-
PDF
- Split View
-
Views
-
Cite
Cite
Wenke Ludwig, Scott Hayes, Jana Trenner, Carolin Delker, Marcel Quint, On the evolution of plant thermomorphogenesis, Journal of Experimental Botany, Volume 72, Issue 21, 20 November 2021, Pages 7345–7358, https://doi.org/10.1093/jxb/erab310
- Share Icon Share
Abstract
Plants have a remarkable capacity to acclimate to their environment. Acclimation is enabled to a large degree by phenotypic plasticity, the extent of which confers a selective advantage, especially in natural habitats. Certain key events in evolution triggered adaptive bursts necessary to cope with drastic environmental changes. One such event was the colonization of land 400–500 million years ago. Compared with most aquatic habitats, fluctuations in abiotic parameters became more pronounced, generating significant selection pressure. To endure these harsh conditions, plants needed to adapt their physiology and morphology and to increase the range of phenotypic plasticity. In addition to drought stress and high light, high temperatures and fluctuations thereof were among the biggest challenges faced by terrestrial plants. Thermomorphogenesis research has emerged as a new sub-discipline of the plant sciences and aims to understand how plants acclimate to elevated ambient temperatures through changes in architecture. While we have begun to understand how angiosperms sense and respond to elevated ambient temperature, very little is known about thermomorphogenesis in plant lineages with less complex body plans. It is unclear when thermomorphogenesis initially evolved and how this depended on morphological complexity. In this review, we take an evolutionary–physiological perspective and generate hypotheses about the emergence of thermomorphogenesis.
Introduction
In the context of global warming, temperature and its impact on the biosphere has gained increasing awareness throughout the past 30 years. Due to their immobile lifestyle, plants are particularly affected by changes in ambient temperature. Species of many plant lineages acclimate to warm temperatures with a wide range of cellular, physiological, and morphological responses. These acclimations can enhance plant fitness, but superoptimal temperatures (above optimum, but below lethal stress) still exert a negative effect on plant growth and development, and likewise on crop yields (Lobell et al., 2011; Lobell and Tebaldi, 2014; Ostberg et al., 2018; Tigchelaar et al., 2018; reviewed in Lippmann et al., 2019 and Zhu et al., 2021). Understanding how plants perceive elevated ambient temperature and transduce it into a physiological response is therefore an important goal. The study of ambient temperature-induced changes in plant architecture (thermomorphogenesis) is an emerging research field that has seen significant progress, particularly over the last decade (reviewed in Quint et al., 2016; Casal and Balasubramanian, 2019; Lamers et al., 2020; Hayes et al., 2021). A hitherto neglected aspect is how thermomorphogenesis evolved. Studying the evolution of warm ambient temperature adaptations could unlock potential avenues towards the development of temperature-resistant crops.
It is widely accepted that early land plants (embryophytes) emerged from streptophyte algae, freshwater green algae, in the middle Cambrian–early Ordovician around 400–500 million years ago (Fig. 1; Rubinstein et al., 2010; Morris et al., 2018). While many details of terrestrialization remain unclear, recent efforts in palaeobotany, phylogeny, and evo-devo have generated an impressive amount of data, bringing us closer to understanding the processes that were essential for the colonization of land (reviewed in Rensing, 2018). During the transition from water to land, alterations of temperature, light, and water conditions were presumably amongst the greatest challenges that plants faced. Moving from water to an air-based habitat required a number of adaptational innovations on the level of metabolism, morphology, and gene regulation. Many of these aspects were recently highlighted by Fürst-Jansen et al. (2020). Light is absorbed by water, which alters its intensity and quality. Likewise, the physical capacities of air and water are very different with regard to temperature. The specific thermal capacity of air at room temperature (1.005 kJ kg−1 K−1) is much smaller than that of water (4.2 kJ kg−1 K−1). As a result, temperature fluctuations are reasonably well buffered in water, but much more pronounced on land. After the initial terrestrialization event, average global temperatures drastically changed during the evolution of the geosphere (Snyder, 2016), requiring land plants to steadily adapt to a changing thermal environment. In addition, temperature fluctuates in a diurnal or seasonal manner, constantly affecting plant growth on the metabolic, cellular, and morphological level. The ability of plants to properly integrate and respond to temperature fluctuations is important for plant fitness (Ibañez et al., 2017). One set of reactions that help plants to better cope with such variation is the ability to alter the plant phenotype in response to the environment, a type of ‘behaviour’ defined as phenotypic plasticity (Nicotra et al., 2010).

Body plan innovations during plant terrestrialization. In the course of evolution plants acquired several innovations. One of the earliest was the development of multicellularity (already present in green algae). This was followed by three-dimensional growth in specific algal lineages and in land plants (embryophyta), which evolved in the middle Cambrian–early Ordovician (450–500 million years ago). Whereas bryophytes lack a water conduction system and possess rhizoids, tracheophytes developed a vasculature system and roots. These novelties enabled them to increase body size and to become more complex with the advent of apical branching (450 million years ago). This increased morphological complexity enabled plastic architectural responses to a broad range of abiotic factors including temperature.
In this review, we will take a closer look at the evolution of temperature acclimation from a physiological perspective and with a special focus on phenotypic plasticity in response to elevated temperature, a process known as thermomorphogenesis (Erwin, 1989, Delker et al., 2014). Thermomorphogenic responses occur within the ambient temperature range and are distinct from heat stress responses, which are induced at temperatures well above the superoptimal range. As thermomorphogenesis is still a rather young sub-discipline of the plant sciences, experimental data are scarce for most plant lineages. For this reason, several of our thoughts and conclusions remain hypothetical.
Temperature effects on land plant metabolism
Phenotypic plasticity is mostly understood as altered plant growth and/or morphology, but it also occurs at the molecular level. Alterations in metabolism often respond much more quickly than adjustments in growth. Almost all metabolic processes are temperature-dependent. Ambient warm temperatures lower the activation energy of enzymatic reactions and enhance reaction rates, thereby affecting metabolic flux. The temperature coefficient Q10 is used as a measure of temperature sensitivity. While classically used to describe the exponential temperature effect on chemical reactions, Q10 is also useful as a measure of temperature sensitivity in physiological responses. It describes the factor by which a certain reaction (or trait) responds by every 10 °C change in temperature. Since Q10 was originally developed for simple chemical reactions, adjusted calculations may be needed to account for distorted reaction shapes in biological systems (Mundim et al., 2020). In plants, one of the best characterized temperature-dependent metabolic pathways is photosynthesis in C3 plants. Photosynthetic rates increase with rising temperature until a species-specific optimal temperature is reached (e.g. Marsh et al., 1986; Battaglia et al., 1996; Rasmusson et al., 2019). While carbon fixation rates are positively affected by increasing temperatures up to that optimal point, the overall net photosynthetic rates start to decline before reaching the thermal optimum. This decrease is largely explained by the simultaneous increase in respiration rates (Hofstra and Hesketh, 1969; Clark and Menary, 1980; Heskel et al., 2016), which exponentially increase when exposed plant tissues heat up (Crawford et al., 2012; Park et al., 2019). At some point, this results in a net carbon loss.
Above the species-specific thermal optimum, net photosynthesis rates decline. Heat-induced reductions are mainly due to thermal damage of the photosynthetic apparatus (Yamori et al., 2014). For example, thylakoid membranes become leaky, allowing the crossing of protons and disrupting ATP synthesis (Chapman et al., 1983; Tikhonov and Vershubskii, 2020). Even moderate temperature elevations affect the capacity for carbon fixation due to their effect on Rubisco, one of the major determinants of photosynthetic efficiency. Rubisco is affected on several levels by elevated temperatures. This includes, but is not limited to, reduced activation and limited CO2 fixation (Kim and Portis, 2005; Yamori et al., 2014), increased oxygenation reactions in its active centre followed by the necessity for costly recycling via photorespiration (Ku and Edwards, 1977a, b; Jordan and Ogren, 1984), and restricted regeneration of Rubisco’s substrate ribulose-1,5-bisphosphate under certain climatic conditions (Sage and Kubien, 2007). Plants can respond to this temperature stress and limit its negative effects by inducing cellular changes such as stabilizing thylakoid membranes and accumulating lipid droplets. The latter are suggested to serve as a sink for free fatty acids and can be reintegrated as membrane lipids after temperature stress has ended (Mueller et al., 2015; Yang and Benning, 2018). Alternative ways of heat stress mitigation include the activation of HEAT SHOCK PROTEINs (HSPs) to stabilize structural components of the photosynthetic machinery, and the reduction of respiration rates. Notably, the genetic framework of HSPs and lipid droplet formation in response to abiotic stress seems to be evolutionarily ancient and generally present across land plants and streptophyte algae (de Vries and Ischebeck, 2020; Waters and Vierling, 2020). These mechanisms therefore potentially provided plants with a suitable set-up to respond to elevated temperatures on the metabolic level early on. Temperature has a similar effect on various other branches of plant primary and secondary metabolism, and these effects are likewise buffered to minimize their negative outcomes. Together, these measures confer a sort of metabolic plasticity, enabling plants to mitigate the effects of high temperature and to reproduce even in superoptimal temperature conditions. This metabolic plasticity likely became even more important during the colonization of land.
Phenological and phenotypic plasticity help to keep metabolism within limits
Another option to avoid temperature stress is to limit heat exposure. Much of our understanding of these heat-avoidance strategies has been shaped through the study of the angiosperm model organism Arabidopsis. Acclimation to unfavourable thermal conditions can be achieved by phenological changes and/or by adjusting architecture and morphology to minimize the heating up of tissues, especially those that are photosynthetically active (Crawford et al., 2012; Park et al., 2019).
On the phenological level, several transitions throughout a plant’s life cycle are temperature-sensitive, including germination, flowering, and senescence (Ibañez et al., 2017). While selected species flower later on exposure to high temperatures (e.g. several chrysanthemum species; Karlsson et al., 1989; Nakano et al., 2013), multiple studies describe enhanced flowering at warm temperatures (Fig. 2). Flowering can occur 4–7 d earlier per degree Celsius, depending on the species (Fitter and Fitter, 2002; Miller-Rushing and Primack, 2008). Elevated ambient temperature also promotes the formation of new leaves in Arabidopsis, accelerating the progression from the juvenile to the adult vegetative phase (Frei et al., 2014; Springthorpe and Penfield, 2015; Ibañez et al., 2017). Towards the end of an ontogenetic life cycle, elevated temperatures induce early senescence in both vegetative and reproductive tissues (Al-Khatib and Paulsen, 1984; Kim et al., 2020). Collectively, temperature-induced phenological changes often invoke a ‘decision’ to accelerate the transition to the next generation through a switch to reproductive growth and the remobilization of resources. This acceleration ensures the completion of the life cycle by avoiding the risk of aborted reproductive development.
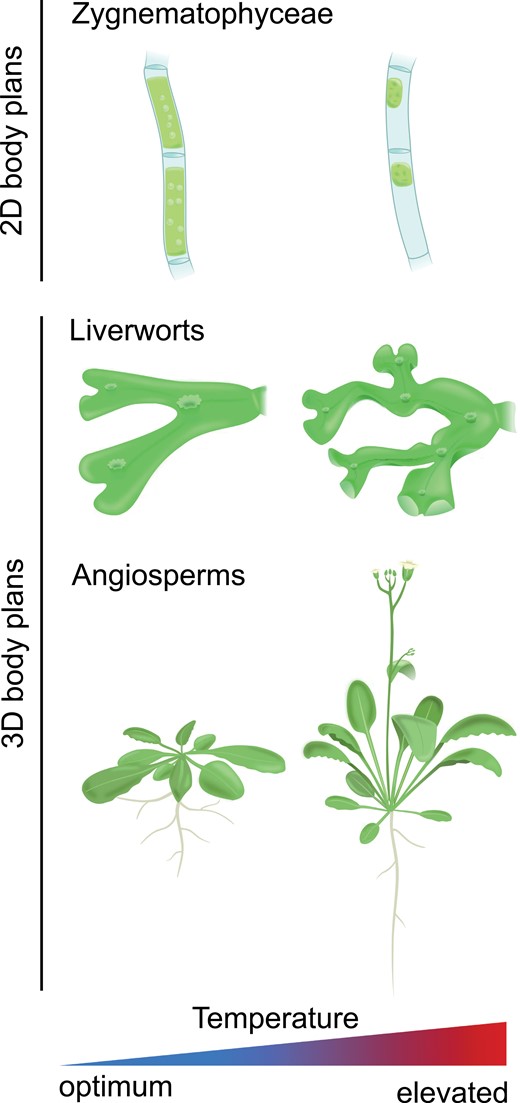
Effects of elevated ambient temperature on the morphology of organisms of different levels of body plan complexity. Temperature phenotypes of 2D-growing green algae (exemplified by Zygnematophyceae—Mougeotia sp.), 3D-growing bryophytes (exemplified by Marchantia polymorpha), and angiosperms (exemplified by Arabidopsis). The images of Mougeotia sp. are based on de Vries et al. (2020).
In addition to phenological plasticity, elevated temperature also induces morphological changes in many plant species. Such architectural adjustments have the capacity to limit the warming of photosynthetic tissues, thereby mitigating negative temperature effects like those described above on plant metabolism (Crawford et al., 2012; Quint et al., 2016; Casal and Balasubramanian, 2019). In general, elongation growth in land plants seems to be promoted by increasing temperatures. Similarly, aquatic plants like morphologically complex streptophyte algae and several submerged macrophytes (embryophytes that have adapted to living in aquatic environments) often show higher growth rates and/or internode elongation at warmer temperatures (Mckee et al., 2002; Auderset Joye and Rey-Boissezon, 2015; Li et al., 2017; Puche et al., 2018). In terrestrial eudicots elongation of hypocotyls is one of the most prominent thermomorphogenic responses of young seedlings (Gray et al., 1998; Koini et al., 2009; Stavang et al., 2009) and is usually accompanied by elongated petioles (Bridge et al., 2013) and upward bending of the leaves (hyponasty; van Zanten et al., 2009). Elongation of hypocotyls and petioles at warm temperatures is largely driven by increased cell elongation. It has been proposed that warmth-induced hypocotyl elongation increases the distance between sensitive meristematic and photosynthetic tissues and the heat-absorbing soil (Gray et al., 1998). Hyponasty is achieved through asymmetrical cell elongation with adaxial (lower side) cells elongating more than those on the abaxial (upper) side of petioles, resulting in an upward bending of the leaves (Polko et al., 2015). These early thermomorphogenic responses result in an open rosette structure (Fig. 2), allowing for enhanced air circulation and increased cooling capacity (van Zanten et al., 2010; Crawford et al., 2012; Bridge et al., 2013; Park et al., 2019). This response is supported by quickly enhancing stomatal aperture upon exposure to high temperatures, enabling transpirational cooling that results in a significant reduction in leaf temperature (Kostaki et al., 2020). This rapid and plastic response may be the fastest (in terms of time after the temperature stimulus) physiological response to elevated ambient temperatures (Gommers, 2020; Kostaki et al., 2020).
A similar effect for thalli of the liverwort Marchantia polymorpha is shown in Fig. 3. We observed a different thallus morphology for plants that have been acclimated at high temperature (28 °C) compared with those that have been acclimated at 20 °C (Fig. 3A). As previously observed for A. thaliana, the more erect morphology of high temperature acclimated plants is associated with more effective tissue cooling when exposed to superoptimal temperature also in the liverwort model (Fig. 3B). This suggests that thermomorphogenic protection of photosynthetic tissue may be a feature conserved across land plants. Thermomorphogenic growth is, however, not restricted to architectural adjustments in this ‘phylotactic’ sense. The morphology of various plant organs can change in response to ambient temperatures. For example, leaf thickness of various species is decreased by elevated ambient temperatures and accompanied by an overall smaller size of the leaves (e.g. Smith and Nobel, 1978; Niinemets, 2001; Rodríguez et al., 2015), most likely because thinner and smaller leaves enhance heat dissipation via evaporation and convection (Franklin and Wigge, 2014). Interestingly, elevated temperature not only affects stomatal aperture, it can also promote (Hu et al., 2014) or repress (Lau et al., 2018) stomatal development depending on the species.
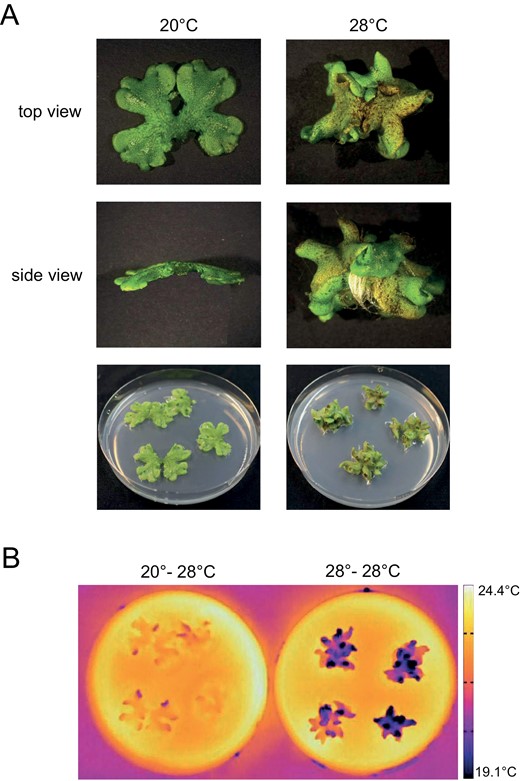
Impact of elevated temperature on Marchantia polymorpha morphology. (A) Photographs showing representative M. polymorpha plants grown at 20 °C or 28 °C for 21 d. Plants were grown under long day conditions (90 μmol m−2 s−1, white light, 16:8 h photoperiod). (B) Thermal images of 3-week-old M. polymorpha plants. Plants were grown as in (A). After equilibration to room temperature, plants were incubated for 15 min at 28 °C. Then thermal images were taken with a forward-looking infrared camera.
In general, it is reasonable to assume that the overarching function of thermomorphogenic growth during vegetative development is to reduce the exposure of photosynthetic tissues to damaging temperatures. This probably reduces the negative effect of high temperature on plant metabolism and protects against temperature-induced production of reactive oxygen species due to disturbances in reaction cascades or metabolic fluxes. It therefore seems that the ecophysiological purpose of selected shoot thermomorphogenic responses is understood reasonably well. In contrast to the shoot, very little is known about root responses to elevated temperatures and this will be subject to discussion later in this review. From an agricultural perspective, it is tempting to predict the generation of climate-resilient crops on the basis of a thorough understanding of thermomorphogenesis. However, it should be noted that shoot thermomorphogenesis often comes at the expense of biomass accumulation (Foreman et al., 2011). This needs to be considered in cost–benefit analyses, especially in comparison with other strategies.
Thermomorphogenesis signalling
Over the past 10–15 years, the molecular pathways underlying shoot thermomorphogenesis have started to be uncovered in the A. thaliana model system. Currently, very little is known about the molecular basis of root thermomorphogenesis (reviewed in the same issue of this journal by Fonseca de Lima et al., 2021). The best studied shoot thermosensory pathway is the temperature-dependent function of phytochrome B (phyB) (Jung et al., 2016; Legris et al., 2016). At low ambient temperatures, active phyB interacts with members of the PHYTOCHROME-INTERACTING FACTOR (PIF) family of bHLH transcription factors and promotes their degradation. Warm temperature enhances the conversion of phyB from the active Pfr to the inactive Pr form. This stabilizes PIFs, which then induce auxin biosynthesis and promote cell elongation (reviewed in Delker et al., 2017). On an additional regulatory level, the DE-ETIOLATED 1 (DET1)–CONSTITUTIVE PHOTOMORPHOGENIC 1 (COP1)/SUPPRESSOR OF PHYTOCHROME A-105 (SPA) signalling pathway targets the bZIP transcription factor ELONGATED HYPOCOTYL 5 (HY5) for degradation (Ang et al., 1998; Osterlund et al., 2000; Schwechheimer and Deng, 2000). Under elevated temperatures this de-represses PIF4 action (Delker et al., 2014; Gangappa and Kumar, 2017). In A. thaliana seedlings, warm temperatures promote PIF stabilization in the cotyledons and this increases auxin flow to the hypocotyl. In the hypocotyl, auxin induces elongation growth via the activation of brassinosteroid (BR) biosynthesis and signalling (Ibañez et al., 2018; Martínez et al., 2018; Bellstaedt et al., 2019). Given that auxin biosynthesis genes are infrequently present in streptophyte algae (Nishiyama et al., 2018), and ‘true’ AUX/IAA signalling genes, to the best of our knowledge, emerged later in the tracheophyte lineage (Mutte et al., 2018; Nishiyama et al., 2018), it is reasonable to assume that auxin-dependent thermomorphogenesis developed after the colonization of land. Despite their lack of canonical AUX/IAA proteins, we cannot rule out that a rudimentary auxin pathway could have played a role in thermomorphogenesis in streptophyte algae. As BR signalling was suggested to have evolved during the diversification of bryophytes and vascular plants (Rensing et al., 2008; Santner and Estelle, 2009; Ross et al., 2010), its role in temperature-dependent growth may have been established even later than auxin. In addition to phytochromes, temperature also regulates the activity of phototropins (Ma et al., 2016; Kostaki et al., 2020) and UVB-RESISTANCE 8 (UVR8; Findlay and Jenkins, 2016), and it has been proposed that other photoreceptors could display temperature-dependent activation (Hayes, 2020).
All of the signalling components described above were previously shown to regulate photomorphogenic responses (Casal and Qüesta, 2018). In many ways, shoot thermomorphogenesis signalling and the corresponding phenotypic outputs mirror those of shade-avoidance signalling (Lorrain et al., 2008; Fraser et al., 2016). Both pathways promote elongation growth by utilizing the same signalling components. Furthermore, as in land plants, shade or reduced light intensities also trigger internode elongation in aquatic charophytes (Schneider et al., 2006). In most aquatic environments, variations in light intensity and quality are more pronounced than variations in temperature, which are comparably well buffered in water. This suggests that in aquatic environments light has been the dominant environmental stimulus, opening the possibility that light signalling pathways were co-opted to enable plant responses to temperature variations. If so, co-option of an established signalling network may have been an efficient way to evolve a first layer of protection against (mildly) detrimental temperatures and to integrate responses to both stimuli. It is reasonable to assume that plant life in shallow water already experienced more pronounced temperature fluctuations even before or parallel to the initial colonization of land. Hence, the ability to sense and respond to elevated ambient temperature may have provided a fitness advantage in both shallow water and on land. Whether this signalling cascade was, in this hypothetical case, used to trigger growth or metabolic responses remains unknown.
Recently, additional plant thermosensors have been discovered that are based on either prion-like proteins undergoing temperature-sensitive phase-separation (Jung et al., 2020) or temperature-dependent mRNA secondary structures affecting translational efficiency (Chung et al., 2020). Both of these (likely ancient) mechanisms affect signalling components that are involved in the same light signalling pathways as described above. The targeting of these components via phase-separation or mRNA secondary structures may represent a more recent fine-tuning of the pathway. However, at this point this conclusion remains rather speculative.
Thoughts about the evolutionary origin(s) of thermomorphogenesis
Our current understanding of thermomorphogenesis and its underlying regulatory mechanisms is defined by the acclimation responses of selected angiosperms with complex body plans. The capacity for thermal acclimation via morphological adjustments may have been less pronounced in early land plants due to their reduced morphological complexity. As a result, the ability to acclimate morphology to elevated temperatures was likely comparatively limited. Little has, however, been reported about non-flowering plants. Consequently, any assumptions about other plant lineages remain hypothetical, including the ones put forward below.
Despite their simple body plan, unicellular green algae do respond to elevated temperatures on the morphological as well as on the cellular level. Cell volume and surface area of the chlorophyte alga Dunaliella tertiolecta, for example, responds to a temperature gradient from 12 °C to 25 °C. With increased temperature, cell volume and surface area decrease, whereas cell division rates increase (Eppley and Sloan, 1966). As many chlorophyte genomes, including that of D. tertiolecta (or Chlamydomonas reinhardtii, see Fig. 4), lack phytochromes, the most parsimonious explanation would be that these temperature responses are regulated via mechanisms that are distinct from the above described Phy–PIF cascade. These mechanisms may be as simple as passive thermodynamic processes or, alternatively, as of yet unknown thermosensing and signalling pathways that are at work in these organisms. Temperature responses have also been described among multicellular, but planar growing members of the streptophyte algae. Under short term heat stress, for example, plastids from filamentous and unbranched Mougeotia sp. (micellous green algae from the Zygnemataceae) show a decrease in length, width, and area (de Vries et al., 2020). This is reminiscent of temperature-dependent chloroplast movements in A. thaliana and M. polymorpha (Łabuz et al., 2015; Sakata et al., 2019), all of which seem to aid in the protection of the chloroplasts from excessive damage due to elevated temperature or heat stress. Although most sequenced Zygnemataceae included in our analysis (Mougeotia sp., Spirogyra sp., Netrium digitus, Penium margaritaceum, Mesotaenium endlicherianum, and Spirogloea muscicola) as well as Chara braunii (Charophyceae) and Klebsormidium nitens (Klebsormidiophyceae) seem to possess phytochromes and also downstream components of the established light/thermomorphogenesis signalling pathway (Fig. 4; Ludwig et al., 2021; http://doi.org/10.5281/zenodo.4945381), chloroplast movements are usually controlled by blue light. In the case of some streptophyte algae this can be more complicated due to the presence of NEOCHROMEs, chimeras of phytochromes and phototropins (Suetsugu et al., 2005). Unfortunately, molecular data on the regulatory network(s) controlling such responses in green algae are scarce, but it appears that a basal capacity to respond to temperature cues was established before plant terrestrialization (at least in shallow water with temperature variations). There is, however, no evidence that temperature-induced changes in these morphologically often simple organisms are evolutionarily related to the architectural responses in land plants described above (Fig. 2). The evolutionary origin of this architectural type of thermomorphogenesis is likely to have been in species with more complex body plans. A full set-up of the Phy–PIF signalling pathway described in Fig. 4 would enable such plants to utilize an established mechanism for plastic growth responses. Distinct, unknown thermosensing and signalling mechanisms may likewise exist, maybe even within the same species in a tissue-/organ-specific manner.
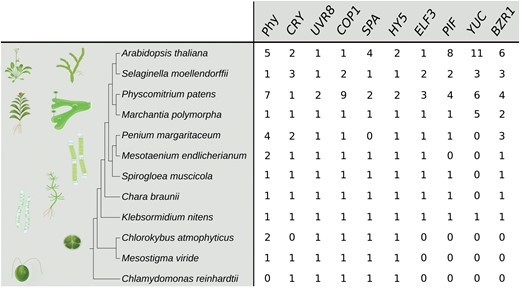
Copy number of genes involved in thermomorphogenesis signalling in various plant genomes based on the Arabidopsis protein and coding sequences and a combination of HMMER (Potter et al., 2018; http://hmmer.org/) and BLASTp (Altschul et al., 1990) searches of available genome data (an extended version of this table including methodological details and alignments can be found at http://doi.org/10.5281/zenodo.4945381) as in Schumann et al. (2011). BZR1, BRASSINAZOLE-RESISTANT 1; COP1, CONSTITUTIVE PHOTOMORPHOGENIC 1; CRY, CRYPTOCHROME; ELF3, EARLY FLOWERING 3; HY5, ELONGATED HYPOCOTYL 5; PIF, PHYTOCHROME-INTERACTING FACTOR; Phy, PHYTOCHROME; SPA, SUPPRESSOR OF PHYA-105; UVR8, UVB-RESISTANCE 8; YUC, YUCCA FLAVIN MONOOXYGENASE.
Another layer of temperature protection that was already in place before plant-specific mechanisms evolved involved HEAT SHOCK PROTEINs (HSPs), which are highly conserved throughout plants and other organisms (Lindquist, 1986). To maintain proper protein folding and functions under different stress settings, HSPs are involved in important processes like protein assembly and conveyance of proteins across membranes (reviewed in Vierling, 1991). HSP gene expression is up-regulated under different abiotic stresses such as heat, drought, salinity, and high temperature. As several HSPs are basally expressed and/or up-regulated also in non-stressful temperatures (reviewed in Al-Whaibi, 2011), they can already protect proteins when temperatures are anticipated to become stressful. As such, HSPs were probably among the first components that evolved to protect cells from temperature damage.
Thermomorphogenesis as a consequence of three-dimensional growth
Despite the fact that two-dimensional (2D) algae are able to change their morphology in response to temperature cues, being able to grow in three spatial dimensions dramatically enhances these possibilities. Three-dimensional (3D) morphology is not exclusive to land plants. Already prior to plant terrestrialization several aquatic algal lineages evolved complex body plans. In Charophytes, for example, complex, upright and branched body plans can be found (e.g. Chara braunii; Delwiche and Cooper, 2015; Harrison, 2017) in addition to unicellular species (e.g. Mesostigma viride), multicellular unbranched filaments with no apical growth (e.g. Klebsormidium sp.), and branching filaments with apical growth (e.g. Coleochaete pulvinata and some Zygnematophyceae). Furthermore, several Coleochaete spp. display perpendicular cell growth (Delwiche et al., 2002). The earliest land plants likely possessed at least branching filaments, and potentially parenchymatous growth (reviewed in Fürst-Jansen et al., 2020). In contrast to most terrestrial algae, which typically grow in filamentous or planar 2D forms, embryophytes exhibit an elaborate 3D growth with multiple axes (Harrison, 2017). 3D growth facilitates increased morphological variation, enabling plants to respond in a plastic manner to environmental cues like elevated temperatures. Moreover, the acquisition of 3D growth opened new ecological niches simply by the plants growing taller. Acquiring vasculature allowed plants to further increase their body size (Delwiche and Cooper, 2015). Increased height resulted in better access to photosynthetically active radiation. This required an extensive system to allow transport of assimilates, nutrients, minerals, and water, accompanied by higher levels of transpiration. Transpiration is especially important for the above described evaporative leaf cooling mechanism. Increased height also meant significant differences in light and temperature conditions to which different parts of the same plant are exposed in their own microclimates. Extant angiosperms, for example, can have light- and shade-adapted leaves on the same plant that differ considerably in their morphology. An increased capacity for phenotypic plasticity, even within the same individual, may have been a prerequisite for efficient utilization (and remobilization) of resources and to successfully colonize diverse terrestrial environments. In this system, temperature alterations are, presumably, almost always accompanied by variations in light quantity and quality, due to (self) shading. Again, to safeguard efficient plant metabolism across a range of temperatures, neo-functionalization of light signalling components would have been an efficient solution (Legris et al., 2017).
Thermomorphogenesis in bryophytes
As bryophytes were likely among the first embryophytic lineages to acquire a high degree of phenotypic plasticity via the ability to grow in all three dimensions, they represent a suitable system to investigate the initial evolution of 3D growth-based thermomorphogenesis. Bryophytes are typically small in size (millimetres up to a few centimetres in height) compared with vascular plants which can reach up to 100 m (e.g. Sequoia sempervirens). They lack complex tissue organization, but possess structures resembling stems, roots, and leaves during the gametophyte stage. Mosses grow small leaflets, but in hornworts and liverworts the main photosynthetic tissues are known as thalli. Bryophytes have a poorly developed hydraulic system because they lack water conduits equivalent to those in tracheophytes. A recent study suggested a reductive evolution of stomata, further depriving them of a regulated water homeostasis (Harris et al., 2020). Obviously, these are both features that are prerequisites for evaporative leaf cooling as described above for angiosperms. Although classic hyponastic growth is unlikely, folding and bending of thalli as illustrated in Fig. 3A for M. polymorpha could be an option to decrease the temperatures experienced by these tissues. We hypothesize that in the absence of controlled evaporative cooling of photosynthetic tissues, these altered morphological properties of folded thalli may be sufficient for plant cooling. Romero-Montepaone et al. (2021) recently suggested that the ecological function of warmth-induced hypocotyl elongation is to enhance the access of shaded photosynthetically active tissues to light. However, the above described observations in M. polymorpha would likewise corroborate the hypothesis Bill Gray initially proposed for A. thaliana in what was most likely the first modern mechanistic thermomorphogenesis paper published (Gray et al., 1998): ‘Hypocotyl elongation elevates the photosynthetic and meristematic tissues away from the heat-adsorbing soil and may allow the plant to take better advantage of the cooling effect of moving air.’ If so, increased transpiration of warm acclimated plants at elevated temperatures (Crawford et al., 2012) would represent a mechanism that acts ‘on top of’, and thereby in concert with, tissue elevation to further enhance the cooling effect.
As a consequence of their morphology, bryophytes are poikilohydric (lacking the ability to actively regulate and maintain their water content). Poikilohydric plants largely depend on the humidity from the environment and thus typically inhabit sheltered and humid environments (Dilks and Proctor, 1979; Proctor and Tuba, 2002; Ligrone et al., 2012; Merced and Renzaglia, 2017). In contrast to vascular plants, it is possible that bryophytes are more likely affected by an interplay of elevated temperature and desiccation rather than light. In fact, several studies indicate an increased tolerance towards elevated temperature under decreased relative humidity for some bryophytes (Meyer and Santarius, 1998; He et al., 2016). In addition, tissue temperature of the moss Grimmia pulvinata was shown to exceed the ambient air temperature by up to 15 °C when dry on sunny days, while it maintained tissue temperature similar to its surrounding when wet (Proctor, 2011). When viewed from the perspective of a bryophyte population in their respective habitat, thermomorphogenic growth as shown in Figs 2 and 3 might lead to a dense plant mat or cushion that maintains humidity and preserves the population from desiccation.
The role of roots in thermomorphogenesis
Thermomorphogenesis research has so far largely focused on shoot organs and relatively little is known about root responses to elevated temperatures. Roots are thought to have emerged at least twice independently, in lycophytes and euphyllophytes (Raven and Edwards, 2001). The innovation of an anchor within the soil provided plants with structural support and a mechanism of water uptake. Roots show a remarkable degree of phenotypic plasticity. Root morphology and anatomy is determined by many different environmental factors, including soil compactness, nutrient availability, salinity, water potential, and also temperature (see also van Zanten et al., 2021 in this issue).
How plant roots sense temperature is currently unknown. The soil environment differs greatly from the surface. The soil is (like a body of water) buffered against rapid temperature changes. Nevertheless, the upper soil layer can easily heat up to a higher extent than the aerial shoot environment and temperature alterations of all soil levels are highly dependent on its hydration status (Yang and Wang, 2008; Blight, 2009). Moreover, soil is dark. The darkness underground would seem to suggest that temperature-sensitive photoreceptors that coordinate light with temperature information are likely not the primary mechanism by which roots perceive temperature. The slow rate of temperature fluctuations in the soil may mean that rapidly responsive sensors like phyB are not necessary for thermosensing in the deepest of roots. As an aside, buffered soils may mean that temperature sensing in the roots is more attuned to long term changes in average temperature (such as those between seasons). If this is the case, it could be that root temperature provides important information to the shoot. In the spring, for example, cool soils might limit temperature responsiveness of the shoot. Root zone temperature has been shown to affect shoot growth (e.g. Al-Rawahy et al., 2019; Vapaavuori et al., 1992; Lam et al., 2020), but evidence of direct signalling is currently lacking. In any case, it seems logical that the drastically different environments between soil and air open the possibility that temperature sensing mechanisms fundamentally differ between roots and shoots.
As discussed above, temperature sensing in shoots may have been co-opted from light signalling pathways. It is possible that root temperature sensing also emerged from an existing signalling network. One environmental factor in the soil that is often correlated with temperature is soil water potential (Hoerling, 2017). Cool temperatures are often associated with wetter soils, and warm temperatures with dryer soils (at least in temperate climate zones). Interestingly, soil temperature and water availability tend to have similar effects on root architecture. At low temperatures, root elongation is inhibited. Main root elongation increases as temperatures rise. When temperatures reach a certain threshold, root elongation is again inhibited (Koevoets et al., 2016). When water potential is high (i.e. when water is abundant) root elongation is slow. As water potential decreases, root elongation is promoted. When water potential decreases below a certain threshold, root elongation is again repressed (Seijo et al., 1997; Dong et al., 2020). The effect of temperature and soil water potential on root anatomy is also similar. Mildly increased temperature tends to result in a shorter root meristem (Yang et al., 2016; Martins et al., 2017; Feraru et al., 2019; Gaillochet et al., 2020). Mild water stress has been shown to have a similar effect on the root meristem in several angiosperms (Seijo et al., 1997; Ji et al., 2014).
It is conceivable that the deeper rooting structure promoted by both warm temperatures and mild water stress acts to increase water uptake. It is well documented that deep rooting can protect plants against drought (Thorup-Kristensen et al., 2020). As discussed above, warm temperature promotes evaporative cooling of the leaves in vascular plants (Crawford et al., 2012). Enhanced evaporative cooling presumably increases the demand for water from the roots, and so deeper rooting may help to compensate for increased water loss. It should, however, be noted that root elongation at warm temperatures is probably not driven solely by increased water demands from the shoot, as dissected roots also elongate in response to warm temperatures (Bellstaedt et al., 2019).
Our current understanding of ambient root temperature signalling is limited (see review by Fonseca de Lima et al., 2021). So too is our understanding of root elongation under mild water stress because the majority of studies on water stress have been conducted under severe drought conditions (Claeys et al., 2014). This lack of data makes it difficult to draw comparisons between the signalling pathways that underlie these responses. It has been proposed that root elongation at warm temperatures requires the modulation of brassinosteroid signalling (Martins et al., 2017). Mild water stress-induced elongation could also be regulated through brassinosteroid signalling. Recently, it was shown that the roots of mutants deficient in ARABIDOPSIS THALIANA SHAGGY-RELATED KINASE 11 (AtSK11) and AtSK12 (two homologues of BRASSINOSTEROID-INSENSITIVE 2) were hypersensitive to mild water stress conditions (Dong et al., 2020). Warm ambient temperatures also promote auxin activity at the root tip (Shibasaki et al., 2009; Wang et al., 2016; Feraru et al., 2019). A similar increase in root tip auxin signalling was shown for tobacco plants exposed to moderate drought stress (Wang et al., 2018). Another hormone that could play a role is abscisic acid (ABA). It is well established that ABA participates in the inhibition of root growth in response to drought stress (Sharp et al., 2004). In Arabidopsis roots, around a third of the genes regulated by transient exposure to 28 °C (Martins et al., 2017; Bellstaedt et al., 2019) are also regulated by short term ABA treatments (Yang et al., 2016), indicating that there is overlap between these signalling networks. Furthermore, homologous genes of the land plant ABA signalling cascade are known to be widely present in streptophyte algae. All of them were found in the Zygnomatophyceae (de Vries et al., 2018; Cheng et al., 2019; Sun et al., 2019) providing a possible framework of ABA signalling in the last common ancestors of land plants.
Despite these insights, dissecting genetic relationships and the roles of different hormones in temperature-induced root elongation is not straightforward. Roots are more sensitive to hormonal imbalance than the shoot. Brassinosteroids, ABA, and auxin all promote root elongation at low concentrations and repress root elongation at high concentrations. In addition to this, hormonal mutants often display pleiotropic phenotypes and show severe root elongation defects even at optimal temperatures. More subtle approaches, including tissue-specific and inducible knock-downs, may help to clarify the role of hormones in warm temperature-induced root elongation.
Taken together, our superficial understanding of ambient temperature and mild drought signalling pathways in roots means that we cannot conclusively say that the two pathways are interrelated, but the information we currently have is at least consistent with this hypothesis. Further research into this area is needed to clarify this point. In any case, understanding the integration of drought and temperature signals may open new avenues in generating climate-resilient plants as both abiotic stressors tend to go hand in hand.
Conclusion
Cellular and morphological responses to temperature changes seem to generally have the goal of maintaining plant physiology and metabolism stable over a wide range of ambient temperatures and to prevent damage. Whilst 2D-growing algal lineages with less complex body plans are able to respond to temperature changes on a cellular level, thermomorphogenesis in the sense of architectural acclimation is likely closely related to the emergence of 3D growth. Here, it is feasible that thermomorphogenesis signalling evolved at least two times independently. In the absence of experimental data, we have to base our hypotheses on environmental and physiological circumstances that likely prevailed at the time of emergence of thermomorphogenesis. Possibly, thermomorphogenesis signalling first developed in co-option with desiccation stress as during the transition from water to land temperature alterations were more likely expected to coincide with drought than with light. With the development of a complex vasculature, plants became less dependent on the humidity of their microhabitat and due to the increased size, fluctuations of temperature were presumably more connected to differences in light rather than desiccation (Fig. 5). To resolve this point, it will be important to study whether thermomorphogenesis already existed in morphologically complex aquatic algae like Chara braunii. These algae possess a full set-up of the Phy–PIF signalling pathway (Fig. 4) but reside in entirely aquatic environments. If they do not exhibit thermomorphogenic traits, it would lend weight to the idea that temperature sensing first emerged from a drought signalling pathway. As roots of land plants mostly occupy a humidity-based environment (soil), it is tempting to speculate that root thermomorphogenesis is driven by pathways similar to those that exist in bryophytes. If there is an overlap between these signalling pathways in bryophytes and the roots of vascular plants, it could open up potential avenues to understanding thermomorphogenesis in both of these systems.
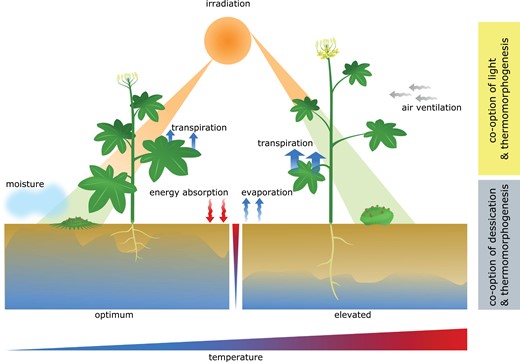
General overview of possible temperature responsive growth patterns in bryophytes and vascular plants. To keep plant metabolism stable, elevated temperatures influence angiosperm morphology in numerous above- and belowground organs. To date, very little is known about thermomorphogenesis in algae, bryophytes, and other non-seed plants. Growth patterns displayed in this figure and in the text are therefore largely speculative and based on environmental and physiological circumstances. During the transition from (shallow) water to land, temperature fluctuations most likely occurred together with drought and changes in light, facilitating the possibility for co-option of pre-existing signalling pathways. Considering the most likely ecological niche/microhabitat of early land plants, it seems a reasonable theory that drought signalling could have initially given rise to thermomorphogenesis signalling. Such a thermosignalling pathway may have persisted until today in roots of vascular plants and throughout bryophytes. In addition, co-occurrence of changes in temperature with light intensity and quality might have resulted in the emergence of another temperature signalling pathway that has been co-opted from light signalling. This pathway is currently the best understood temperature signalling pathway, controlled by the master regulator PIF4. The chronological sequence of emergence of these different thermosignalling pathways is as yet unclear.
The lack of experimental data on thermomorphogenesis from diverse species is reflected in the speculative character of large parts of this review. To fill the existing gaps, it would be helpful to generate temperature-responsive phenotypic (and molecular) data across all major lineages of land plants, including ferns and lycophytes. These studies should be complemented by streptophyte algae as the closest aquatic relatives of land plants to identify when thermomorphogenesis first evolved. This would also enable us to study thermoresponsive growth and development in aquatic versus terrestrial environments in an ecological context. Understanding the evolution of thermomorphogenesis will likely lead to exciting discoveries on the molecular mechanisms that govern this process. These discoveries could well pave the way towards hardier crops in a warming world.
Acknowledgements
We wish to thank Martijn van Zanten for inspiring comments on the manuscript. Likewise, we thank the two anonymous reviewers for excellent input on several evolutionary aspects of this story. The thermocamera for the images in Fig. 3B was provided by Edgar Peiter. This project was carried out in the framework of MAdLand (http://madland.science, DFG priority programme 2237). MQ is grateful for funding by the DFG (Qu 141/10-1, Qu 141/3-2). SH is funded through a Wageningen Graduate School (WGS) Post-Doctoral Talent Fellowship.
Author contributions
WL conducted the M. polymorpha experiments and prepared the figures. All authors contributed to writing of the manuscript.
Data availability
Extended version of Fig. 4 (additional species/genomes), alignments of the identified homologues of potential thermomorphogenesis signalling genes, sequence files, and a more detailed description of the methodological approach used to identify potentially homologous sequences are available at Zenodo http://doi.org/10.5281/zenodo.4945381; Ludwig et al., (2021).
Comments