabolites required for whole-plant growth. The integration of N nutrition at the whole plant level allows adjusting the NO3- acquisition capacities to the N demand of the whole plant. The use of split-root systems experimentally revealed inter-organ systemic signaling controlling root N acquisition (Gansel et al., 2001). Molecular mechanisms behind these systemic regulations are starting to be unraveled (Okamoto et al., 2016; Ohkubo et al., 2017; Ota et al., 2020). Another whole plant control concerns the regulation by photosynthates of transporter genes expressed in the roots, allowing root N acquisition to match the shoot photosynthetic capacity (Lejay et al., 2003; 2008; Chaput et al., 2020). Although these local and systemic signaling mechanisms (local NO3-, plant N demand, photosynthates) can be discriminated conceptually and experimentally, it is a major challenge to decipher their interactions and crosstalk at the molecular level because they act synergistically, share many targets, and contribute to pleiotropic aspects of plant physiology. For example, three signaling pathways regulate the major root high-affinity NO3- transporter gene AtNRT2.1 involved in NO3- uptake in Arabidopsis (Chaput et al., 2020).
One of the major outcomes of the rhizobia-legume symbiosis is the delivery of N to the plant; therefore it is not surprising that the plant N status strongly determines symbiotic organ development and functioning. Successful nodule formation depends on the whole plant N deficit (Jeudy et al., 2010), and nodule senescence is activated when the holobiont has a sufficient mineral N supply (Pérez Guerra et al., 2010; Lambert et al., 2020b). There is a local suppression of symbiosis by the plant when nodules do not fix N2 that was qualified as a ‘sanction’ mechanism against ineffective symbiotic partners (Kiers et al., 2003). However, the N signaling mechanisms controlling symbiosis are not as well characterized as those involved in the control of mineral N acquisition. Although in L. japonicus, low amounts of nodule NO3- intake stimulate nodule functioning (Valkov et al., 2017), both the high amount of NO3- supply and the accumulation of downstream N metabolites repress symbiosis activity (Silsbury et al., 1986; Bacanamwo and Harper, 1997; Ruffel et al., 2008). A major impact of systemic N signaling on rhizobia-legume symbiosis was reported, particularly in Medicago truncatula (Jeudy et al., 2010; Laguerre et al., 2012; Lambert et al., 2020b). The provision of ample amounts of mineral N to symbiotic plants carrying mature nodules induces a systemic N signal that activates nodule senescence rapidly (Lambert et al., 2020b). Conversely, a N-deficit of a symbiotic plant caused by the suppression of SNF in half-root systems (Ar/O2 treatment) resulted in systemic stimulation of pre-existing nodule expansion and de novo nodule formation, to compensate for the N-deficit in the other roots not exposed to the Ar/O2 treatment (Jeudy et al., 2010; Laguerre et al., 2012; Lambert et al., 2020b). Systemic responses to variations of the symbiotic plant N demand correlates with variations of shoot-to-root sucrose translocation and/or hormonal pools in mature nodules (Lambert et al., 2020b).
There are contrasting reports about the balance between local and systemic regulation of nodule formation by mineral N, depending on the plant. Localized repression of nodule formation by NO3- was reported in soybean (Hinson, 1975; Tanaka et al., 1985; Cho and Harper, 1991; Xia et al., 2017), but the systemic repression of nodulation by mineral N supply is prominent in Medicago truncatula (Jeudy et al., 2010; Kassaw et al., 2015). Studies have shown that the addition of mineral N to the roots of legume-rhizobia holobionts is associated with important variation of the nodule transcriptome (Omrane et al., 2009; Moreau et al., 2011; Seabra et al., 2012; Cabeza et al., 2014). However, in the absence of split-root experiments, these studies did not discriminate between the local effects of mineral N (i.e. at the site of application) and the systemic effects (i.e. related to the satisfaction of the whole-plant N demand). A previous report based on split-root systems analysis showed that whole-plant systemic N signaling has a strong impact on the transcriptome of nodulated roots, but the effects of systemic N signaling on nodule formation and/or mature nodules were not discriminated (Ruffel et al., 2008). In a recent study, using split-root systems we characterized the impact of systemic N signaling on mature nodules in the Medicago truncatula/Sinorhizobium medicae holobiont (Lambert et al., 2020b). However, using the appropriate split-root system, characterization of systemic N signaling regulation of the legume root transcriptome reprogramming associated with early rhizobial interaction and nodule formation, has not yet been reported.
In legume-rhizobia holobionts, the autoregulation mechanism (AON) is involved in the systemic control of nodulation. It enables the earliest formed nodules to suppress further nodulation (Kosslak and Bohlool, 1984). Because AON is activated at the early stages of the interaction during nodule development, far before SNF becomes active, AON cannot be considered as a feedback mechanism related to the satisfaction of the N demand and/or accumulation of downstream N metabolites (Kosslak and Bohlool, 1984; van Brussel et al., 2002; Li et al., 2009). However, several lines of evidence argue for crosstalk between AON and N signaling. AON mutants form a high number of nodules (hypernodulating mutants) under a high mineral N supply, that is normally a suppressive condition for nodulation in the corresponding wild types (reviewed by Reid et al., 2011b). The suppression of SNF by Ar/O2 treatments on split-root plants revealed that whole-plant N-limitation releases the systemic inhibition of nodule formation by autoregulation (Jeudy et al., 2010; Laguerre et al., 2012). The AON mechanism involves a leucine-rich repeat receptor-like kinase (LRR-RLK) mainly present in leaves in several legume species (reviewed by Mortier et al., 2012b). In Medicago truncatula this receptor is encoded by SUPER NUMERIC NODULES (SUNN; Penmetsa et al, 2003; Schnabel et al., 2005). CLAVATA3/EMBRYO SURROUNDING REGION LIKE PEPTIDES (CLE) produced in the roots in response to the interaction with rhizobia are translocated from the root to the shoot, where they associate with the receptor, resulting in systemic inhibition of nodule formation (Mortier et al., 2012a; Okamoto et al., 2013). This inhibition is associated, both in Lotus and Medicago, with a lower translocation from the shoot to the root of the miRNA miR2111 (Tsikou et al., 2018; Nishida and Suzaki, 2018; Gautrat et al., 2020). Shoot cytokinin and methyl jasmonate accumulation might also have a role in the systemic control of nodulation in Lotus japonicus (Nakagawa and Kawaguchi, 2006; Kinkema and Gresshoff, 2008; Sasaki et al., 2014; Azarakhsh et al., 2018). In soybean, Lotus, and Medicago, some types of CLE, able to activate AON, accumulate in roots in response to NO3- through a pathway that may implicate NODULE INCEPTION (NIN) and other NIN-LIKE PROTEINS (NLP) transcription factors (Reid et al., 2011a; Nishida et al., 2018; Mens et al., 2021). Unexpectedly, these observations suggest an activation of systemic repression of nodulation resulting initially from a local sensing of NO3- rather than downstream accumulation of N metabolites.
Despite the reports indicating crosstalk between AON and systemic N signaling, there is also increasing evidence suggesting that AON-independent systemic N signaling mechanisms contribute to control nodule development (Jeudy et al., 2010; Kassaw et al., 2015). The nodule expansion response to systemic signaling of plant N deficit remains active in the sunn mutant (Jeudy et al., 2010). A systemic mechanism controlling nodule formation implicating COMPACT ROOT ARCHITECTURE 2 (CRA2), another LRR-RLK acting in shoots in Medicago truncatula, positively regulates nodule formation in parallel with the classical SUNN/AON pathway (Huault et al., 2014; Laffont et al., 2019; 2020). MtCRA2 is activated by small peptides of the CEP (C-TERMINALLY ENCODED PEPTIDES) family produced in roots exposed to mineral N deprivation or rhizobia (Mohd-Radzman et al., 2016). In Arabidopsis, CEP RECEPTOR 1 (CEPR1), the homolog of CRA2, was also found to interact with CEPs and mediate a systemic signaling pathway responsible for adjusting NO3- uptake capacity, to meet the N demand of the plant (Tabata et al., 2014; Ota et al., 2020). However, until now, the contribution of the CRA2/CEP pathway to the regulation of symbiosis by N signaling remains poorly understood.
In the present study, we characterized the control exerted by systemic N signaling on the root transcriptome reprogramming associated with early Sinorhizobium medicae interaction and nodule formation in Medicago truncatula. Systemic N signaling control of the plant demand was precisely characterized using split root systems, and its impact on nodule formation was determined. We identified systemic N signaling targets using RNAseq and statistical modeling on roots during early Sinorhizobium interaction and nodule organogenesis. The Medicago truncatula sunn mutant was compared with the wild type in order to characterize the AON-dependent and independent molecular targets of systemic N signaling controlling nodule formation.
Materials and methods
Split-root plant growth conditions
The Medicago truncatula genotypes were the wild type Jemalong A17 and the TR122 sunn mutant (sunn-2 allele; Sagan et al., 1995; Schnabel et al., 2005). The transgenic lines carried a pENOD11::GUS transgene in the A17 (line L416; Charron et al., 2004) or in TR122 backgrounds (obtained by genetic introgression of the L416 transgene into sunn). Experimental planning of the split-root experiments is presented in Supplementary Fig. S1. Seeds were scarified and germinated as described in Lambert et al., (2020b). Individual plantlets were transferred into hydroponic culture tanks containing a vigorously aerated HY basal nutrient solution (Lambert et al., 2020b) adjusted to pH 5.8 with KOH and supplemented with 1 mM KNO3. The primary root tips of plantlets were cut to promote branching of the root system. The culture chamber had a light intensity of 250 μmol m−2 s−1, a relative humidity of 70%, a light/dark cycle of 16 h/8 h and an ambient temperature of 22 °C/20 °C. We separated the root systems of 4-week-old plants into two parts. Initially all the split-root compartments contained HY nutrient solution supplemented with 0.5 mM KNO3 as a low mineral N source (pH adjusted to 7). We initiated the N treatments by providing a HY nutrient solution supplemented with high mineral N (10 mM NH4NO3) and low mineral N sources (0.5 mM KNO3) to the N satisfied and N-limited plants respectively (Fig. 1A). SNO and SN roots belong to N satisfied plants. LNO and LN belong to N-limited plants. The SNO and LNO compartments were supplied with NO3- containing HY nutrient solution whereas the SN and LN compartments were supplied with HY nutrient solution without mineral N (Fig. 1A). Roots of all compartments were inoculated 48 h after the N treatment initiation with Sinorhizobium medicae md4 (107 exponentially growing bacteria per ml; Laguerre et al., 2012). We renewed the nutrient solutions every 4 d. We initiated N treatments at different times before harvest in order to compare plants of the same age (6-week-old plants) differing by the duration post-inoculation (Supplementary Fig. S1). The scanned images (600 dpi) of the root systems were analyzed by using the Optimas image analysis software (MediaCybernetics, USA) to characterize root growth parameters. The GUS histochemical staining procedure has already been described (Lagarde et al., 1996).
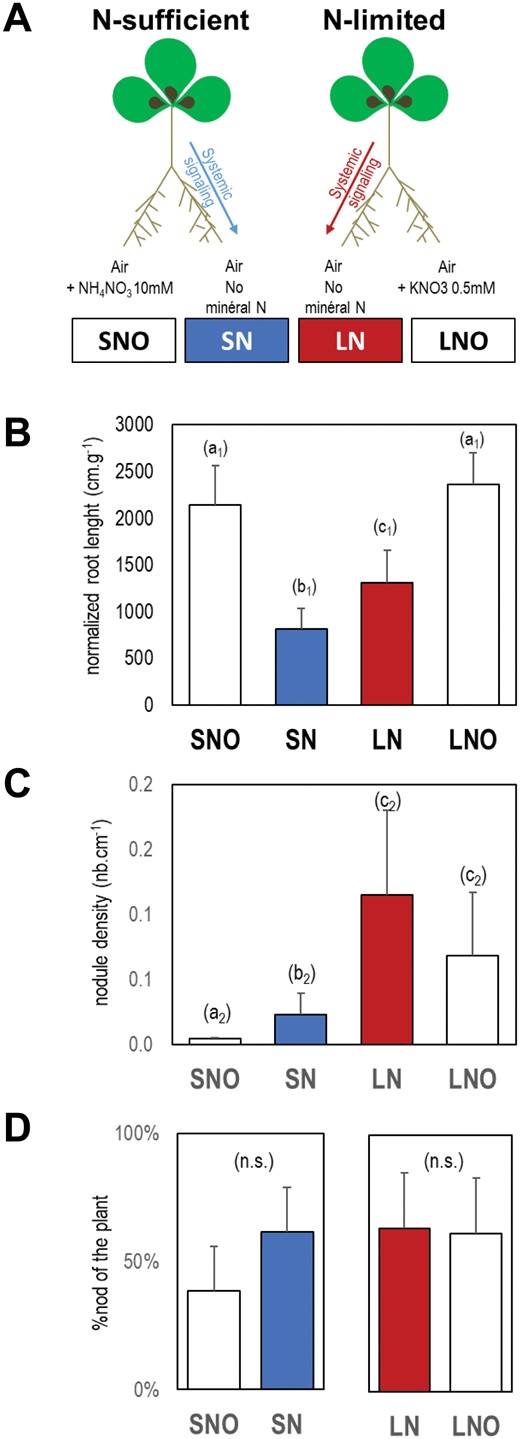
Split-root systems to study the response of nodule formation to N-sufficient and N-limitation signaling. (A) Plants were cultivated hydroponically for six weeks. Mineral N-treatments were applied on one half of the root system (SNO, LNO) 12 d before harvest. N sufficient or N limited treatments were achieved by providing 10 mM NH4NO3 or 0.5 mM KNO3, respectively. Effects of the N-treatments were studied on treated root sides (SNO, LNO) or on the other sides of the root system supplied with nutrient solution without mineral nitrogen (SN in blue, LN in red). All roots were inoculated with Sinorhizobium medicae md4 48 h after initiating the N treatments. (B) Normalized root length per shoot dry mass (NRL). (C) Nodule density (nodule number per root length). (D) Proportion of nodules (%) present in both compartments of the split-root systems. Detailed data are provided in Supplementary Table S2. Letters indicate distinct groups of values deduced from Kruskall-Wallis and pairwise Wilcoxon tests using a P-value threshold of 0.05, and Benjamini-Hochberg correction for multiple testing. n.s. indicates a non-significant difference. Values are means ±SD (n=5).
RNA analysis
The first experiment (exp1) compared the LN A17 roots at 0, 1, and 2 days post-inoculation (dpi; 0 is the non-inoculated control). The second experiment (exp2) compared the LN and SN roots at 2, 4, and 7 dpi. The third experiment (exp3) compared the LN and SN roots of A17 and sunn at 2 and 7 dpi. For each experiment, all roots samples were collected simultaneously in LN or SN compartments of the split-root systems (Supplementary Fig. S1). RNAseq analysis included three (exp1 and 3) or four (exp2) biological replicates per condition. Each biological replicate was a pool of the half root systems of two plants. Total RNA was extracted using QIAzol Lysis Reagent, purified using miRNeasy®, and digested by DNAse I to eliminate DNA contamination, according to the supplier’s recommendations (Qiagen, France). Polyadenylated plant mRNA libraries were generated and sequenced in single-read 50 nt mode using Illumina HiSeq 2500 technology (Illumina, USA), as described previously (Lambert et al., 2020b). The sequencing reads (ArrayExpress database accession numbers E-MTAB-9932, E-MTAB-9941, E-MTAB-9942) were mapped on the M. truncatula v4.2 genome using the Glint software (T. Faraut and E. Courcelle; http://lipm-bioinfo.toulouse.inrae.fr). The DiCoExpress tool used to analyze the RNAseq data is described in Lambert et al. (2020a). Briefly, after filtering, normalization of the raw data and quality control, the differential expression analysis used generalized linear models. For the first experiment, we expressed the log of the average gene expression as a function of dpi. For the second experiment, we expressed the log of the average gene expression as an additive function of the effects of N treatment, dpi, and interaction between N treatment and dpi. For exp3, we performed two analyses separately at 2 dpi and 7 dpi. In this case, we expressed the log of the average gene expression as an additive function of the effects of the N treatment, the genotype, and the interaction between N treatment and the genotype. Likelihood ratio tests allowed to evaluate the expression changes associated with ‘dpi’ in the first experiment, ‘N treatment’ in exp2, to ‘N treatment’ and interaction between ‘N treatment’ and ‘genotype’ in exp3. This last test allowed us to identify transcripts in exp3 differentially regulated by ‘N treatment’ in the sunn mutant compared with the wild type. The Benjamini–Hochberg procedure allowed the adjustment of the probabilities of significance to control the false discovery rate (FDR). We used a threshold of 0.05 to select differentially accumulated transcripts. Co-expression analysis was performed by using the ‘Coseq’ (v.1.4.0) algorithm (Rau and Maugis-Rabusseau, 2018) implemented and optimized in the DiCoexpress script (Lambert et al., 2020a). We complemented the MtV4 annotation with the Plant metabolic network (PMN)-Medicyc annotation of biochemical pathways (Urbanczyk-Wochniak and Sumner, 2007), to perform enrichment analysis using hypergeometric tests with the reference set defined as the whole genome. A functional enrichment of an annotation term was declared when the P-value associated with the hypergeometric test was lower than 0.05. Targeted RT–qPCR was performed on specific plant transcripts in a LightCycler (LightCycler 480; Roche,France) as described previously (Girin et al., 2007) using primers mentioned in Supplementary Table S1. The MtACTIN11 and MtGAPDH A housekeeping transcripts were used to normalize the data (Supplementary Table S1).
Results
Whole plant N signaling controls nodule formation
We characterized the effect of the plant’s N status on nodule formation using split root systems in Medicago truncatula (Fig. 1A). Plants were cultivated hydroponically in aerated nutrient solution. We separated roots of individual plants into two compartments. We supplied one half of the plant’s root system with two concentrations of mineral N in order to grow the plants at highly contrasted N regimes. Inhibition of nodule formation by addition of mineral N in the root medium has been known for a long time (Streeter and Wong, 1988). Our study focused on the systemic signaling during whole plant N demand. We designed split root systems to discriminate between local signal(s) related to the direct impact of the mineral N source and systemic signal(s) related to the N status of the whole plant (N-sufficient versus N-limited). We provided either a highly concentrated and rapidly assimilated N source (10 mM NH4NO3) or poorly concentrated N source (0.5 mM KNO3) to half root systems of the plants (40-fold variation of N concentration). These N regimes were already well characterized in previous studies on plants grown in equivalent conditions, and are known to result in highly contrasted N regimes and N intake (Girin et al., 2007; Ruffel et al., 2008; Jeudy et al., 2010). We confirmed that these two N regimes resulted in shoot dry weight higher in N-sufficient plants than in N-limited plants (Supplementary Table S2). We provided an aerated nutrient solution without mineral N to the second half root systems of these plants (Fig. 1A, SN and LN compartments).
We inoculated the roots of all the split-root compartments with Sinorhizobium medicae two days after establishing the N-treatments. To characterize the impact of systemic signals generated by the plants under these two regimes on inoculated roots, we compared roots exposed to the same local environment devoid of mineral N but connected to N-sufficient or N-limited plants (Fig. 1A, SN and LN compartments, respectively). To estimate the local effect of the N treatments, we compared half root systems of the same plant. As expected, adding NO3- (high or low concentrations) drastically stimulated root proliferation at the application site, doubling the normalized root length per shoot dry biomass (NRL; Fig. 1B; Supplementary Table S2 comparison of SNO versus SN and LNO versus LN). Comparing roots exposed to the same local environment but belonging to N-sufficient or N-limited plants (i.e. SN versus LN, both supplied with aerated nutrient solution without mineral N) indicated some repression of root development by systemic N-sufficient signaling, resulting in a 30% reduction of NRL in N-sufficient plants compared with N-limited plants (Fig. 1B; Supplementary Table S2). Systemic N signaling strongly repressed nodulation in N-sufficient plants compared with N-limited plants (Fig. 1C; Supplementary Table S2, comparison of SN versus LN). Some local effects of mineral N availability on root nodule density were also seen (Fig. 1C; Supplementary Table S2, comparison of SNO versus SN). However, because the relative proportion of nodules in the treated and untreated half root systems of N-sufficient or N-limited plants remained equivalent (Fig. 1D; Supplementary Table S2), these nodule density differences were more likely explained by the stimulation of root growth, rather than by a direct effect on nodulation. Interestingly, although root and nodule development was controlled, both by local and systemic signaling, their responses to N treatments were rather different. As expected, root development (root length) mainly resulted from local signaling of mineral N presence, whereas nodule formation mainly resulted from whole plant N systemic signaling. We further confirmed the morphological analysis by comparing LN and SN responses to inoculation in stable transgenic plants expressing pENOD11:: GUS reporter gene cultivated in split root systems (Fig. 1A). Both SN and LN roots displayed early infection responses at 1 and 2 dpi that were more attenuated in SN compared with LN at 4 dpi (Supplementary Fig. S2).
Impact of N signaling on transcript reprogramming associated with plant-rhizobia interaction
As a preliminary study, we confirmed that the transcriptome reprogramming associated with plant-rhizobia interaction in our split-root system was equivalent to previous reports on slightly different biological systems and culture conditions. We investigated the effect of the Sinorhizobium medicae inoculation (24 h, 48 h) on the LN roots of N-limited plants (Fig. 1A) using RNAseq. A total of 11 464 Sinorhizobium medicae-responsive transcripts (RRTs) were identified (Supplementary Table S3). As expected, they included typical transcripts encoding early nodulins, transcriptions factors, structural or regulatory proteins already characterized as markers of the induction of the Sinorhizobium infection, and nodule organogenesis programs (Supplementary Table S4). The activation of these genes correlates with the down-regulation of transcripts encoding transporters and enzymes involved in NO3- utilization (Supplementary Table S4).
Next, we addressed the question of the effect of the plant systemic N signaling on this transcriptome reprogramming associated with early plant-Sinorhizobium interaction and nodule formation. We used RNAseq and statistical modeling to compare the LN roots of N-limited plants and the SN roots of N-sufficient plants at 2, 4, and 7 dpi. Differential analyses at different inoculation times (Fig. 2) indicated that the number of transcripts differentially accumulated in LN versus SN roots increased strongly in the 4–7 d period (qualified in the text as ‘late’) compared with the 2–4 d period (qualified in the text as ‘early’). This observation suggested that the responses to systemic N signaling of roots inoculated by Sinorhizobium varied during the interaction. A total of 8133 N-responsive Differentially Accumulated Transcripts (DATs) in at least one of these pairwise comparisons (2,4, or 7 dpi) were identified (Supplementary Table S5). Only 25% of the RRTs were N-responsive DATs, which was a highly significant (hypergeometric test, P-value<0.05), but a marginal fraction. This indicated that the plant response to Sinorhizobium is not fully dependent on the plant N status. However, the RRTs were particularly abundant in the N-responsive DATs (57%) indicating that most of the root transcripts regulated by systemic N signaling were involved in the response to Sinorhizobium (Fig. 3). Globally, the N-responsive DATs were significantly enriched in transcripts of genes belonging to symbiosis-related islands of the Medicago truncatula genome (36% of the N-responsive DATs; hypergeometric test, P-value<0.05) described by Pecrix et al. (2018), as well as in transcripts specifically accumulated in the nodule (35% of the N-responsive DATs; hypergeometric test, P-value<0.05) described by Roux et al. (2014). Many transcripts associated with the late phases of nodule organogenesis and SNF maturation belonged to N-responsive DATs (Fig. 3). A strong impact of systemic signaling was particularly noticed on transcripts associated with bacteroid differentiation. The accumulation of numerous transcripts encoding NCR (nodule cysteine-rich peptides) and GRP (nodule glycine-rich peptides; (Supplementary Fig. S2), associated with bacteroid differentiation (Kereszt et al., 2018), as well as MtDNF1, MtDNF2 (DEFECTIVE IN NITROGEN FIXATION 1 and 2, respectively), and MtCCS52a (CELL CYCLE SWITCH 52a)), strongly depended on systemic N signaling (i.e. up-regulated in N-limited plants compared with N-sufficient plants). Transcripts involved in nodule functioning such as LEGHEMOGLOBIN and the sugar efflux transporter MtSWEET11, potentially involved in nodule sugar allocation, displayed similar behaviors.
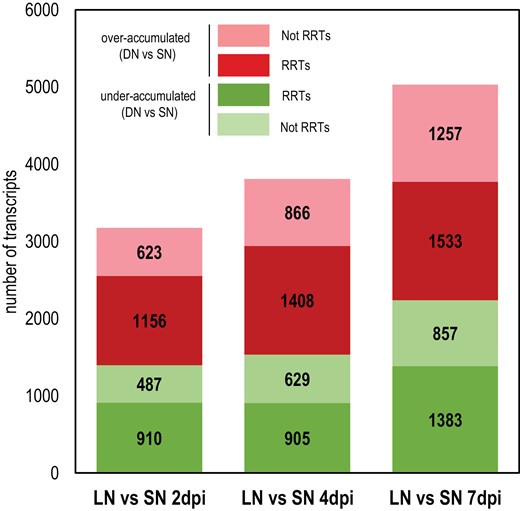
Effect of systemic N signaling on the transcriptome of inoculated roots. LN and SN roots were compared at 2, 4, and 7 dpi. Red and green areas represent transcripts over-accumulated and under-accumulated, respectively, in DN roots, compared with SN roots. Dark colors represent Sinorhizobium medicae-responsive transcripts (RRTs).
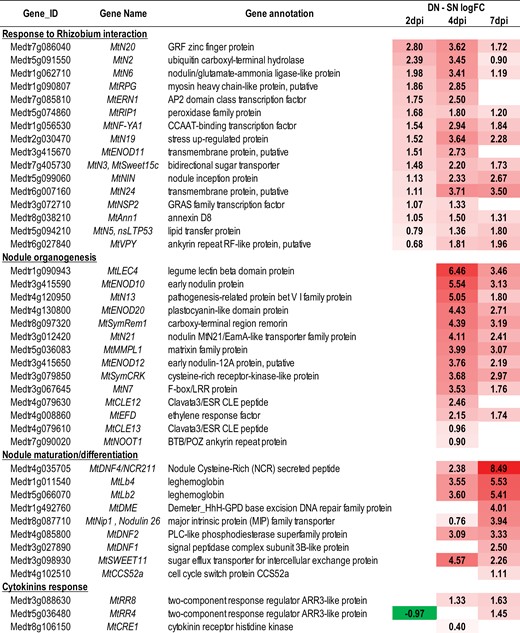
Heat map of the effects of systemic N signaling on the expression of marker genes associated with nodule formation. LN and SN roots were compared at 2, 4, and 7 dpi (Supplementary Table S4). Only significant FC difference (P-values and FDR <0.05) are indicated. Red and green colors represent transcripts up-regulated and down-regulated, respectively, in LN roots, compared with SN roots.
The co-expression analysis based on mixture models organized the N-responsive DATs in 10 clusters according to their accumulation kinetics in LN- and SN-inoculated roots (Fig. 4A; Supplementary Table S6). The model fitted the data well as only 10% of transcripts were not classified. The 10 clusters can be grouped according to the transcriptional profile in response to N-limitation signaling: up-regulation in clusters 1, 2, 4, 5, 8, 10, and down-regulation in clusters 3, 6, 7, 9 (Fig. 4A). As expected, the RRTs identified in our initial response to inoculation analysis (1 and 2 dpi; Fig. 4A; Supplementary Table S5) were highly represented in all these clusters except for cluster 4 that only gathered transcripts activated in the late phases of nodule formation: bacteroid differentiation (NCRs, GRPs), activation of SNF (LEGHEMOGLOBINs). Compared with the whole annotated genome, each cluster is associated with specific functions, as shown in Fig. 4B (hypergeometric test P<0,05; Supplementary Table S6)). Cluster 3 was particularly enriched in transcripts related to NO3- utilization, while clusters 4 and 5 gathered many transcripts encoding ‘nodulins’. Clusters 2 and 4 contained most of the NCRs, GRPs, and LEGHEMOGLOBIN transcripts, as well as the sugar transporter MtSWEET11 transcript.
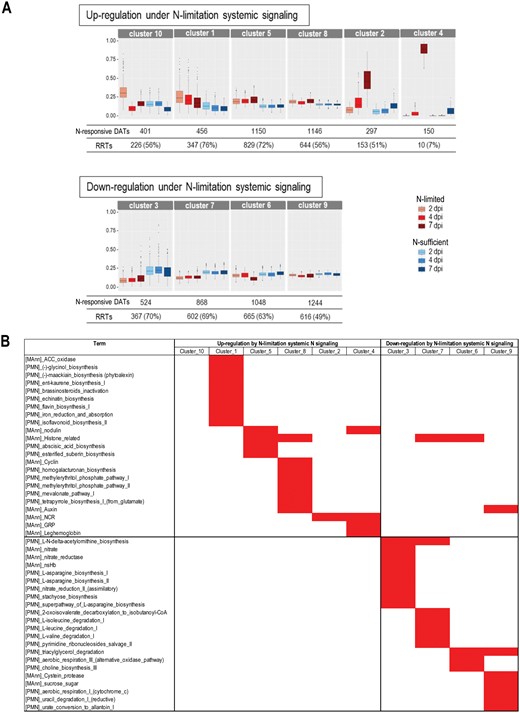
Co-expression analysis of the transcripts differentially accumulated in response to systemic N signaling in Sinorhizobium medicae-inoculated roots. LN and SN roots were compared at 2, 4, and 7 dpi. N-responsive DATs, specified co-expression clusters, RRT annotation, gene names, and annotations are listed in Supplementary Table S6. (A) Co-expression clusters and their normalized accumulation kinetics (coseq package). (B) Red blocks indicate specific annotation enrichment of the cluster compared with the entire annotated genome (estimated by hypergeometric test with a threshold of 0.05 for P-value).
Role of AON in the repression of nodule formation by systemic N -sufficient signaling
We compared the sunn mutant impaired in the LRR-RLK receptor required for AON signaling in Medicago truncatula, with the WT A17 plants, in split-root systems (Fig. 1). We monitored the expression of six marker genes known to be up-regulated at various stages of the nodulation process (Fig. 5). Medicago truncatula transcripts encoding the early nodulin MtENOD11 (Journet et al., 2001), the transcription factors MtNIN (Marsh et al., 2007), NUCLEAR FACTOR Y-A1 (MtNFY-A1; Combier et al., 2007), NODULATION SIGNALING PATHWAY 2 (MtNSP2 ; Kaló et al., 2005), and ETHYLÈNE RESPONSIVE FACTOR REQUIRED FOR NODULATION 1 (MtERN1; Middleton et al., 2007) orchestrating transcriptome reprogramming associated with rhizobia-legume interaction, marked the early infection stage. RESPONSE REGULATOR 4 (MtRR4; Gonzalez-Rizzo et al., 2006), involved in the cytokinin response, or MATRIX METALLOENDOPROTEINASE-LIKE 1 (MtMMPL1 ; Combier et al., 2007), involved in the progression of infection, marked nodule organogenesis. Late stages of nodule formation were marked by NCR transcripts, associated with bacteroid differentiation, as well as transcripts encoding LEGHEMOGLOBINs, allowing the bacterial nitrogenase to be active in a microoxic environment (Ott et al., 2005). Transcript levels were quantified by RT–qPCR before inoculation and 1, 2, 3, and 7 dpi, in LN and SN root systems. In parallel, we monitored the expression of pENOD11::GUS reporter gene in roots of stable transgenic plants of the same genotypes (Supplementary Fig. S2). As expected, the six transcripts were all up-regulated in response to Sinorhizobium in LN roots. We confirmed they were all regulated by systemic N signaling in A17. MtENOD11, MtNIN, and MtNFYA1 were up-regulated rapidly at the early stages of the interaction in both SN and LN roots. Their responses to Sinorhizobium at 3–7 dpi were attenuated in SN roots compared with LN roots. MtRR4, MtMMPL1, MtNCR084, and LEGHEMOGLOBIN were induced in LN roots only after 2 dpi (Fig. 5). Their inductions were strongly reduced in SN roots compared with LN roots. The impact of the SUNN mutation on the regulation of these genes by systemic N signaling was investigated. Both RT–qPCR and pENOD11::GUS reporter gene analysis support that SUNN mutation prevented the repression by N sufficient systemic signaling, of MtNIN, MtNFYA1, MtENOD11, MtRR4, and MtNCR084 expression (Fig. 5; Supplementary Fig. S2). However, this was not true for LEGHEMOGLOBIN that remained repressed by N-sufficient systemic signaling in both sunn and A17. Although the SUNN mutation partially released the repression of nodulation by N-sufficient systemic signaling, a SUNN-independent systemic repression by N-sufficient conditions remained active for the LEGHEMOGLOBIN transcript.
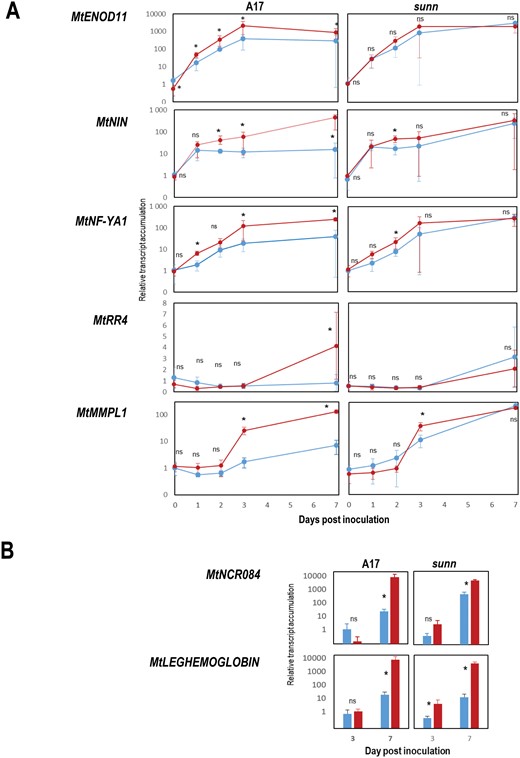
Accumulation of selected transcripts in Sinorhizobium medicae-inoculated roots under contrasted systemic N-signaling. sunn and WT plants were cultivated in the split root systems described in Fig. 1A. Inoculated LN (in red) and SN (in blue) roots belonging to N-limited and N-sufficient plants, respectively, were compared at 0, 1, 2, 3, and 7 dpi. Total RNA was extracted from the roots and transcript of interest accumulation was quantified by RT–qPCR. The MtACTIN11 and MtGAPDH A ‘housekeeping’ transcripts were used to normalize the data. For each transcript of interest, normalized levels are presented relative to the average level measured at the first time point (arbitrarily fixed to 1 for each time point). (A) Kinetics of MtENOD11, NFYA1, NIN, MtRR4, MtMMPL1 transcripts at 0, 1, 2, 3, and 7 dpi in WT and sunn. (B) Transcript accumulation of MtNCR084 and MtLEGHEMOGLOBIN at 3 and 7 dpi in WT and sunn (transcripts not detected at 0, 1, and 2 dpi). Values are means ± SD, n=6. At each time point LN and SN values were compared according to the Kruskall-Wallis test (threshold P=0.05). * significant difference; ns, non-significant difference.
We extended these observations to the whole genome by comparing the responses of the Sinorhizobium-inoculated root transcriptome to systemic N signaling (SN versus LN) in A17 and sunn at 2 and 7 dpi. Transcripts displaying differential or equivalent responses to systemic N signaling in both genotypes were discriminated by statistical modeling and likelihood ratio tests (Supplementary Table S7). The proportion of transcripts displaying a differential response to systemic signaling comparing sunn and A17 were higher at 7 dpi (43%, 3666/8442) than at 2 dpi (11%, 388/3458). Furthermore, 60% of transcripts displaying a differential response to systemic N signaling between A17 and sunn at 7 dpi belong to N-responsive-DATs. The overlap was large, with N-responsive-DATs up-regulated in response to N-limitation (compared to N satisfaction), particularly for the co-expression clusters 2 and 4 (>90% of the transcripts differentially regulated in sunn and A17). The regulation by N-systemic signaling of typical transcripts known to be associated with rhizobia interaction and nodule formation was clearly impaired in the mutant, consistent with its phenotype that results in the maintenance of nodule formation under N-sufficient signaling (Supplementary Table S8). Nonetheless, our data confirm that not all the responses of the nodulated roots to systemic N-sufficient signaling were impaired in the sunn mutant. Most of the transcripts encoding LEGHEMOGLOBIN present in the transcriptome at 7 dpi (8/11) displayed an equivalent response to N signaling in sunn and A17, confirming our RT–qPCR studies (Supplementary Table S9). Among the 498 NCR transcripts identified in the roots of A17 at 7 dpi, 313 displayed impaired regulation by systemic N signaling in the mutant compared with A17, but 185 other transcripts displayed equivalent regulation in the two genotypes (Supplementary Table S10). Similarly, transcripts encoding GRP or annotated as ‘nodulins’ may be easily discriminated into two categories according to the impact of the sunn mutation on their regulation by systemic N signaling (Supplementary Tables S11; S12). This duality of expression profiles did not concern all families of transcripts present at 7 dpi: for example, the systemic N signaling regulation of the 20 transcripts encoding defensin peptides was always dependent on SUNN (Supplementary Table S13). Despite a clear role of SUNN in the control of nodule formation by systemic N signaling, altogether, these data demonstrated that additional SUNN-independent mechanisms control the late phase of nodule formation/maturation, and importantly, may contribute towards the adjustment of the symbiotic capacity to the plant N demand.
Discussion
Systemic signaling of the whole plant N demand is a major driver of nodule formation
This study provided new insights about the control of legume-rhizobia symbiosis by the plant N demand. The success of the developmental process initiated by the plant-bacterial interaction is under the control of systemic signaling of the whole plant N demand. This control has a great biological significance because symbiotic organ formation and SNF have elevated carbon and energy costs (fulfilled through the allocation of photosynthates to the symbiotic organs). This whole plant mechanism allows adjusting the root nodule capacity to the N demand of the entire holobiont, and prevent unnecessary dissipation of resources and energy.
Several mechanisms have been described in non-symbiotic plants that contribute to adjust N acquisition capacity to N availability and N demand. Numerous studies have characterized the local stimulation of root development by NO3- resulting in root foraging (Drew, 1975; Forde and Lorenzo, 2001; Li et al., 2014; Gent and Forde, 2017). NO3- acts as a signal allowing sessile plants to explore and preferentially deplete NO3--rich soil patches. This mechanism interacts with a whole plant control that stimulates or represses mineral N uptake and root development as a function of the plant N demand. In symbiotic plants, both NO3- foraging and symbiotic nodule formation may be revealed and may mediate plant adaptation to N-limitation (Jeudy et al., 2010). However, the underlying N signaling mechanisms associated with these two processes are different. This study shows that NO3- stimulates the root proliferation locally in inoculated plants equivalent to that in non-symbiotic plants (Fig. 1B). However, we failed to obtain a clear argument supporting a specific local signaling effect of mineral N on nodule formation. Although we found that NO3- reduces nodule density locally (Fig. 1C), this may be explained by the stimulation of root expansion (Fig. 1B, C), rather than by a direct reduction of nodule formation. We present evidence showing that both root development and nodule formation are under the control of systemic signaling of the plant N demand in symbiotic plants (Fig. 1B, C). Specificities of the underlying developmental processes (nodule versus root development) do not rule out the hypothesis of a common upstream control mechanism responsible for sensing and integrating the whole plant N demand. Indeed, mineral N acquisition and SNF fuel the entire plant’s N metabolism, and result in the same downstream products. However, although several studies provided evidence for whole plant N demand regulation, and despite several molecular components associated with systemic N signaling being identified (Li et al., 2014; Okamoto et al., 2016; Bellegarde et al., 2017; Jia and von Wirén, 2020), globally, the underlying whole-plant N sensing mechanism remains elusive.
Systemic signaling of the whole plant N demand controls the progression of root transcriptome reprogramming initiated by rhizobia infection
This study reports the identification at the genomic level of the root transcripts that are under the control of systemic signaling of the plant N demand during nodule formation (2, 4, and 7 dpi). This large set of data will benefit from further studies on the complex mechanisms involved in this systemic control. Globally, rapid responses to rhizobia were observed whatever the N status of the plant, suggesting that the capacity of the root to respond to rhizobia is not dependent on systemic N signaling. However, systemic N signaling related to plant N sufficient conditions and plant N limitation modulates a significant part of the transcriptome reprogramming associated with Sinorhizobium-legume interaction (but not all). Both the number of transcripts differentially accumulated in response to systemic N signaling (Fig. 2) and the amplitude of their responses increased during the progression of the plant-Sinorhizobium interaction (Figs 3; 4). The accumulation of many transcripts rapidly up-regulated by Sinorhizobium responded to systemic N signaling, but the impact of systemic N signaling was generally modest at 2 dpi while more pronounced at 4 and 7 dpi (Fig. 3). Many typical transcripts that responded rapidly to nod factor signaling, such as, for example, ENOD11, NFY-A1, or NIN, were up-regulated in response to Sinorhizobium, whatever the N status of the plant (Fig. 5). However, they were progressively repressed in roots under N-sufficient signaling after several days, whereas they remained activated in roots under N-limitation signaling. Transcripts strongly activated by Sinorhizobium at the late stage of the interaction in N-limited conditions generally responded very poorly to Sinorhizobium when analyzed in roots under N-sufficient signaling. These transcripts were either associated with nodule organogenesis and rhizobia colonization (e.g. MtRR4, MtMMPL1, MtDME), bacteroid differentiation (e.g. NCRs, GRPs), or associated with the activation of SNF (e.g. MtSWEET11, LEGHEMOGLOBIN). We conclude that systemic N signaling does not likely determine the competency of the root to respond to rhizobia, but instead controls the symbiotic process leading to progress or abortion of bacterial infection, nodule organogenesis and SNF activation.
Control by systemic N signaling of transcriptome reprogramming associated with nodule formation requires AON-dependent and AON-independent components
AON is frequently interpreted as a negative feedback mechanism allowing the plant to limit symbiotic development to prevent the useless dissipation of energy and photosynthates (Reid et al., 2011b). Mutants impaired in the SUNN receptor form nodules even when supplied with a high amount of mineral N, suggesting an important role of AON in the systemic control of nodulation by the plant’s N status (Kinkema et al., 2006). The SUNN mutation clearly impaired systemic repression by N-sufficient signaling of numerous transcripts associated with the response to rhizobia, the infection, and nodule organogenesis, in agreement with this hypothesis (Fig. 5; Supplementary Table S8). However, we showed that the AON pathway is required for only a part of systemic N signaling control of the early rhizobia-legume interaction. Firstly, the impact of the sunn mutant on systemic N signaling control of the root transcriptome reprogramming at 2 dpi is significant, but marginal (Supplementary Table S7). Secondly, although the active SUNN gene is required for systemic N signaling-control of many transcripts of root transcriptome reprogramming at 7 dpi, many other N-responsive transcripts contributing to this reprogramming display equivalent systemic N signaling control in the mutant and in the wild type at 7 dpi (Supplementary Table S7). Notably, many nodule-specific transcripts involved in bacteroid differentiation (NCRs and GRPs families; Supplementary Tables S10 and S11), activation of SNF, and sucrose allocation (LEGHEMOGLOBIN, SWEET11) belong to this last group (Supplementary Table S9). As the present study is only restricted to the regulation of transcript amounts, further work is needed to confirm the functional impact of these regulations (protein synthesis and stability, activity, fluxes, etc.). Nevertheless, these data provided molecular evidence to previous physiological studies on the sunn mutant (Jeudy et al., 2010, Kassaw et al., 2015), suggesting that the N signaling response of symbiosis had SUNN-dependent and SUNN-independent components. Furthermore they strongly suggest why the hypernodulation phenotype paradoxically does not result in a higher level of SNF per plant, despite a higher nodule biomass per plant (Jeudy et al., 2010). Our study supports the hypothesis of a SUNN-independent systemic N signaling mechanism downstream of nodule organogenesis controlling SNF activity in every nodule. This mechanism would have a major role in the adjustment of symbiotic N acquisition capacity to the whole plant N demand. The targets of such a mechanism are likely to be the transcripts encoding proteins involved in bacteroid differentiation, SNF, and sucrose allocation of nodules from the plant. This hypothetic mechanism has features apparently reminiscent of the systemic N signaling mechanism operating in the mature nodule (Lambert et al., 2020b). Whether they are distinct mechanisms or the same ones observed at different stages of nodule development, remains to be clarified.
A control by the N demand of plant allocation of C metabolites to the roots fueling the SNF, and providing a carbon skeleton to amino acid synthesis, is consistent with the strong integration of C and N metabolism at the whole plant level (Stitt et al., 2002; Ruffel et al., 2014; Chaput et al., 2020). This study also revealed little impact of the SUNN mutation on the systemic N signaling response observed at 2 dpi, suggesting that another SUNN-independent mechanism might be also required to explain the N-signaling responses of the early symbiotic interaction. Interestingly another systemic mechanism controlling early stages of nodule formation and operating in parallel to the SUNN-dependent AON has been discovered (Huault et al., 2014; Laffont et al., 2019; 2020). The possible role of this mechanism in the systemic N-signaling of early legume-rhizobia interaction is not known and deserves to be investigated.
Symbiotic-specific or global systemic N signaling mechanism?
Altogether, our data shed light on the high complexity of the control of symbiosis by the plant’s N status and their high level of integration. Because these controls target specific symbiotic processes, they may be interpretated as the result of symbiosis-specific mechanisms. However, whether they form specific pathways or are symbiotic components orchestrated by a global signaling hub common to root development and mineral N acquisition, remain to be clarified. There is increasing evidence indicating that SUNN/CLE and CRA2/CEP pathways, initially identified for their role in the systemic control of nodule formation, also have non-symbiotic functions in root development or NO3- uptake (Okamoto et al., 2013; Tabata et al., 2014; Goh et al., 2019; Lagunas et al., 2019). Furthermore, closely related pathways controlling root development and NO3- uptake have been identified in non-legume plants (Tabata et al., 2014; Ota et al., 2020). This argues for the hypothesis that they have been recruited by symbiosis for specific function(s), but also suggests they may be components of a global network of systemic N signaling mechanisms, controlling and coordinating underground plant development as a function of plant nutritional demand.
Supplementary data
The following supplementary data are available at JXB online.
Table S1. List of primers used for RT–qPCR analysis.
Table S2. Developmental parameters of N-satisfied and N-limited plants cultivated in the split-root systems used to study the response of nodule formation to N signaling.
Table S3. Differentially accumulated transcripts in response to the inoculation of LN roots by Sinorhizobium medicae md4 (RRTs).
Table S4. Differential expression in LN roots inoculated with Sinorhizobium medicae md4 of selected marker transcripts associated with early interaction with rhizobia in previous studies.
Table S5. Differentially accumulated transcripts in response to systemic N signaling in Sinorhizobium medicae md4 inoculated roots (N-responsive DATs).
Table S6. Assignment of N-responsive DATs to co-transcribed clusters.
Table S7. Comparison of the transcriptome responses to systemic N signaling of sunn and A17 Sinorhizobium-inoculated roots at 2 and/or 7 dpi.
Table S8. Effect of the SUNN mutation on the systemic N signaling response of marker transcripts associated with symbiosis.
Table S9. Effect of the SUNN mutation on the systemic N signaling response of plant hemoglobin transcripts in roots at 7 dpi.
Table S10. Effect of the SUNN mutation on the systemic N signaling response of NCR transcripts in roots at 7 dpi.
Table S11. Effect of the SUNN mutation on the systemic N signaling response of GRP transcripts in roots at 7 dpi.
Table S12. Effect of the SUNN mutation on the systemic N signaling response of transcripts annotated as nodulins in roots at 7 dpi.
Table S13. Effect of the SUNN mutation on the systemic N signaling response of transcripts annotated as defensins in roots at 7 dpi.
Fig. S1. Experimental planning of the split-root experiments.
Fig. S2. Histochemical localization of GUS activity in the SN and LN roots of sunn and A17 transgenic pENOD11:GUS plants.
Fig. S3. Transcripts encoding NCR and GRPs differentially accumulated in response to systemic N signaling in inoculated roots.
Acknowledgements
We thank Etienne-Pascal Journet for kindly providing seeds of A7/NL415 and sunn-2/NL415, Renaud Brouquisse and Pierre Frendo for critical reading of the manuscript.
Author contributions
ML designed the experiments; MP, AK, MN, and ML performed the split-root experiments and the root developmental analysis; MP, IL, SC, MT, FJ, M-LM-M, and ML performed the RNAseq analysis; MP, AK, and MN performed RT–qPCR analysis; ML wrote the manuscript, with contributions of SC and MP, and revisions from all authors.
Funding
This work was supported by the Agence Nationale de la Recherche (ANR) grants Psyché (ANR-16-CE20-0009) and LabEx Saclay Plant Sciences-SPS (ANR-10-LABX-0040-SPS).
Data availability
The data supporting the findings of this study are available within the paper, within its Supplementary materials published online or in ArrayExpress database (Accession numbers E-MTAB-9932, E-MTAB-9941, E-MTAB-9942).
Comments