-
PDF
- Split View
-
Views
-
Cite
Cite
Chen Yuan, Hongmei Li, Cheng Qin, Xian Zhang, Qianqian Chen, Pengcheng Zhang, Xiaorui Xu, Meiling He, Xinlian Zhang, Mahmut Tör, Dawei Xue, Huizhong Wang, Stephen Jackson, Yuehui He, Yule Liu, Nongnong Shi, Yiguo Hong, Foxtail mosaic virus-induced flowering assays in monocot crops, Journal of Experimental Botany, Volume 71, Issue 10, 30 May 2020, Pages 3012–3023, https://doi.org/10.1093/jxb/eraa080
- Share Icon Share
Abstract
Virus-induced flowering (VIF) exploits RNA or DNA viruses to express flowering time genes to induce flowering in plants. Such plant virus-based tools have recently attracted widespread attention for their fundamental and applied uses in flowering physiology and in accelerating breeding in dicotyledonous crops and woody fruit-trees. We now extend this technology to a monocot grass and a cereal crop. Using a Foxtail mosaic virus (FoMV)-based VIF system, dubbed FoMViF, we showed that expression of florigenic Flowering Locus T (FT) genes can promote early flowering and spikelet development in proso millet, a C4 grass species with potential as a nutritional food and biofuel resource, and in non-vernalized C3 wheat, a major food crop worldwide. Floral and spikelet/grain induction in the two monocot plants was caused by the virally expressed untagged or FLAG-tagged FT orthologs, and the florigenic activity of rice Hd3a was more pronounced than its dicotyledonous counterparts in proso millet. The FoMViF system is easy to use and its efficacy to induce flowering and early spikelet/grain production is high. In addition to proso millet and wheat, we envisage that FoMViF will be also applicable to many economically important monocotyledonous food and biofuel crops.
Introduction
Virus-induced flowering (VIF) uses RNA or DNA viruses as vectors to express flowering time genes such as Flowering Locus T (FT) (Kardailsky et al., 1999; Srikanth and Schmid, 2011) to induce flowering in plants (McGarry et al., 2017). This approach has recently attracted wide interest for its practical application in accelerating breeding in crops and woody fruit-trees (McGarry et al., 2017; Qin et al., 2017). The first VIF was established in Cucurbita moschata through ectopic expression of Arabidopsis FT (AtFT) proteins from Zucchini yellow mosaic virus to induce early flowering (Lin et al., 2007; Yoo et al., 2013). Subsequently, a Potato virus X (PVX)-based VIF was developed to express AtFT protein or mRNA to promote flowering in short-day (SD) tobacco (Nicotiana tabacum Maryland Mammoth, MM) under non-flowering long-day (LD) conditions (Li et al., 2009, 2011). Apple latent spherical virus, Citrus leaf blotch virus, and Cotton leaf crumple virus were also later used to express FT for floral induction in soybean, apple, pear, gentian, and lisianthus plants (Yamagishi and Yoshikawa, 2011; Yamagishi et al., 2011, 2014, 2016; Fekih et al., 2016), cotton (McGarry and Ayre, 2012; McGarry et al., 2016), and citrus (Velázquez et al., 2016). More recently, we further characterized and developed the PVX-based VIF approach to assess FT protein function including investigations into the impact of single amino acid mutations on the floral-inducing function of the AtFT protein; the influence of various polypeptide tags on the AtFT activity; and the function of mono- and dicotyledonous FT and FT-like genes to induce flowering in MM tobacco under non-inductive conditions (Qin et al., 2017). These findings demonstrate that VIF represents an efficient system for functional analysis of proteins in flowering and a potential strategy to speed up crop breeding programs.
To date, VIFs have only been developed in dicotyledonous plants (dicots) (McGarry et al., 2017; Qin et al., 2017), but not for monocotyledonous species (monocots) including any economically important cereal crops. Barley stripe mosaic virus (BSMV) has, however, been modified as a functional genomics tool for virus-induced gene silencing (VIGS) in barley (Hordeum vulgare L.) and wheat (Triticum aestivum) (Holzberg et al., 2002; Meng et al., 2009; Pacak et al., 2010; Yuan et al., 2011), while VIGS platforms based on Brome mosaic virus in rice (Oryza sativa L.), barley, and maize (Zea mays L.; Ding et al., 2006), Bamboo mosaic virus in Brachypodium distachyon (Liou et al., 2014), Rice tungro bacilliform virus in rice (Purkayastha et al., 2010), and Cucumber mosaic virus in maize (Wang et al., 2016) have also been reported. Recently, Foxtail mosaic virus (FoMV) was engineered as an effective VIGS system to induce gene silencing in barley, wheat, sorghum (Sorghum bicolor), foxtail millet (Setaria italica), green foxtail (Setaria viridis), and the sweet corn line Golden 3 Bantam (Liu et al., 2016; Mei et al., 2016). Moreover, BSMV and FoMV have been exploited to allow overexpression of endogenous and exogenous proteins in both dicots and monocots (Liu and Kearney, 2010; Bouton et al., 2018; Cheuk and Houde, 2018; Zhang et al., 2019), indicating these viruses have the prospect of being modified as valuable VIF tools in monocots.
FoMV, like PVX, is a positive-sense single-stranded (ss) RNA potexvirus. FoMV can infect 56 Poaceae species and at least 35 dicot species (Paulsen and Niblett, 1977; Short and Davies, 1987). The FoMV genome consists of a 5′-methylguanosine cap and a 3′-polyadenylated tail. It encodes an RNA-dependent RNA polymerase, three movement proteins expressed from the triple-gene block, coat protein (CP), and a small unique 5A polypeptide (Robertson et al., 2000; Bruun-Rasmussen et al., 2008). Similar to PVX, FoMV has been used to silence (Ruiz et al., 1998; Lin et al., 2008; Zhou et al., 2012; Chen et al., 2015a,b; Zhao et al., 2016) or overexpress genes in plants (Liu et al., 2016; Mei et al., 2016; Bouton et al., 2018). To test whether FoMV can be used for VIF (designated FoMViF hereafter) in monocots, we took the advantage of the FoMV vector previously developed in our groups (Liu et al., 2016) to express four flowering time genes including AtFT, rice Hd3a, tomato (Solanum lycopersicum) SFT, and tobacco NtFT4 (Kojima et al., 2002; Lifschitz et al., 2006; Harig et al., 2012) in proso millet (Panicum miliaceum L.) and wheat. We have demonstrated that expression of FT genes from FoMV was able to trigger early flowering in monocot crops and therefore successfully established a virus-induced flowering assay FoMViF in cereal crops.
Materials and methods
Plant materials and growth conditions
Plants of Nicotiana benthamiana, proso millet (Panicum miliaceum L. CGRIS 00000390), and winter wheat (Triticum aestivum L, 2n=6x=42, AABBDD, originated from the elite variety YZ4110; Zhang et al., 2018) were grown under long-day (LD, 16 h light–8 h dark) or short-day (SD, 8 h light–16 h dark) conditions at 23 °C. For vernalization, wheat seeds were sown, germinated and grown at 4 °C for 4 weeks before being transferred to 23 °C under LD for further growth.
Construction of FoMViF vectors
The Arabidopsis, rice, tomato, and tobacco FT homologous genes were PCR amplified using plasmid PVX/AtFT, PVX/Hd3a, PVX/SFT, and PVX/NtFT4 (Qin et al., 2017) as DNA templates, PrimeSTAR HS DNA polymerase and four sets of primers, FoMV-AtFT-F/R, FoMV-Hd3a-F/R, FoMV-SFT-F/R, and FoMV-NtFT4-F/R (Supplementary Table S1 at JXB online), respectively. The PCR products were digested with HpaI and AscI, and cloned into the HpaI/AscI sites of the FoMV-sg vector (Liu et al., 2016) to produce FoMV/AtFT, FoMV/Hd3a, FoMV/SFT, and FoMV/NtFT4 (Fig. 1B). To in-frame fuse the 3×FLAG to each of the FT open reading frame, we first amplified the 3×FLAG sequence using the primer FoMV-FTs-FLAG-R along with FoMV-AtFT-FLAG-F, FoMV-Hd3a-FLAG-F/FoMV-SFT-FLAG-F, or FoMV-NtFT4-FLAG-F (Supplementary Table S1), respectively. The PCR reaction contained 0.5 µl of 10 mM primer F/R, 0.5 µl of 2.5 mM dNTPs, 1 µl of 10 ng/µl template, 2 µl of 10× PrimeSTAR HS DNA polymerase buffer, 0.1 µl of PrimeSTAR HS DNA polymerase and 15.4 µl of ddH2O. The amplification was carried out with 30 cycles of 95 °C for 30 s, 58 °C for 30 s, and 72 °C for 30 s, followed by one cycle of 72 °C for 10 min. The amplified fragments were then assembled by Seamless Cloning (pEASY-Uni Seamless Cloning and Assembly Kit, TransGen Biotech) into the AscI-linearized FoMV/AtFT, FoMV/Hd3a, FoMV/SFT, or FoMV/NtFT4 to generate FoMV/AtFT-FLAG, FoMV/Hd3a-FLAG, FoMV/SFT-FLAG, and FoMV/NtFT4-FLAG, respectively (Fig. 1B). All FoMViF constructs were confirmed by nucleotide sequencing. Each of the FoMViF vectors was mobilized into Agrobacterium tumefaciens GV3101 as previously described (Hong et al., 1996) and confirmed by PCR. Agrobacterium glycerol stocks were made and kept at −80 °C in a freezer.
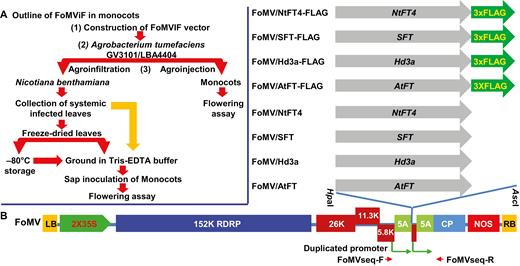
A FoMV-induced flowering (FoMViF) assay in monocotyledonous plants. (A) Outline of the FoMViF procedure in monocots. (B) FoMV vectors for expression of florigen genes. The genome of Foxtail mosaic virus (FoMV) encodes a 152 kDa RNA-dependent RNA polymerase (RDRP), three movement proteins (26, 11.3, and 5.8 kDa), coat protein (CP), and the small 5A polypeptide. The duplicated CP subgenomic RNA promoters are indicated. The cDNA copy of the FoMV genomic RNA is under the control of the enhanced CaMV 35S promoter and the NOS terminator in a plant binary vector (Liu et al., 2016). The left and right borders (LB and RB) of the Ti-plasmid are indicated. Arabidopsis AtFT, rice Hd3a, tomato SFT, and tobacco NtFT4 and their FLAG-tagged genes were cloned downstream of the duplicated CP promoter into the HpaI/AscI sites of the FoMV vector. The positions of primers FoMVseqF/FoMVseq-R (Table S1) which were used for colony-PCR screening, sequencing and RT-PCR detection are indicated along the FoMV genome.
Enrichment of FoMV vectors in Nicotiana benthamiana
Agrobacterium tumefaciens GV3101 containing each of the FoMV vectors were freshly cultured from Agrobacterium stocks. Overnight cultures were then centrifuged at 6797 g for 10 min and resuspended in sterile distilled water to a final OD595 of 1.0. Agrobacterium suspension (OD595: 1.0) was then infiltrated into young leaves of N. benthamiana at six-leaf stage through a needleless 0.5-ml syringe. Plants were then grown in an insect-free growth room at 23 °C under LD conditions. FoMV infection normally caused mild leaf curling and mosaic symptoms on systemic leaves at 10–14 d after agroinfiltration. At this stage, young leaf tissues were collected and used directly for FoMViF assays or freeze-dried and stored at −80 °C in a freezer for later use.
FoMViF in proso millet and wheat
Sap was prepared by grinding 1 g of leaf tissues of healthy or FoMV-infected N. benthamiana in 1 ml TE (10 mM Tris–1 mM EDTA buffer, pH 8.0). Young leaves of five to eight proso millet plants or wheat plants without vernalization treatment at two- to three-leaf stage (17–26 d after sowing seeds; DASS) were mechanically inoculated with sap (Liu et al., 2016). Such inoculation was handled with care because heavy-handed rubbing of leaves sometimes damaged young plants and led them to premature death. For mock controls in all FoMViF experiments, plants were inoculated with healthy N. benthamiana leaf sap prepared in TE buffer. Plants were then grown at 23 °C under the non-inductive LD conditions in insect-free growth rooms. Local and systemic viral symptoms, flowering time and spikelet development in millet and wheat plants were daily examined after sap inoculation, and phenotypically recorded using a Nikon D7000 camera. Sap inoculation of proso millet or wheat often resulted in 100% plant infection. Each set of FoMViF assays was repeated at least twice. For statistical analysis, equal variances and a two-tailed Student’s t-test against FoMV were used out to verify if changes of flowering time in these FoMViF assays would show statistically significant differences.
RT-PCR
Total RNA was extracted from proso millet and wheat leaf tissues with obvious viral symptoms at 7–14 d post-inoculation. RNA was also isolated from healthy leaves that were mock-inoculated and used as controls. First-strand cDNA was synthesized from DNase I-treated total RNAs by M-MLV Reverse Transcriptase according to the manufacturer’s instructions (Promega). RT-PCR was performed to detect virally expressed FT RNAs using primers FoMV seq-F and FoMV seq-R. The PCR reaction contained 0.5 µl of 10 mM primer F/R, 0.5 µl of 2.5 mM dNTPs, 2 µl of 1/10 cDNA template, 10 µl of 2×Taq MasterMix (CWBIO), and 8.5 µl of ddH2O. The amplification was carried out with 40 cycles of 95 °C for 30 s, 58 °C for 30 s and 72 °C for 30 s. The resulting RT-PCR products were isolated and directly sequenced using primer FoMVseq-F (Supplementary Table S1) as previously described (Qin et al., 2017). Proso millet and wheat 18S rRNAs were served as the internal control (Supplementary Table S1). Densitometric analysis of RT-PCR bands was performed using ImageJ software (National Institutes of Health) to show the stability of recombinant viruses. Intensity of the larger or small bands in individual agarose gels was measured using the ImageJ software following the supplier’s guidance. The relative RNA levels were determined by the ratio of intensities of upper (recombinant virus) band against lower (wild-type virus) band.
Protein extraction and immumoblot analysis
Total protein was extracted from symptomatic young leaf tissues at 7–14 d post-inoculation using extraction buffer containing 50 mM Tris, pH 7.5, 150 mM NaCl, 2 mM EDTA, 5% glycerol, 0.1% Tween 20, 1 mM dithiothreitol, and 0.2 mM PMSF (Hong et al., 1996). Protein was also extracted from healthy leaves that were mock-inoculated, collected, and used as controls. Ten micrograms of total proteins were separated by electrophoresis on 10% sodium dodecyl sulfate (SDS)-polyacrylamide gels, transferred to a nitrocellulose membrane (Bio-Rad), blotted with 1:2000 mouse anti-FLAG (Sigma-Aldrich) antibodies, and detected by 1:5000 goat anti-mouse IgG horseradish peroxidase-conjugated secondary antibodies (Santa Cruz Biotechnology) following the ECL chemiluminescence detection protocol. Ponceau S staining was used to show an equal loading of total protein samples.
Results
FoMV-induced flowering in monocots
We have previously described the development of the FoMV-based vector to deliver hairpin double-stranded (ds) RNAs in order to silence genes in monocot plants (Liu et al., 2016). In this vector, the FoMV CP subgenomic RNA promoter was duplicated upstream of the original CP promoter. We envisaged that the duplicated promoter, as with those designed in the PVX vector (van Wezel et al., 2002), could direct the biosynthesis of an extra subgenomic RNA from the recombinant viral genome as mRNA to promote efficient translation of the protein of interest. This is how flowering time genes could be virally expressed via FoMV in monocot plants (Fig. 1). The procedure of the FoMViF assay is outlined in Fig. 1A and is as follows. (i) The FT gene (or any flowering gene) is cloned into the binary FoMV vector (Fig. 1B) and verified by sequencing. (ii) The recombinant FoMV vector is mobilized into Agrobacterium tumefaciens GV3101 or LBA4404. (iii) Agrobacterium carrying the binary FoMV vector can be directly injected into the stem tissues, a procedure called agroinjection, to inoculate monocot plants for FoMViF. Alternatively, we often include an extra step in which the Agrobacterium carrying the FoMV vector is first infiltrated into N. benthamiana leaves via agroinfiltration to enrich the titre of recombinant FoMV inoculum for the subsequent infection of the monocot plants. Systemic N. benthamiana leaf tissues infected with FoMV are collected, and the fresh leaf materials can be either used directly or freeze-dried for storage at −80 °C for later use. Sap is prepared by grinding fresh or dried leaf tissues in 10 mM Tris-EDTA buffer (pH 8.0) and mechanically inoculated onto young leaves of monocot plants. Plants are then grown under LD or SD conditions and monitored for floral induction (Li et al., 2009; Qin et al., 2017).
In this work we cloned both free and 3×FLAG-tagged Arabidopsis AtFT, rice Hd3a, tomato SFT, and tobacco NtFT4 into the FoMV vector (Liu et al., 2016) and produced FoMV/AtFT, FoMV/Hd3a, FoMV/SFT, FoMV/NtFT4, FoMV/AtFT-FLAG, FoMV/Hd3a-FLAG, FoMV/SFT-FLAG, and FoMV/NtFT4-FLAG constructs (Fig. 1B). These FT genes display a range of nucleotide polymorphisms (Supplementary Fig. S1), although at the amino-acid level their protein products are conserved (Supplementary Fig. S2). We investigated inductive and non-inductive conditions for early flowering in proso millet and the winter wheat, and then tested whether these FT genes could be expressed by FoMV and whether viral expression of FT genes could trigger early flowering in proso millet and wheat under non-inductive conditions.
Flowering conditions in proso millet and wheat
In order to test the potential application of FoMViF in monocots, we selected two cereal crops—one is the lesser-studied proso millet whilst the other is the major food crop wheat. Proso millet is a C4 photosynthetic annual grass species that is well adapted to many soil and climatic conditions. It produces grains rich in starch, protein, essential amino acids, and health-promoting phenolic compounds and has a high calcium content, but lacks gluten, and thus it is particularly nutritious for people who cannot tolerate wheat. Moreover, starch derived from proso millet can be readily converted to ethanol. There is an increased demand for breeding new proso millet varieties for human nutrition products and as an alternative to corn for biofuel production (Taylor et al., 2006; Saleh et al., 2012). To test how proso millets respond to photoperiods in our laboratories, we grew it at 23 °C under SD (8 h light–16 h dark), LD (16 h light–8 h dark) or LD/SD mixed conditions (Fig. 2). Under SD plants started to produce millet spikelet at an average of 56 d after sowing seeds (DASS) (Fig. 2A). However, at this time under LD, no spikelet developed, but development of millet spikelet was delayed by 3–5 weeks (Fig. 2B). The appearance of spikelet in proso millet occurred at approximately 88 DASS on average under LD (Fig. 2C, Table 1). We also germinated seeds and grew plants initially under LD for 4 weeks, then changed the growth condition to SD. Under such LD/SD transfer conditions, proso millet started to flower and produce spikelet at about 63 DASS (Fig. 2D). These data show that proso millet is a facultative SD plant, consistent with previous characterization (Kumar et al., 1977).
. | Flowering time (DASS) . | . | . | . | P-valuea . |
---|---|---|---|---|---|
. | Expt I . | Expt II . | Expt III . | Expt I–III . | . |
Mock | 78.2±1.9 | 91.3±2.3 | 92.7±3.3 | 87.4±8.0 | 0.3733 |
FoMV | 74.6±4.6 | 80.0±0.0 | 86.5±5.1 | 80.4±6.0 | n.a. |
FoMV/AtFT | 73.4±6.8 | 72.8±1.5 | 82.8±3.9 | 76.3±5.5 | 0.4892 |
FoMV/Hd3a | 59.6±1.1 | 59.5±1.0 | 68.3±1.5 | 62.5±5.1 | 0.0147 |
FoMV/SFT | 72.0±1.6 | 71.0±0.7 | 83.0±5.0 | 75.3±6.7 | 0.3117 |
FoMV/NtFT4 | 71.6±4.3 | 83.4±0.5 | 86.3±2.9 | 80.4±7.8 | 0.9308 |
. | Flowering time (DASS) . | . | . | . | P-valuea . |
---|---|---|---|---|---|
. | Expt I . | Expt II . | Expt III . | Expt I–III . | . |
Mock | 78.2±1.9 | 91.3±2.3 | 92.7±3.3 | 87.4±8.0 | 0.3733 |
FoMV | 74.6±4.6 | 80.0±0.0 | 86.5±5.1 | 80.4±6.0 | n.a. |
FoMV/AtFT | 73.4±6.8 | 72.8±1.5 | 82.8±3.9 | 76.3±5.5 | 0.4892 |
FoMV/Hd3a | 59.6±1.1 | 59.5±1.0 | 68.3±1.5 | 62.5±5.1 | 0.0147 |
FoMV/SFT | 72.0±1.6 | 71.0±0.7 | 83.0±5.0 | 75.3±6.7 | 0.3117 |
FoMV/NtFT4 | 71.6±4.3 | 83.4±0.5 | 86.3±2.9 | 80.4±7.8 | 0.9308 |
Raw data for flowering time (days after sowing seeds, DASS) in each experiment are shown in Supplementary Table S3. The flowering time (DASS) in each experiment and overall is represented as mean ±SD. As demonstrated in individual experiments (Supplementary Table S3), only viral transient expression of rice Hd3a triggered significantly early flowering. n.a., not applicable.
aP-values generated with equal variances with two-tailed Student’s t-test against FoMV for the overall flowering time are shown. P≤0.05 (shown in bold) is regarded as a statistically significant difference.
. | Flowering time (DASS) . | . | . | . | P-valuea . |
---|---|---|---|---|---|
. | Expt I . | Expt II . | Expt III . | Expt I–III . | . |
Mock | 78.2±1.9 | 91.3±2.3 | 92.7±3.3 | 87.4±8.0 | 0.3733 |
FoMV | 74.6±4.6 | 80.0±0.0 | 86.5±5.1 | 80.4±6.0 | n.a. |
FoMV/AtFT | 73.4±6.8 | 72.8±1.5 | 82.8±3.9 | 76.3±5.5 | 0.4892 |
FoMV/Hd3a | 59.6±1.1 | 59.5±1.0 | 68.3±1.5 | 62.5±5.1 | 0.0147 |
FoMV/SFT | 72.0±1.6 | 71.0±0.7 | 83.0±5.0 | 75.3±6.7 | 0.3117 |
FoMV/NtFT4 | 71.6±4.3 | 83.4±0.5 | 86.3±2.9 | 80.4±7.8 | 0.9308 |
. | Flowering time (DASS) . | . | . | . | P-valuea . |
---|---|---|---|---|---|
. | Expt I . | Expt II . | Expt III . | Expt I–III . | . |
Mock | 78.2±1.9 | 91.3±2.3 | 92.7±3.3 | 87.4±8.0 | 0.3733 |
FoMV | 74.6±4.6 | 80.0±0.0 | 86.5±5.1 | 80.4±6.0 | n.a. |
FoMV/AtFT | 73.4±6.8 | 72.8±1.5 | 82.8±3.9 | 76.3±5.5 | 0.4892 |
FoMV/Hd3a | 59.6±1.1 | 59.5±1.0 | 68.3±1.5 | 62.5±5.1 | 0.0147 |
FoMV/SFT | 72.0±1.6 | 71.0±0.7 | 83.0±5.0 | 75.3±6.7 | 0.3117 |
FoMV/NtFT4 | 71.6±4.3 | 83.4±0.5 | 86.3±2.9 | 80.4±7.8 | 0.9308 |
Raw data for flowering time (days after sowing seeds, DASS) in each experiment are shown in Supplementary Table S3. The flowering time (DASS) in each experiment and overall is represented as mean ±SD. As demonstrated in individual experiments (Supplementary Table S3), only viral transient expression of rice Hd3a triggered significantly early flowering. n.a., not applicable.
aP-values generated with equal variances with two-tailed Student’s t-test against FoMV for the overall flowering time are shown. P≤0.05 (shown in bold) is regarded as a statistically significant difference.
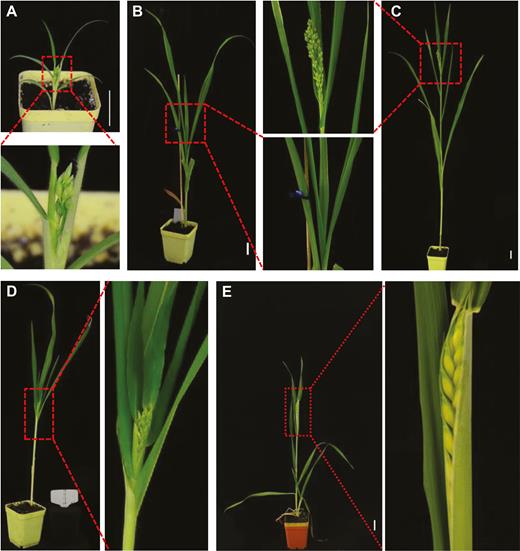
Photoperiod effects on flowering time in proso millet and wheat. (A) Proso millet plant flowered at an average of 56 DASS in SD. (B) Proso millet plant remained vegetative at 56 DASS in LD. (C) Vegetative-reproductive transition in proso millet in LD. Plants started to flower at an average of 88 DASS in LD. (D) Proso millet plant flowered at 63 DASS when grown under LD for 4 weeks and transferred to SD. (E) Flowering time/heading date in wheat. Wheat plants underwent vernalization treatment for 4 weeks, then grew under LD and started to flower (heading) at approximately 90 DASS. Plants were photographed at 56 DASS (A, B); 63 DASS (D); 84 DASS (C), and 90 DASS (E). The boxed sections of each panel are enlarged to show clear phenotypes. Scale bar: 3 cm.
For the hexaploid wheat, vernalization treatment under cold conditions is essential for flowering/heading (Zhang et al., 2019). In our laboratories we found that giving wheat a vernalization period of 4 weeks at 4 °C and then growing at 23 °C under LD conditions (16 h light–8 h dark) induced flowering/heading at approximately 90 DASS (Fig. 2E). Under such conditions, wheat plants with mock inoculation or treated with the empty FoMV or the four FoMViF vectors all started to flower at the same time (Supplementary Fig. S3). Therefore, these conditions could not be used for our FoMViF assays in wheat. However, without vernalization, wheat remained vegetative at 23 °C under LD settings and no flowering/heading was observed at 126 DASS or later. Thus, we choose the less inductive LD (for proso millet), or LD without vernalization treatments (for wheat) to examine whether FoMViF could trigger early flowering/heading and spikelet development in these monocot plants.
FoMViF induces early flowering in proso millet
Although agroinjection could be directly used to inoculate FoMV into monocot plants, we preferred to use higher titer viral inoculum enriched via agroinfiltration of N. benthamiana in our FoMViF assay (Fig. 1A). We mechanically inoculated young leaves of proso millet plants with virus-infected N. benthamiana leaf sap at the three-leaf stage (21 DASS) and found that this led to almost 100% occurrence of FoMV infection (Fig. 3). Compared with proso millet with mock-inoculation (Fig. 3A), plants inoculated with FoMV, FoMV/AtFT, FoMV/Hd3a, FoMV/SFT, or FoMV/NtFT4 developed local infections approximately 1 week post-inoculation (i.e. 28 DASS), as evidenced by development of irregular mosaic and yellowing lesions on the inoculated leaves (Fig. 3B–F). Similar viral symptoms appeared on emerging systemic young leaves and FoMV-FT mRNA was readily detectable by RT-PCR in these leaf tissues although we also detected a smaller band (Fig. 3G; Supplementary Fig. S4; Supplementary Table S2). These results suggest that some recombinant FoMViF viruses lost the inserted FT genes and reverted to FoMV. Systemically infected plants were often dwarfed when compared with mock-inoculated healthy control plants (Fig. 3H–M). However, at an average of 63 DASS, proso millet in which the rice Hd3a florigen was expressed by FoMV/Hd3a developed spikelets (Fig. 3K, Table 1; Supplementary Table S3). Millet spikelets also appeared later in plants that were infected with FoMV/AtFT (Fig. 3J) or FoMV/SFT (Fig. 3L) at 76 or 75 DASS on average, respectively (Table 1; Supplementary Table S3). At this time no flowering or spikelet development was observed in mock-inoculated or FoMV-infected millet plants (Fig. 3H, I). These control plants produced spikelets afterwards, but always later than exogenous FT-expressing plants except for FoMV/NtFT4 that only triggered early flowering in one experiment (Fig. 3M; Table 1; Supplementary Table S3). Taken together, these results demonstrate a successful establishment of FoMViF in proso millet, a monocot grass species.
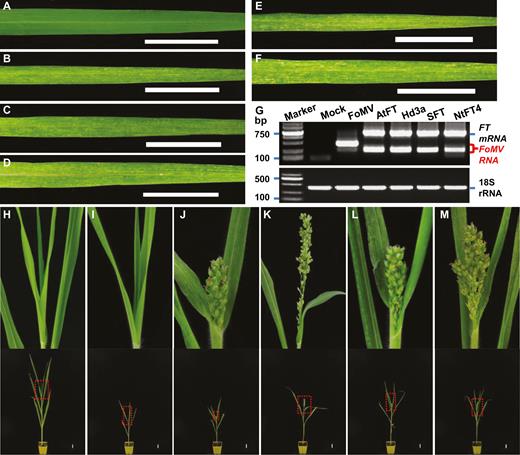
Viral expression of heterologous monocot and dicot florigen genes induces flowering and early spikelet development in proso millet. (A) Healthy leaf from a mock-inoculated millet plant. (B–F) Viral symptoms on millet leaves infected with FoMV (B), FoMV/AtFT (C), FoMV/Hd3a (D), FoMV/SFT (E), or FoMV/NtFT4 (F). (G) RT-PCR detection of virally expressed FT mRNA (upper panel). The size of the 2000-bp DNA marker as well as the positions of FT mRNA and FoMV RNA are indicated. The proso millet 18S rRNA was used as internal RT-PCR control (bottom panel). (H–M) Early flowering and millet spikelet development. Millet plants remained vegetative in mock (H)- or FoMV (I)-inoculated controls. Effective FT expression (G) induced early flowering and development of millet spikelet in plants infected with FoMV/AtFT (J), FoMV/Hd3a (K), FoMV/SFT (L), or FoMV/NtFT4 (M). Photographs were taken at 28 DASS (A–F), or 77 DASS (H–M). The boxed section of each plant was enlarged to show clear phenotypes on the top of panels of (H–M). Scale bar: 3 cm.
Differential activities of monocot and dicot FT genes in flowering induction in proso millet
Rice Hd3a, and the dicot FT genes to a lesser extent, when expressed from the FoMViF vectors promoted early floral and spikelet development in proso millet (Fig. 3), suggesting that the monocot and dicot FT genes had different capabilities to accelerate or induce flowering in our FoMViF assays (Table 1; Supplementary Table S3). We observed visible FoMV/FT infection (Fig. 3C–F) and detected efficient accumulation of virally expressed FT mRNAs in all tested plants (Fig. 3G; Supplementary Fig. S4). Interestingly, AtFT and SFT showed some effect on flowering induction and spikelet formation, whilst NtFT4 had very little impact on flowering and spikelet-forming time (Table 1; Supplementary Table S3). In contrast, expression of the rice Hd3a gene from FoMV/Hd3a induced floral and spikelet development markedly earlier than the three dicot counterparts and controls (Table 1; Supplementary Table S3). Considering the equivalent expression levels of viral FT transcripts (Fig. 3G) but varied flowering time (Fig. 3J–M; Table 1; Supplementary Table S3) in proso millets treated with different FoMViF vectors, we concluded that the monocot rice Hd3a gene is the most efficient at inducing flowering in proso millet among the four tested monocot and dicot FT genes.
Expression of FLAG-tagged FT proteins affects flowering time in proso millet
To further investigate the correlation of FT gene expression with the induction of early flowering in proso millets under LD conditions, we used 3×FLAG to tag Hd3a, AtFT, SFT, and NtFT4 (Figs 1B, 4). In plants that were infected with FoMV/AtFT-FLAG, FoMV/Hd3a-FLAG, FoMV/SFT-FLAG, or FoMV/NtFT4-FLAG, we were able to detect both the FoMV-FT-FLAG mRNA by RT-PCR (Fig. 4A) and the FLAG-tagged FT proteins probed with a specific anti-FLAG antibody (Fig. 4B, C). Expression of both FT transcripts and protein products was clearly linked with the viral infection of these plants (Supplementary Fig. S5). No virally expressed FT RNA or FT protein was detected in mock-inoculated proso millets, although FoMV RNA but not FT protein was readily detectable in FoMV-infected plants (Fig. 4A–C). Expression of the FLAG-tagged monocot (Hd3a-FLAG) and dicot FT proteins (AtFT-FLAG, SFT-FLAG, and NtFT4-FLAG) was able to induce early flowering when compared with mock-inoculated or FoMV-infected control plants (Fig. 5A–F; Table 2; Supplementary Table S4). We also noticed a negative impact of the FLAG tag on the florigenic activity of the free rice Hd3a protein. However, the influence of the FLAG tag on the functionality of dicot FT proteins was less obvious (Tables 1, 2). Consequently, the four FT-FLAG fusion proteins enabled plants to flower 4–11 d earlier although they did not show much difference among each other to shorten flowering time in proso millets under LD conditions (Fig. 5; Table 2; Supplementary Table S4).
. | Expt I . | . | Expt II . | . |
---|---|---|---|---|
. | Flowering time (DASS) . | P-valuea . | Flowering time (DASS) . | P-valuea . |
Mock | 80.0±0.0 | 0.006 | 80.0±2.3 | 0.08 |
FoMV | 77.5±1.7 | n.a. | 77.0±0.0 | n.a. |
FoMV/AtFT-FLAG | 69.5±3.7 | 0.008 | 73.6±1.1 | 0.001 |
FoMV/Hd3a-FLAG | 66.6±2.1 | 6.67×10−5 | 73.4±0.9 | 9.62×10−5 |
FoMV/SFT-FLAG | 67.8±3.6 | 0.002 | 73.2±1.7 | 0.012 |
FoMV/NtFT4-FLAG | 70.7±3.5 | 0.018 | 72.6±1.8 | 0.005 |
. | Expt I . | . | Expt II . | . |
---|---|---|---|---|
. | Flowering time (DASS) . | P-valuea . | Flowering time (DASS) . | P-valuea . |
Mock | 80.0±0.0 | 0.006 | 80.0±2.3 | 0.08 |
FoMV | 77.5±1.7 | n.a. | 77.0±0.0 | n.a. |
FoMV/AtFT-FLAG | 69.5±3.7 | 0.008 | 73.6±1.1 | 0.001 |
FoMV/Hd3a-FLAG | 66.6±2.1 | 6.67×10−5 | 73.4±0.9 | 9.62×10−5 |
FoMV/SFT-FLAG | 67.8±3.6 | 0.002 | 73.2±1.7 | 0.012 |
FoMV/NtFT4-FLAG | 70.7±3.5 | 0.018 | 72.6±1.8 | 0.005 |
Raw data are available in Supplementary Table S4. Flowering time is shown in days after sowing seeds (DASS; mean ±SD) in each experiment. n.a., not applicable.
aP values generated with equal variances with two-tailed Student’s t-test against FoMV are shown. P≤0.05 is regarded as a statistically significant difference.
. | Expt I . | . | Expt II . | . |
---|---|---|---|---|
. | Flowering time (DASS) . | P-valuea . | Flowering time (DASS) . | P-valuea . |
Mock | 80.0±0.0 | 0.006 | 80.0±2.3 | 0.08 |
FoMV | 77.5±1.7 | n.a. | 77.0±0.0 | n.a. |
FoMV/AtFT-FLAG | 69.5±3.7 | 0.008 | 73.6±1.1 | 0.001 |
FoMV/Hd3a-FLAG | 66.6±2.1 | 6.67×10−5 | 73.4±0.9 | 9.62×10−5 |
FoMV/SFT-FLAG | 67.8±3.6 | 0.002 | 73.2±1.7 | 0.012 |
FoMV/NtFT4-FLAG | 70.7±3.5 | 0.018 | 72.6±1.8 | 0.005 |
. | Expt I . | . | Expt II . | . |
---|---|---|---|---|
. | Flowering time (DASS) . | P-valuea . | Flowering time (DASS) . | P-valuea . |
Mock | 80.0±0.0 | 0.006 | 80.0±2.3 | 0.08 |
FoMV | 77.5±1.7 | n.a. | 77.0±0.0 | n.a. |
FoMV/AtFT-FLAG | 69.5±3.7 | 0.008 | 73.6±1.1 | 0.001 |
FoMV/Hd3a-FLAG | 66.6±2.1 | 6.67×10−5 | 73.4±0.9 | 9.62×10−5 |
FoMV/SFT-FLAG | 67.8±3.6 | 0.002 | 73.2±1.7 | 0.012 |
FoMV/NtFT4-FLAG | 70.7±3.5 | 0.018 | 72.6±1.8 | 0.005 |
Raw data are available in Supplementary Table S4. Flowering time is shown in days after sowing seeds (DASS; mean ±SD) in each experiment. n.a., not applicable.
aP values generated with equal variances with two-tailed Student’s t-test against FoMV are shown. P≤0.05 is regarded as a statistically significant difference.
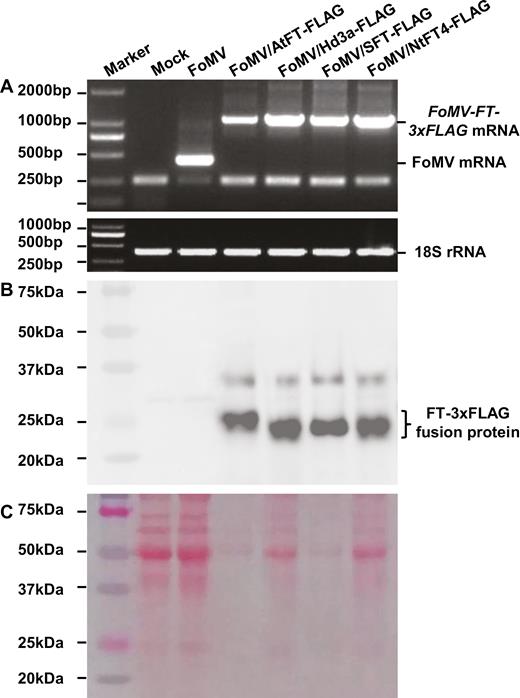
Analysis of FT-FLAG expression in proso millet. (A) RT-PCR assay of FT-FLAG mRNA (upper panel). Proso millet 18S rRNA served as internal control (bottom panel). (B, C) Detection of FT-FLAG proteins using a specific anti-FLAG antibody. Western blot (B) and a protein PAGE gel (C) are shown. Total RNAs or proteins were isolated from plants mock-inoculated or infected with FoMV, FoMV/AtFT-FLAG, FoMV/Hd3a-FLAG, FoMV/SFT-FLAG, or FoMV/NtFT4-FLAG. Sizes and positions of the 2000-bp DNA ladder or the pre-stained protein marker, positions of viral FT-FLAG mRNA, FoMV RNA, 18S rRNA, as well as the FT-FLAG fusion proteins are indicated.
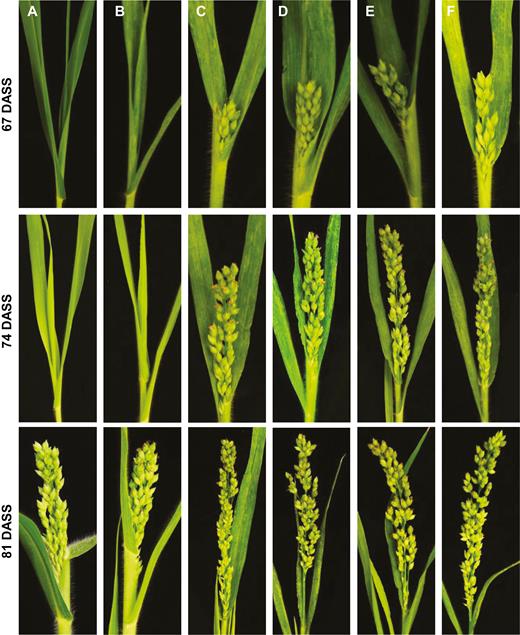
Foral and spikelet induction by virally expressed FLAG-tagged FT proteins in proso millet. Proso millet plants were mock-inoculated (A), or infected with FoMV (B), FoMV/AtFT-FLAG (C), FoMV/Hd3a-FLAG (D), FoMV/SFT-FLAG (E), or FoMV/NtFT4-FLAG (F). Flowering and spikelet development under LD conditions was photographed at 67, 74, and 81 DASS.
FoMViF in wheat
To test the feasibility of the FoMViF assay in other monocots, we mechanically inoculated young wheat plants at two- to three-leaf stage (approximately 21 DASS) with sap in 10 mM Tris–1 mM EDTA buffer (pH 8.0) produced from healthy N. benthamiana (mock) or plants infected with FoMV/Hd3a (Figs 1A, 6). Mock-inoculated controls and virus-infected wheat plants were then grown at 23 °C under LD conditions without vernalization (i.e. non-flowering inductive conditions). As we previously reported, FoMV could effectively infect wheat (Liu et al., 2016). Local chlorosis and yellowing developed on sap-inoculated leaves at approximately 1 week post-inoculation (i.e. 28 DASS; Fig. 6A, B), and systemic infection occurred subsequently. No flowering/heading and no grain development were observed in mock-inoculated wheat plants at 8 weeks post-inoculation (i.e. 84 DASS; Fig. 6C), at which time (approximately 77 DASS on average) the first heading was clearly appearing on wheat plants that were infected with FoMV/Hd3a (Fig. 6D; Supplementary Table S5). At 119 DASS, control plants remained vegetative and underwent no reproductive transition (Fig. 6E) whilst wheat plants with virally expressed Hd3a developed the second, third and fourth heads at this stage (Fig. 6F, G). Indeed, even after the wheat grains fully matured in plants in which Hd3a was expressed from FoMV/Hd3a, no obvious heading/flowering was noticeable in all control plants, although these plants (including mock-inoculated and FoMV- or FoMV/Hd3a-FLAG infected wheats) started to develop heading at very late stage (Fig. 6G; Supplementary Table S5). Moreover, the induction of flowering and development of spikelet were well-correlated with the expression of the Hd3a transcripts by FoMV/Hd3a in virus-infected wheat, even if the level of the Hd3a mRNA was relatively low (Fig. 6H; Supplementary Table S2). The FLAG-tagged Hd3a fusion protein was readily detected in wheat plants infected with FoMV/Hd3a-FLAG, but not FoMV or mock treatment (Fig. 6I; Supplementary Fig. S6). These results show that FoMViF is functional in the economically important cereal crop wheat.
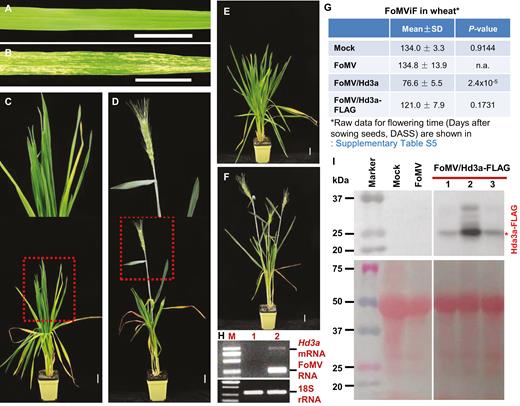
Expression of rice Hd3a triggers early flowering and grain production in wheat. (A, B) Development of viral infection in wheat. Wheat with mock inoculation was symptomless (A). Infection by FoMV/Hd3a led to development of yellowing and chlorosis in wheat leaves (B). (C–F) FoMViF in wheat. Wheat plants mock-inoculated remained vegetative and showed no sign of reproductive growth (C, E). Plants with FoMV/Hd3a infection produced early heading/flowering at 11 weeks after sowing seeds (D), and more heads were developed later on (F). Plants were photographed at 28 DASS (A, B), 84 DASS (C, D) or 119 DASS (E, F). The boxed section of plants in (C, D) was enlarged to show clear phenotypes at the top of each panel. Scale bar: 3 cm. (G) Summary of FoMViF in wheat. Flowering time (DASS) is shown as mean ±SD. Raw data for the flowering time are shown in Supplementary Table S5. P-values generated with equal variances with two-tailed Student’s t-test against FoMV are shown. P≤0.05 is regarded as a statistically significant difference. n.a., not applicable. (H) RT-PCR detection of virally expressed Hd3a transcript in wheat. Total RNA samples were isolated from plants with mock inoculation (lane 1) or plants infected with FoMV/Hd3a (lane 2). The positions of Hd3a mRNA and FoMV RNA as well as the 2000-bp DNA marker are indicated (upper panel). Wheat 18S rRNA served as internal control (bottom panel). (I) Detection of Hd3a-FLAG proteins using a specific anti-FLAG antibody. Western blot (upper panel) and a protein PAGE gel (bottom panel) are shown (Supplementary Fig. S6). Total proteins were isolated from wheat plants mock-inoculated or infected with FoMV or FoMV/Hd3a-FLAG. Three individual plants (nos 1, 2, and 3) were used in cases of FoMV/Hd3a-FLAG infection. Sizes and positions of the pre-stained protein marker as well as positions of the Hd3a-FLAG fusion proteins are indicated.
Discussion
Plant viruses have either an RNA or a DNA genome. These viral genomes can be modified as RNA delivery vehicles and gene expression vectors for functional genomics in plants. For instance, VIGS has emerged as a powerful tool to inhibit gene expression at the transcriptional, post-transcriptional, or translational level for dissecting gene function in dicots and monocots, including important crops recalcitrant to classical forward or reverse genetic manipulation (Liu et al., 2002; Becker and Lange, 2010; Tang et al., 2010; Kanazawa et al., 2011; Senthil-Kumar and Mysore, 2011; Sha et al., 2014; Chen et al., 2015c; Qin et al., 2015). Using viruses to deliver small guide RNA can lead to virus-induced genome editing in plants (Baltes et al., 2014; Yin et al., 2015). Virus-based technology has also been used to study RNA signaling that is associated with different plant physiological processes such as flowering and tuberization (Li et al., 2009, 2011; Cho et al., 2015). On the other hand, transient overexpression of exogenous or endogenous genes from engineered viruses can result in gain-of-function or virus-induced gene complementation in plant development and growth, fruit ripening, plant response to biotic stresses, and viral DNA replication (Hong et al., 1997, 2003; van Wezel et al., 2002; Lin et al., 2008; Zhou et al., 2012; Kong et al., 2013; Bouton et al., 2018). Moreover, VIF is the latest example of how plant viruses can be exploited for the benefit of fundamental research in the field of flower physiology and practical applications in facilitation of plant breeding (McGarry et al., 2017; Qin et al., 2017). It should be noted that VIGS is completely different from VIF. VIGS is a means to knock-down target gene expression via homologous RNA-mediated degradation of target mRNA, whilst VIF such as FoMViF involves using a virus vector to transiently overexpress flowering time gene to induce early flowering in plants. Thus, the two plant virus-based technologies differ in principle.
Since the early VIF assay was reported in Cucurbita (Lin et al., 2007) and tobacco (Li et al., 2009), several VIF systems have been developed in dicots including cotton and fruit trees (Yamagishi and Yoshikawa, 2011; Yamagishi et al., 2011, 2014, 2016; McGarry and Ayre, 2012; Fekih et al., 2016; McGarry et al., 2016, 2017; Velázquez et al., 2016; Qin et al., 2017). This work reveals the mechanism of how VIF operates and the potential of VIF for the study of plant reproductive biology and in crop breeding (McGarry et al., 2017; Qin et al., 2017). However, no VIF system has been yet developed for any of the cereal crops such as wheat, rice, maize, and millets that are essential for human nutrition, global food security, agriculture, and biofuel industry. Lack of VIF in monocots may be due to a shortage of suitable viruses for development of such technology. Unlike VIGS vectors, which only involve delivery of a short fragment of translatable or non-translatable RNA homologous to the target genes to plant cells, virus vectors for VIF or production of functional proteins need to have the capacity to express the entire mRNA from which protein products can be translated. Only a few monocot-infecting viruses such as BSMV, Wheat streak mosaic virus, Triticum mosaic virus, and FoMV have been shown to have this capacity (Tatineni et al., 2015; Bouton et al., 2018; Cheuk and Houde, 2018). Here we demonstrate that FoMV can be exploited to express FT genes and induce early flowering and spikelet development in the grass proso millet (Figs 1–5; Tables 1, 2; Supplementary Tables S3, S4) and the cereal crop wheat (Fig. 6; Supplementary Table S5).
Stability is essential for using recombinant viruses as a toolbox for gene expression in plants. Recombinant viruses are known to be unstable and commonly undergo rearrangements to their native form during infection. Moreover, those with longer infection times likely undergo rearrangements to their native forms during infection. These are potential issues for the use of recombinant virus technology in plants. Indeed, in our FoMViF assays loss of inserted FT genes from recombinant FoMV genome occurred during viral infection of proso millet and wheat plants (Figs 3G, 4A, 6H; Supplementary Table S2). On the other hand, florigenic FT proteins, once expressed during the early stage of plant development, would prime and initiate the vegetative-to-reproductive transition and then lead to flowering. This implies that no persistent production of FT mRNA and protein is needed at the later stage of plant growth. In this regard, stability of recombinant viruses as such may not impose a significant challenge or weakness for VIF technology, such as FoMViF described here. This notion is supported by the finding that even if a population of FoMViF vectors converted back to FoMV in proso millet and wheat (Supplementary Table S2), these plants with virally expressed FT proteins still flowered early (Figs 3, 4, 6). Indeed, loss of recombinant sequences, and reversion to a naturally occurring structure can be argued as advantageous for containment.
Another concern associated with VIF and gene expression from recombinant viruses in general is the potential VIGS effect. Since FoMV is a ssRNA virus, it has a dsRNA intermediate, and these are active triggers for VIGS in plants. As such, many ssRNA viruses are commonly used for VIGS and less commonly for gene delivery and protein expression. However, this does not seem to be an issue for FoMViF. Firstly, FT proteins, and the phosphatidylethanolamine-binding protein domains particularly, are conserved among different plant species (Turck et al., 2008; Supplementary Fig. S2). At the nucleotide level, the overall homologies of FT genes and mRNAs are also notably high. However, these mRNA sequences are not identical; instead nucleotide polymorphisms are common. No 20 nucleotides or longer are identical among the FT mRNAs (Supplementary Fig. S1; unpublished data). Evidently, there is insufficient homology between the millet PmFT and the rice or dicot FTs to result in silencing. Secondly, our FoMViF assays were carried out under non-flowering inductive conditions. This implies that little or no endogenous FT mRNA would be produced and thus there would be little or no target mRNA for VIGS to occur. Thirdly, viral expression of florigenic FTs could occur during the early and/or later infection stage, thus triggering plants to flower no matter whether VIGS would be induced later or not. This is evident as viral expression of FT genes, even native PmFT (unpublished data), can result in early flowering in proso millet.
Apart from these potential disadvantages, the FoMViF has several advantages. (i) The monopartite genome makes FoMV much easier to handle. Moreover, the enriched viral inoculum in N. benthamiana is highly infectious in monocot plants (this work; Liu et al., 2016; Bouton et al., 2018). (ii) The broad spectrum of dicot and monocot host plants allow FoMViF and other FoMV-based technology applicable to many of the most important food and biofuel crops such as wheat, millets, and maize. This is particularly useful for functional genomics in monocot crops including wheat and millets in which genetic manipulation is difficult or no transformation system is yet available. (iii) As shown in our work here and that recently reported by Bouton et al. (2018), FoMV can be utilized to efficiently express small and large proteins for ‘gain-of-function’ analysis in monocots. (iv) The differential activities among monocot and dicot FT genes, as well as the impact of a FLAG tag on FT function revealed in our work, provide choices of florigenic genes for FoMViF-based cereal crop breeding programs dependent on various degrees of requirements for floral and spikelet/grain induction.
Our FoMViF demonstrates that heterologous FT genes from either monocot (rice) or dicot (tomato and Arabidopsis) plants can trigger early flowering and early spikelet development in monocotyledonous proso millet (Fig. 3), and wheat in the case of Hd3a (Fig. 6), consistent with our previous finding that these FT genes were also able to induce early flowering in the PVX-based VIF system in dicot tobacco plants (Qin et al., 2017). Interestingly, the rice Hd3a was equally as efficient as SFT, AtFT, and NtFT4 in inducing flowering in dicot tobacco plants under non-inductive conditions (Qin et al., 2017). By contrast, the dicot SFT, AtFT, and NtFT4 seem much less active than Hd3a in monocot proso millet (Fig. 3; Table 1) although their protein products share high amino acid identities (Supplementary Fig. S2). We also found that the FLAG tagging could affect the function of FT proteins (Figs 5, 6). These results further support that the photoperiodic FT-mediated pathway in floral induction is conserved among different plants (Turck et al., 2008), but also imply that this pathway may have undergone evolutionary divergence in dicot and monocot species. Moreover, the FoMViF works in C3 wheat (Fig. 6), reaffirming the idea that the technology can be applied to both C3 and C4 crops.
Conclusions
VIF has recently attracted extensive interest for its practical applications in accelerating breeding in dicotyledonous plants and woody fruit-trees. We now extend this technology to a monocot grass and a cereal crop. Using the FoMV-based VIF system, we showed that expression of FT orthologues can promote early flowering and development of spikelet in proso millet, a C4 grass species with potential as a nutritional food and biofuel resource, and in wheat, a main food crop worldwide. Floral induction in the two monocot plants is caused by the virally expressed FT genes, and the florigenic activity of rice Hd3a was more pronounced than its dicotyledonous counterparts in proso millet. This system is easy to perform and the efficacy to induce flowering is high. In addition to proso millet and wheat, we envisage that FoMViF will be also applicable to many important food and biofuel monocots in terms of functional genomics as well as molecular breeding for cereal improvement.
Supplementary data
Supplementary data are available at JXB online.
Fig. S1. Comparisons of tomato SFT, tobacco NtFT4, rice Hd3a, wheat TaFT1, and Arabidopsis AtFT nucleotide sequences.
Fig. S2. Comparisons of Arabidopsis AtFT, tobacco NtFT4, tomato SFT, rice Hd3a, and wheat TaFT1 protein sequences.
Fig. S3. Vernalization rescinds impact on FoMViF to induce early heading/flowering in wheat.
Fig. S4. Sequencing confirmation of heterologous FT gene expression in proso millet.
Fig. S5. Systemic symptoms of FoMV infection in proso millet.
Fig. S6. Original blot and PAGE gel used for Fig. 6I.
Table S1. Primers used in this work.
Table S2. Stability of recombinant FoMViF vectors in plants.
Table S3. Data for FoMViF in proso millet.
Table S4. Data for FLAG-tagged FoMViF in proso millet.
Table S5. Data for FoMViF in wheat.
Abbreviations
- BSMV
Barley stripe mosaic virus
- CP
coat protein
- FoMV
Foxtail mosaic virus
- FoMViF
FoMV-induced flowering
- FT
Flowering Locus T
- LD
long-day
- PVX
Potato virus X
- SD
short-day
- VIF
virus-induced flowering
- VIGS
virus-induced gene silencing
Acknowledgements
We are grateful to X. Kong for her kind gift of the winter-wheat seeds. We thank two anonymous reviewers for their constructive comments to improve this manuscript. This work was supported by grants from the Ministry of Science and Technology of China (National Key R&D Program of China 2017YFE0110900); the Ministry of Agriculture of China (National Transgenic Program 2016ZX08009-001-004); the National Natural Science Foundation of China (NSFC 31872636), and the Hangzhou Normal University (Sino-EU Plant RNA Signaling S&T Platform Initiative 9995C5021841101). CY, HL and CQ designed and performed experiments; XZ, QC, PZ, XX and MH performed research; CY, HL, CQ, X-lZ, MT, DX, HW, SJ, Y-hH, YL and NS, were involved in the analysis of data and helped writing the paper. Y.H. conceived and initiated the project, designed experiments, analyzed data and wrote the paper. The authors declare no conflicts of interest.
References
Author notes
These authors contributed equally to this work.
Comments