-
PDF
- Split View
-
Views
-
Cite
Cite
Ainhoa Martínez-Medina, Leyre Pescador, Laura C Terrón-Camero, María J Pozo, María C Romero-Puertas, Nitric oxide in plant–fungal interactions, Journal of Experimental Botany, Volume 70, Issue 17, 1 September 2019, Pages 4489–4503, https://doi.org/10.1093/jxb/erz289
- Share Icon Share
Abstract
Whilst many interactions with fungi are detrimental for plants, others are beneficial and result in improved growth and stress tolerance. Thus, plants have evolved sophisticated mechanisms to restrict pathogenic interactions while promoting mutualistic relationships. Numerous studies have demonstrated the importance of nitric oxide (NO) in the regulation of plant defence against fungal pathogens. NO triggers a reprograming of defence-related gene expression, the production of secondary metabolites with antimicrobial properties, and the hypersensitive response. More recent studies have shown a regulatory role of NO during the establishment of plant–fungal mutualistic associations from the early stages of the interaction. Indeed, NO has been recently shown to be produced by the plant after the recognition of root fungal symbionts, and to be required for the optimal control of mycorrhizal symbiosis. Although studies dealing with the function of NO in plant–fungal mutualistic associations are still scarce, experimental data indicate that different regulation patterns and functions for NO exist between plant interactions with pathogenic and mutualistic fungi. Here, we review recent progress in determining the functions of NO in plant–fungal interactions, and try to identify common and differential patterns related to pathogenic and mutualistic associations, and their impacts on plant health.
Introduction
Fungi play a major role in natural and agricultural ecosystems. They are important decomposers and recyclers of organic materials and they can interact with plant roots in the rhizosphere or with above-ground tissues (Zeilinger et al., 2016). The interactions between plants and their associated fungi are complex and the outcomes are diverse, ranging from parasitism to mutualism. Fungal plant pathogens are of huge economic importance because they threaten crop production, not only when plants are growing in the field but also in the form of post-harvest diseases. Most of the major economically relevant plant pathogens are fungi such as Botrytis cinerea, and species of Fusarium, Rhizoctonia, and Magnaporthe (Dean et al., 2012). On the other hand, mutualistic associations between fungi and plants are common in nature and can improve the productivity of crop plants. For instance, it is estimated that about 90% of plants form mycorrhizal symbioses, in which photosynthates from the host are exchanged for mineral resources acquired by the fungus from the soil (Ferlian et al., 2018). To cope with pathogenic fungi, plants are able to activate defence mechanisms, and are generally at least partially resistant to most fungal pathogens. Hence, mutualistic and neutral associations are most prevalent and parasitic associations are considered to be the exception (Staskawicz, 2001).
The interactions of plants with fungi are characterized by a series of sequential events beginning with the initial contact with the host plant, and including the fungal attachment to the host structures, the entry and colonization of the plant tissues, and the fungal reproduction (Lo Presti et al., 2015). Depending on the nature of the interaction (pathogenic, neutral, or mutualistic) and the life cycle of the fungus (necrotrophic or biotrophic), plants may respond to fungal colonization with an immune response in which several plant signalling compounds play pivotal roles, including intracellular calcium (Ca2+) and other ions, reactive oxygen and nitrogen species (ROS/RNS), phytohormones, and small RNAs (Mur et al., 2006; Pieterse et al., 2012; Weiberg et al., 2014; Pozo et al., 2015; Waszczak et al., 2018). It is notable that the signalling networks and key regulatory elements that are involved in the plant responses to pathogenic and mutualistic fungi overlap (Pozo et al., 2015). This indicates that the regulation of the adaptive response of the plant is finely balanced between protection against aggressors and acquisition of benefits from mutualistic associations (Pieterse et al., 2014). Achieving this balance requires the perception of potential invading fungi, followed by rapid and tight regulation of immune responses to promote or contain the fungal colonization of plant tissues (Zamioudis and Pieterse, 2012; Zipfel and Oldroyd, 2017; Plett and Martin, 2018).
Nitric oxide (NO) is a diffusible, reactive free-radical gaseous molecule that is involved in the regulation of a wide range of plant developmental processes, such as seed germination (Gibbs et al., 2014; Albertos et al., 2015; Del Castello et al., 2019), root development (Sanz et al., 2015; Castillo et al., 2018), flowering (He et al., 2004; Prado et al., 2004; Serrano et al., 2012), and fruit development (Manjunatha et al., 2012; Du et al., 2014). NO also regulates plant responses to several abiotic stresses such as hypoxia, salinity, and heavy metals (Gupta et al., 2016; Romero-Puertas et al., 2018), and it is involved in defence responses against microbial pathogens, including bacteria and fungi (Trapet et al., 2015). Indeed, during plant immune responses against fungal pathogens, NO triggers a global reprograming of gene expression, the production of secondary metabolites with antimicrobial properties, and the hypersensitive response (Mur et al., 2017). There is a growing body of evidence that indicates that NO is also produced during the establishment of mutualistic interactions between plants and fungi (Calcagno et al., 2012; Espinosa et al., 2014; Gupta et al., 2014; Martínez-Medina et al., 2019a). Although the specific role(s) of NO in plant–fungal mutualisms remains unclear, recent evidence suggests that tight control of NO levels is required for the control of the mycorrhizal symbiosis (Martínez-Medina et al., 2019a).
The diverse roles of NO during detrimental and mutualistic plant–fungal interactions might seem contradictory but can be explained by its versatile properties. As a signalling molecule, NO function depends on the rate and location of its production, and its concentration is critical: it acts as a signal at low concentrations but displays toxic effect when present at high concentrations (Hancock and Neill, 2019). Moreover, the highly reactive nature of NO facilitates its different regulatory roles as it reacts directly with other free radicals, metals, and proteins, leading to post-translational modifications that regulate protein activity and stability, and gene expression (Abello et al., 2009; Martínez-Ruiz et al., 2013; Lamotte et al., 2014; Yu et al., 2014; Romero-Puertas and Sandalio, 2016).
Here, we review and synthesize recent and relevant information dealing with the role(s) of NO in the interaction of plants with pathogenic and beneficial fungi, highlighting recent advances and identifying the major gaps in our knowledge. We acknowledge that both the plant and the fungal partners are potential sources and regulators of NO during interactions; however, several excellent reviews have recently been published on fungal NO (Arasimowicz-Jelonek and Floryszak-Wieczorek, 2016; Cánovas et al., 2016), so here we focus on the NO produced by plants during their interactions with diverse fungi.
Roles and metabolism of NO in plant immunity
Plants can be considered as unexpectedly healthy given the enormous number of potential pathogens in their environments (Dangl, 2013), and this is mainly due to the plant immune system. After the recognition of potential aggressors through the perception of pathogen-associated molecular patterns (the so called PAMPs; MAMPs when it is associated with non-pathogenic microbes) or from signals related to self-damage (damage-associated molecular patterns, DAMPs), the plant activates a defence response termed the basal or PAMP-triggered immunity (PTI). Some pathogens are able to avoid PTI by evading recognition or by the blocking defense response through small molecules called effectors, which promote infection (Couto and Zipfel, 2016). Plants can, however, possess a second layer of perception involving intracellular receptors with nucleotide binding-site leucine-rich repeats (NBS-LRRs, also termed NLRs) through which they are able to recognize microbe effectors, thus inducing effector-triggered immunity (ETI; Couto and Zipfel, 2016). Although both PTI and ETI activate similar mechanisms, ETI is stronger and faster, and leads to programmed cell death of the invaded area in order to restrain dispersion of the pathogen, a process known as the hypersensitive response (HR; Dodds and Rathjen, 2010).
One of the first biological functions assigned for NO in plants was related to plant immunity (Yu et al., 2014). The occurrence of a peak of NO has been observed during both PTI and ETI responses. However, most studies have dealt with the role of NO in ETI and HR, and less attention has been paid to NO production and function during PTI. Different MAMPs or DAMPs such as cryptogein, lipopolysaccharides, and oligogalacturonides have also been shown to induce NO production (Trapet et al., 2015), and show a feedback interaction with Ca2+ (Courtois et al., 2008). In this context, NO is able to regulate a wide variety of different plant immune responses (Yu et al., 2012; Bellin et al., 2013). Indeed, it is well known that NO produced after microbe recognition triggers a global reprograming of gene expression, the production of secondary metabolites with antimicrobial properties, and ultimately, the HR and systemic acquired resistance (Bellin et al., 2013; Wendehenne et al., 2014). NO and related RNS perform their bioactivity mainly via chemical reactions with specific target proteins, leading to NO-dependent post-translational modification (PTMs), namely S-nitrosylation, nitration, or nitrosylation. Comprehensive reviews on this topic have been published by Scheler et al. (2013) and Yu et al. (2014). The levels of nitrosothiols are very important in the evolution of plant defence responses, as mutants with altered S-nitrosoglutathione reductase levels show impaired pathogen resistance (Feechan et al., 2005; Rustérucci et al., 2007). Furthermore, proteomic analyses of plants undergoing HR show that there are changes in S-nitrosylated proteins related to intermediary metabolism, hormone-dependent signalling, and ROS-producing enzymes, and in proteins related to antioxidant defences and programmed cell death (Feechan et al., 2005; Romero-Puertas et al., 2007, 2008). Different transcription factors have also been shown to be targets of S-nitrosylation. This fact could explain how NO can coordinate gene expression changes. For example, in Arabidopsis NO has been proposed to switch the translocation of NPR1, a transcriptional co-activator involved in the induction of pathogenesis related genes (PR), into the nucleus, and to regulate the specific DNA-binding of its transcription-factor interactor, TGA1 (Tada et al., 2008; Lindermayr et al., 2010). Recently, it has been shown that the zinc finger transcription factor SRG1, which functions as a positive regulator of plant immunity, is a central target of NO bioactivity. When SRG1 is S-nitrosylated (represented as SRG1-SNO) it contributes to a negative feedback loop that decreases the plant immune responses (Cui et al., 2018). Proteomic analyses during plant defence responses have also shown protein targets of nitration that are involved in different cellular processes such as photosynthesis, glycolysis, and nitrate assimilation (Cecconi et al., 2009). Analysis in tobacco has suggested that tyrosine nitration may regulate MAPKK signalling and therefore phosphorylation cascades during the defence response (Vandelle and Delledonne, 2011).
Despite an increasing body of literature on the roles of NO in plants, there are still many unknowns regarding the sources of NO as well as the proteins/molecules that regulate NO levels in the cell. Several mechanisms have been reported with respect to NO production in plants. The best-characterized enzymatic route of NO production is the nitrate reductase (NR) pathway, in which nitrate is reduced to nitrite. The oxidative pathway and nitric oxide synthase (NOS)-like activity have also been shown to be involved in NO production during plant defence. Readers are referred to several excellent reviews for additional information on this topic (Baudouin and Hancock, 2013; Mur et al., 2013; Yu et al., 2014; Jeandroz et al., 2016; Astier et al., 2018). Our knowledge of NO catabolism is also very incomplete. NO can quickly react with glutathione (GSH) to form S-nitrosoglutathione (GSNO), with O2 and O2.− to form nitrogen dioxide (NO2) and peroxynitrite (ONOO–), which is involved in NO-dependent PTMs as described above (Neill et al., 2008). Phytoglobins (previously known as non-symbiotic haemoglobins), which are able to modulate NO levels through their NO dioxygenase activity, have also been shown to be involved in NO modulation in plant immunity (Hebelstrup et al., 2014). Overall, the complex regulation of NO has slowed down the identification of downstream NO-regulated processes because it makes the generation of null NO-producing mutants difficult (Bruand and Meilhoc, 2019). However, thanks to the use of NO donors and scavengers, and mutants impaired in NO metabolism, the regulatory role of NO in numerous plant processes including plant immunity is now well established.
Although our knowledge of the molecular mechanisms mediating the role of NO in plant immunity has increased considerably over recent decades, most of the studies have been performed on model plants (mostly Arabidopsis) interacting with bacteria. Despite the importance of both beneficial and pathogenic fungi on plant health, the roles of NO in plant–fungal interactions have been far less well explored. In the following sections we attempt to compile and summarize the available information on these interactions, and to highlight common and differential patterns and functions during interactions with beneficial and pathogenic fungi.
NO in plant–fungal pathogenic interactions
Pathogenic fungi can use diverse strategies to colonize plants and cause disease. Necrotrophic fungal pathogens, which often show a broad host range, kill their hosts and take up nutrients released from the dead tissues. Several compounds including cell wall-degrading enzymes, ROS, and/or toxins have been implicated in the degradation of host cells by necrotrophic fungi (Wolpert et al., 2002). In contrast, biotrophic fungal pathogens, which show host specificity, do not produce toxins but often secrete effectors to suppress the host immune system (Perfect and Green, 2001). Hemibiotrophic fungal pathogens are intermediate between the necrotrophic and the biotrophic life styles, initially growing as biotrophs and later switching to being necrotrophic (Koeck et al., 2011). In agreement with the essential role of NO in plant immunity (see above), several studies have indicated that NO is an early component of the defence response triggered by plants to combat fungal infections (Table 1, and references therein). However, the specific role(s) of NO during the interaction of plants with pathogenic fungi seems to be influenced by the necrotrophic/biotrophic character of the pathogen, which dictates the concentration and the spatio-temporal patterns of NO accumulation in the plant tissues. Strikingly, in plant–fungal pathogenic interactions, the fungi may also participate in the production and metabolism of NO (Arasimowicz-Jelonek and Floryszak-Wieczorek, 2016; Cánovas et al., 2016). Several studies have indicated that NO plays an important role in fungal development (Wang and Higgins, 2005; Prats et al., 2008; Baidya et al., 2011). Moreover, fungal pathogens may use NO to their own benefit to accelerate the spread of infection, especially in interactions involving necrotrophic and hemi-biotrophic pathogens (van Baarlen et al., 2004; Sarkar et al., 2014; Arasimowicz-Jelonek and Floryszak-Wieczorek, 2016). Indeed, NO has been found to be produced by several necrotrophic pathogens, including B. cinerea, Aspergillus nidulans, Macrophomina phaseolina, Fusarium oxysporum, and Colletotrichum coccodes (Conrath et al., 2004; Wang and Higgins, 2005; Floryszak-Wieczorek et al., 2007; Turrion-Gomez and Benito, 2011; Sarkar et al., 2014). Thus, fungus-produced NO can also be considered as a virulence factor that determines the success of the aggressor. As noted in the Introduction, excellent recent reviews focused on fungal-produced NO during pathogenesis are available (Arasimowicz-Jelonek and Floryszak-Wieczorek, 2016; Cánovas et al., 2016).
A summary of studies where NO production in plant–fungal interactions have been demonstrated, together with its proposed role
Fungus . | Plant . | Type of interaction* . | NO level (technique) . | Time scale . | NO source . | Gene expression . | Pharmacological approach . | Genetic approach . | Suggested function . | Reference . |
---|---|---|---|---|---|---|---|---|---|---|
Blumeria graminis | Hordeum vulgare (leaf) | Path | DAF-2DA | 6–24 h | – | – | cPTIO (0.25 mM), SNP (0.05 mM), L-NAME (1 mM) | – | NO contributes to HR and cell death, leading to infection being halted. NO also contributes to papilla formation. | Prats et al. (2005) |
Botrytis cinerea | Arabidopsis thaliana (leaf) | Path | DAF-2DA | 6 d | – | PR1/LOX2/LOX3/AOS/OPR3/VSP2/GDSL/ERF2 + array | N-isobutyl decanamide (60 μM) | Jar1/Coi1/Eds16/Mpk6 | Alkamides are involved in induction of plant immunity and change NO levels. | Méndez-Bravo et al. (2011) |
B. cinerea | Arabidopsis thaliana (leaf) | Path | DAF-2DA | 30 min–6 h | NR, Arg | – | OG, L-NAME (5 mM), cPTIO (500 µM), Tungstate (µM) | nia1nia2/cngc2/per4-1/per4-2/glu/RBOH-D | NO participates in the regulation of OG-responsive genes (PER4/a b-1,3-glucanase). Plants treated with cPTIO are more susceptible to the fungus. | Rasul et al. (2012) |
B. cinerea (PebC1) | Arabidopsis thaliana (leaf/cells) | Path | Greiss regeant | 3–6 h | – | PR1/BGL-2/PR4/PDF1.2/This2.1 | – | Ein2/Coi1/Npr1/NahG | PebC1 protein promotes resistance to infection by rapid increase of NO. | Zhang et al. (2014) |
B. cinerea | Nicotiana benthamiana (leaf) | Path | DAF-2DA | 2–12 d | NOS, NR | NbPR-1/NbLOX/NbGST/NbCAT1 | DPI (50 μM), L-NAME (5 mM), D-NAME (50 μM), cPTIO (500 μM) | NbNOA1/NbRBOHB VIGS | NO contributes to resistance | Asai and Yoshioka (2009) |
B. cinerea | Pelargonium peltatum (leaf) | Path | DAF-2DA/PGSTAT 30 | 5 min–3 d | – | – | – | – | An early NO burst and a later wave of NO generation enhances resistance. | Floryszak-Wieczorek et al. (2007) |
B. cinerea | Solanum lycopersicum, Nicotiana tabacum, Arabidopsis thaliana (leaf) | Path | DAF-2DA | 1–4 d | – | – | c-PTIO (0.25 mM), L-NAME (5 mM) | – | A NO concentration threshold triggers plant cell death. Below the threshold, NO acts as a signalling molecule to activate diverse plant defence systems. | Turrion-Gomez and Benito (2011) |
B. cinerea | Solanum lycopersicum (leaf) | Path | Quantum cascade laser | 30 min–24 h | NR | – | L-NAME (5 mM), SNP (0.1 mM) | ABA mutant sitiens | ABA can decrease resistance via reduction of NO production. | Sivakumaran et al. (2016) |
B. cinerea | Solanum tuberosum cv. Bintje/Bzura (leaf) | Path | Electrochemical method | 0–24 h | – | PR-1/PR-2/PR-3 | – | – | Infection triggers huge NO over-production. | Floryszak-Wieczorek and Arasimowicz-Jelonek (2016) |
Colletotrichum orbiculare | Nicotiana. benthamiama (leaf) | Path | DAF-2DA | 4–6 d | NR, NOS non-enzymatic | – | Tungstate (100 mM) | NOA1-silenced plants (VIGS) | NO helps to defend the plant. Post-transcriptional control of NOA1 influences NO production and is affected through the MEK2 SIPK/NTF4 cascade. | Asai et al. (2008) |
chitiosan (fungal elicitor) | Pisum. sativum (leaf) | Path | DAF-2DA | 10–20 min | NR, NOS | – | cPTIO (0.2 mM), L-NAME (0.1 mM), Tungstate (0.1 mM) | – | NO production may be responsive to fungal PAMPs. | Srivastava et al. (2009) |
Funneliformis mosseae (AMF) | Trifolium repens (root) | Benef | DAF-FM DA | 5–9 weeks | – | PAL/CHS | – | – | AMF increases NO levels in roots, independently of the mycorrhization time. | Zhang et al. (2013) |
F. mosseae (AMF) | Trifolium repens(root) | Benef | DAF-FM DA | 5–9 weeks | – | PAL/CHS | – | – | AMF increases NO in roots, but not systemically to non-mycorrhizal roots in a split-root system. | Zhu et al. (2015) |
Fusarium oxysporum/ Trichoderma asperelloides | Arabidopsis thaliana (root) | Path, Benef | DAF-2DA | 10–120 min | – | 78 NO-modulated genes | cPTIO (100 µM), L-NAME (2.5 mM) | nia1nia2 | T. asperelloides suppresses NO generation elicited by F. oxysporum. | Gupta et al. (2014) |
F. oxysporum (fusaric acid, FA) | Nicotiana tabacum (cells) | Path | DAF-2, DAF-FM DA | 15–90 min | – | PAL/Hsr203J | cPTIO (100 mM), L-NMMA (100 mM) | – | FA can induce programmed cell death in tobacco suspension cells in a NO-dependent way. | Jiao et al. (2013) |
F. oxysporum | Solanum lycopersicum (root) | Path | DAF-2DA, Haemoglobin assay | 48 h | NR | PRs/PAL/ProtIn/PO/GST/CAM/NR | SNP (100 µM), cPTIO (100 µM), L-NAME (10 µM) | – | Ca-treated plants show higher NO production than control. Disease incidence is reduced in Ca-treated plants, which may be due to the higher NO concentration. | Chakraborty et al. (2017) |
F. oxysporum (fungal elicitor) | Trifolium chinensis (cells) | Path | DAF-2 DA | 0–12 h | NOS | PAL | SNP (10 µM), L-NNA (100 µM), PTIO (100 µM) | – | NO activates fungal elicitor-induced responses involving secondary metabolism. | Wang and Wu (2004) |
Gigaspora margarita (exudates) | Medicago truncatula (root) | Benef (symbiosis) | DAF-2DA | 0–15 min | NR | NR/NiR | cPTIO (1 mM) | Transgenic roots, (DMI1-1, DMI2-2, and DMI3-1) | There is a NO specific signature related to AM interactions and a different NO signature when plants are exposed to a general elicitor such as bacterial lipopolysaccharides extract. | Calcagno et al. (2012) |
Magnaporthe grisea (cell wall) | Oriza sativa (leaf/cells) | Path | Greiss regeant | 30 min; 12 h | NOS | PAL/PR-1/CHI | NO acts as a signal mediating the HR induced by the fungus and it is also necessary for the induction of cell death in combination with H2O2. | Hu et al. (2003) | ||
M. oryzae (Nep1Mo) | Arabidopsis thaliana (leaf) | Path | DAF-2DA | 3 h | – | AtERF1/AtLOX3 | SNP (25 mM), cPTIO (400 µM) | AtALY4 | AtAlY4–H2O2–NO pathway mediates multiple Nep1Mo-triggered responses, including stomatal closure, hypersensitive cell death, and defence-related gene expression. | Teng et al. (2014) |
M. oryzae | Hordeum vulgare, Oriza sativa (leaf) | Path | – | – | – | – | PTIO (250–500 µM) | – | Removal of NO delays fungal germination and reduces disease lesion numbers. | Samalova et al. (2013) |
Macrophomina phaseolina and xylanase | Corchorus capsularis (leaf) | Path | DAF-FM DA | 8 h | – | – | cPTIO (200 mM) | – | Low NO concentration functions as a signalling molecule. High NO concentrations facilitate fungal infection by triggering programmed cell death. M. phaseolina can enhance the infection of plant cells through its own production of NO. | Sarkar et al. (2014) |
Oidium neolycopersici | Solanum lycopersicum cv. Amateur/chmielewskii/hirsutum f. glabratum (leaf) | Path | Oxyhemoglobin method, DAF-FM DA | 0–216 h | NOS | – | cPTIO (0.1 mM), L-NAME (10 mM), AMG (10 mM) | – | NO levels are higher in resistant varieties, leading to plant resistance. | Piterková et al. (2009) |
O. neolycopersici | Solanum lycopersicum/chmielewskii/habrochaites f. glabratum (leaf/disc) | Path | DAF-FM DA | 8–72 h | NOS | – | SNP (0.1 mM), L-NAME (1 mM), PTIO (0.1 mM) | – | In a moderately susceptible genotype the disease rate is diminished if NO production by NOS is reduced. NO activates defences in resistant genotype. With cPTIO, the fungus germinates better on the leaves. | Piterková et al. (2011) |
Puccinia striiformis CY22- 2/CY29-1 | Triticum aestivum cv. Lovrin10 (leaf) | Path | Electron spin resonance | 0–120 h | – | – | SNP (0.5; 2.5 mM) | – | There is a general correlation of NO formation and race-specific resistance. | Guo et al. (2004) |
P. coronata f.sp. avenae | Avena sativa (leaf) | Path | DAF | 12–60 h | – | – | cPTIO (500 µM) | – | Simultaneous generation of NO and H2O2 might be associated with the death of cells adjacent to those infected by the avirulent fungal isolate. | Tada et al. (2004) |
P. triticina | Arabidopsis thaliana, Triticum aestivum (leaf) | Path | DAF-DA | 24 h | – | – | – | atrbohD/atrbohF/atrbohD+F/A. thaliana (natural variation) | Identification of loci controlling non-host disease resistance and changes in NO levels. | Shafiei et al. (2007) |
P. triticina | Triticum aestivum (leaf) | Path | DAF-FM DA | 4–72 h | NR, NOS | – | Na2WO4 (100 μM), c-PTIO (200 μM), L-NAME (100 μM) | – | In this incompatible combination NO acts as an important signalling molecule and mediates HR. | Qiao et al. (2015) |
Trichoderma brevicompactum | Arabidopsis thaliana (leaf) | Path | DAF-DA | 2 h | – | – | Alamethicin (50 µM) | – | rRNA cleavage is suppressed by NO. | Rippa et al. (2007) |
Verticillium dahliae (VD-toxins) | Arabidopsis thaliana (leaf) | Path | DAF-2-DA | 45 min | – | PR-1 | Tungstate (100 µM), cPTIO (100 µM) | Atnoa1 | Cortical microtubule dynamics are mediated by NO-dependent signalling. | Shi et al. (2009) |
V. dahliae (VD-toxins) | Arabidopsis thaliana (leaf) | Path | DAF-2-DA | 60 min | NR | – | Tungstate, cPTIO | nia1nia2 | VD toxin-induced NO accumulation is H2O2 dependent, and H2O2 acts synergistically with NO to modulate the dynamic microtubule cytoskeleton responses to VD toxins. | Yao et al. (2012) |
V. dahliae/Rhizophagus irregularis | Olea europaea (root) | Path, Benef | DAF-2DA | 1–24 h | – | – | PTIO (400 mM) | – | NO may be a key in the establishment of symbiosis and the defence response to pathogens. | Espinosa et al. (2014) |
V. dahliae | Arabidopsis thaliana (leaf) | Path | DAF2-DA | 60 min | – | – | SNP (400 µM) | GhHb1-transgenic Arabidopsis | GhHb1 proteins play a role in the defence responses against pathogenic invasions, possibly by modulating the NO level and the ratio of H2O2/NO in the defence process. | Qu et al. (2006) |
V. dahliae | Arabidopsis thaliana (leaf) | Path | DAF-2-DA | 50–60 min | NR | NIA1 | Tungstate (100 µM), L-NNA (100 µM), cPTIO (100 µM) | Atnoa1/nia1/nia2 | NO is induced in response to VD toxins. | Shi and Li (2008) |
V. dahliae | Helianthus annuus (root) | Path | – | – | – | – | SNP (20 µM), GSNO (50 µM) | – | NO pre-treatments do not reduce wilt symptoms. NO donors appear to promote fungal infection. | Monzón et al. (2015) |
V. longisporum | Arabidopsis thaliana (root/leaf) | Path | DAF-2 | 50–80 min | – | Genes analysis at NO peak | – | – | NO may regulate 732 genes in the roots and 474 genes in the shoot. | Tischner et al. (2010) |
Fungus . | Plant . | Type of interaction* . | NO level (technique) . | Time scale . | NO source . | Gene expression . | Pharmacological approach . | Genetic approach . | Suggested function . | Reference . |
---|---|---|---|---|---|---|---|---|---|---|
Blumeria graminis | Hordeum vulgare (leaf) | Path | DAF-2DA | 6–24 h | – | – | cPTIO (0.25 mM), SNP (0.05 mM), L-NAME (1 mM) | – | NO contributes to HR and cell death, leading to infection being halted. NO also contributes to papilla formation. | Prats et al. (2005) |
Botrytis cinerea | Arabidopsis thaliana (leaf) | Path | DAF-2DA | 6 d | – | PR1/LOX2/LOX3/AOS/OPR3/VSP2/GDSL/ERF2 + array | N-isobutyl decanamide (60 μM) | Jar1/Coi1/Eds16/Mpk6 | Alkamides are involved in induction of plant immunity and change NO levels. | Méndez-Bravo et al. (2011) |
B. cinerea | Arabidopsis thaliana (leaf) | Path | DAF-2DA | 30 min–6 h | NR, Arg | – | OG, L-NAME (5 mM), cPTIO (500 µM), Tungstate (µM) | nia1nia2/cngc2/per4-1/per4-2/glu/RBOH-D | NO participates in the regulation of OG-responsive genes (PER4/a b-1,3-glucanase). Plants treated with cPTIO are more susceptible to the fungus. | Rasul et al. (2012) |
B. cinerea (PebC1) | Arabidopsis thaliana (leaf/cells) | Path | Greiss regeant | 3–6 h | – | PR1/BGL-2/PR4/PDF1.2/This2.1 | – | Ein2/Coi1/Npr1/NahG | PebC1 protein promotes resistance to infection by rapid increase of NO. | Zhang et al. (2014) |
B. cinerea | Nicotiana benthamiana (leaf) | Path | DAF-2DA | 2–12 d | NOS, NR | NbPR-1/NbLOX/NbGST/NbCAT1 | DPI (50 μM), L-NAME (5 mM), D-NAME (50 μM), cPTIO (500 μM) | NbNOA1/NbRBOHB VIGS | NO contributes to resistance | Asai and Yoshioka (2009) |
B. cinerea | Pelargonium peltatum (leaf) | Path | DAF-2DA/PGSTAT 30 | 5 min–3 d | – | – | – | – | An early NO burst and a later wave of NO generation enhances resistance. | Floryszak-Wieczorek et al. (2007) |
B. cinerea | Solanum lycopersicum, Nicotiana tabacum, Arabidopsis thaliana (leaf) | Path | DAF-2DA | 1–4 d | – | – | c-PTIO (0.25 mM), L-NAME (5 mM) | – | A NO concentration threshold triggers plant cell death. Below the threshold, NO acts as a signalling molecule to activate diverse plant defence systems. | Turrion-Gomez and Benito (2011) |
B. cinerea | Solanum lycopersicum (leaf) | Path | Quantum cascade laser | 30 min–24 h | NR | – | L-NAME (5 mM), SNP (0.1 mM) | ABA mutant sitiens | ABA can decrease resistance via reduction of NO production. | Sivakumaran et al. (2016) |
B. cinerea | Solanum tuberosum cv. Bintje/Bzura (leaf) | Path | Electrochemical method | 0–24 h | – | PR-1/PR-2/PR-3 | – | – | Infection triggers huge NO over-production. | Floryszak-Wieczorek and Arasimowicz-Jelonek (2016) |
Colletotrichum orbiculare | Nicotiana. benthamiama (leaf) | Path | DAF-2DA | 4–6 d | NR, NOS non-enzymatic | – | Tungstate (100 mM) | NOA1-silenced plants (VIGS) | NO helps to defend the plant. Post-transcriptional control of NOA1 influences NO production and is affected through the MEK2 SIPK/NTF4 cascade. | Asai et al. (2008) |
chitiosan (fungal elicitor) | Pisum. sativum (leaf) | Path | DAF-2DA | 10–20 min | NR, NOS | – | cPTIO (0.2 mM), L-NAME (0.1 mM), Tungstate (0.1 mM) | – | NO production may be responsive to fungal PAMPs. | Srivastava et al. (2009) |
Funneliformis mosseae (AMF) | Trifolium repens (root) | Benef | DAF-FM DA | 5–9 weeks | – | PAL/CHS | – | – | AMF increases NO levels in roots, independently of the mycorrhization time. | Zhang et al. (2013) |
F. mosseae (AMF) | Trifolium repens(root) | Benef | DAF-FM DA | 5–9 weeks | – | PAL/CHS | – | – | AMF increases NO in roots, but not systemically to non-mycorrhizal roots in a split-root system. | Zhu et al. (2015) |
Fusarium oxysporum/ Trichoderma asperelloides | Arabidopsis thaliana (root) | Path, Benef | DAF-2DA | 10–120 min | – | 78 NO-modulated genes | cPTIO (100 µM), L-NAME (2.5 mM) | nia1nia2 | T. asperelloides suppresses NO generation elicited by F. oxysporum. | Gupta et al. (2014) |
F. oxysporum (fusaric acid, FA) | Nicotiana tabacum (cells) | Path | DAF-2, DAF-FM DA | 15–90 min | – | PAL/Hsr203J | cPTIO (100 mM), L-NMMA (100 mM) | – | FA can induce programmed cell death in tobacco suspension cells in a NO-dependent way. | Jiao et al. (2013) |
F. oxysporum | Solanum lycopersicum (root) | Path | DAF-2DA, Haemoglobin assay | 48 h | NR | PRs/PAL/ProtIn/PO/GST/CAM/NR | SNP (100 µM), cPTIO (100 µM), L-NAME (10 µM) | – | Ca-treated plants show higher NO production than control. Disease incidence is reduced in Ca-treated plants, which may be due to the higher NO concentration. | Chakraborty et al. (2017) |
F. oxysporum (fungal elicitor) | Trifolium chinensis (cells) | Path | DAF-2 DA | 0–12 h | NOS | PAL | SNP (10 µM), L-NNA (100 µM), PTIO (100 µM) | – | NO activates fungal elicitor-induced responses involving secondary metabolism. | Wang and Wu (2004) |
Gigaspora margarita (exudates) | Medicago truncatula (root) | Benef (symbiosis) | DAF-2DA | 0–15 min | NR | NR/NiR | cPTIO (1 mM) | Transgenic roots, (DMI1-1, DMI2-2, and DMI3-1) | There is a NO specific signature related to AM interactions and a different NO signature when plants are exposed to a general elicitor such as bacterial lipopolysaccharides extract. | Calcagno et al. (2012) |
Magnaporthe grisea (cell wall) | Oriza sativa (leaf/cells) | Path | Greiss regeant | 30 min; 12 h | NOS | PAL/PR-1/CHI | NO acts as a signal mediating the HR induced by the fungus and it is also necessary for the induction of cell death in combination with H2O2. | Hu et al. (2003) | ||
M. oryzae (Nep1Mo) | Arabidopsis thaliana (leaf) | Path | DAF-2DA | 3 h | – | AtERF1/AtLOX3 | SNP (25 mM), cPTIO (400 µM) | AtALY4 | AtAlY4–H2O2–NO pathway mediates multiple Nep1Mo-triggered responses, including stomatal closure, hypersensitive cell death, and defence-related gene expression. | Teng et al. (2014) |
M. oryzae | Hordeum vulgare, Oriza sativa (leaf) | Path | – | – | – | – | PTIO (250–500 µM) | – | Removal of NO delays fungal germination and reduces disease lesion numbers. | Samalova et al. (2013) |
Macrophomina phaseolina and xylanase | Corchorus capsularis (leaf) | Path | DAF-FM DA | 8 h | – | – | cPTIO (200 mM) | – | Low NO concentration functions as a signalling molecule. High NO concentrations facilitate fungal infection by triggering programmed cell death. M. phaseolina can enhance the infection of plant cells through its own production of NO. | Sarkar et al. (2014) |
Oidium neolycopersici | Solanum lycopersicum cv. Amateur/chmielewskii/hirsutum f. glabratum (leaf) | Path | Oxyhemoglobin method, DAF-FM DA | 0–216 h | NOS | – | cPTIO (0.1 mM), L-NAME (10 mM), AMG (10 mM) | – | NO levels are higher in resistant varieties, leading to plant resistance. | Piterková et al. (2009) |
O. neolycopersici | Solanum lycopersicum/chmielewskii/habrochaites f. glabratum (leaf/disc) | Path | DAF-FM DA | 8–72 h | NOS | – | SNP (0.1 mM), L-NAME (1 mM), PTIO (0.1 mM) | – | In a moderately susceptible genotype the disease rate is diminished if NO production by NOS is reduced. NO activates defences in resistant genotype. With cPTIO, the fungus germinates better on the leaves. | Piterková et al. (2011) |
Puccinia striiformis CY22- 2/CY29-1 | Triticum aestivum cv. Lovrin10 (leaf) | Path | Electron spin resonance | 0–120 h | – | – | SNP (0.5; 2.5 mM) | – | There is a general correlation of NO formation and race-specific resistance. | Guo et al. (2004) |
P. coronata f.sp. avenae | Avena sativa (leaf) | Path | DAF | 12–60 h | – | – | cPTIO (500 µM) | – | Simultaneous generation of NO and H2O2 might be associated with the death of cells adjacent to those infected by the avirulent fungal isolate. | Tada et al. (2004) |
P. triticina | Arabidopsis thaliana, Triticum aestivum (leaf) | Path | DAF-DA | 24 h | – | – | – | atrbohD/atrbohF/atrbohD+F/A. thaliana (natural variation) | Identification of loci controlling non-host disease resistance and changes in NO levels. | Shafiei et al. (2007) |
P. triticina | Triticum aestivum (leaf) | Path | DAF-FM DA | 4–72 h | NR, NOS | – | Na2WO4 (100 μM), c-PTIO (200 μM), L-NAME (100 μM) | – | In this incompatible combination NO acts as an important signalling molecule and mediates HR. | Qiao et al. (2015) |
Trichoderma brevicompactum | Arabidopsis thaliana (leaf) | Path | DAF-DA | 2 h | – | – | Alamethicin (50 µM) | – | rRNA cleavage is suppressed by NO. | Rippa et al. (2007) |
Verticillium dahliae (VD-toxins) | Arabidopsis thaliana (leaf) | Path | DAF-2-DA | 45 min | – | PR-1 | Tungstate (100 µM), cPTIO (100 µM) | Atnoa1 | Cortical microtubule dynamics are mediated by NO-dependent signalling. | Shi et al. (2009) |
V. dahliae (VD-toxins) | Arabidopsis thaliana (leaf) | Path | DAF-2-DA | 60 min | NR | – | Tungstate, cPTIO | nia1nia2 | VD toxin-induced NO accumulation is H2O2 dependent, and H2O2 acts synergistically with NO to modulate the dynamic microtubule cytoskeleton responses to VD toxins. | Yao et al. (2012) |
V. dahliae/Rhizophagus irregularis | Olea europaea (root) | Path, Benef | DAF-2DA | 1–24 h | – | – | PTIO (400 mM) | – | NO may be a key in the establishment of symbiosis and the defence response to pathogens. | Espinosa et al. (2014) |
V. dahliae | Arabidopsis thaliana (leaf) | Path | DAF2-DA | 60 min | – | – | SNP (400 µM) | GhHb1-transgenic Arabidopsis | GhHb1 proteins play a role in the defence responses against pathogenic invasions, possibly by modulating the NO level and the ratio of H2O2/NO in the defence process. | Qu et al. (2006) |
V. dahliae | Arabidopsis thaliana (leaf) | Path | DAF-2-DA | 50–60 min | NR | NIA1 | Tungstate (100 µM), L-NNA (100 µM), cPTIO (100 µM) | Atnoa1/nia1/nia2 | NO is induced in response to VD toxins. | Shi and Li (2008) |
V. dahliae | Helianthus annuus (root) | Path | – | – | – | – | SNP (20 µM), GSNO (50 µM) | – | NO pre-treatments do not reduce wilt symptoms. NO donors appear to promote fungal infection. | Monzón et al. (2015) |
V. longisporum | Arabidopsis thaliana (root/leaf) | Path | DAF-2 | 50–80 min | – | Genes analysis at NO peak | – | – | NO may regulate 732 genes in the roots and 474 genes in the shoot. | Tischner et al. (2010) |
* Path, pathological; Benef, beneficial.
A summary of studies where NO production in plant–fungal interactions have been demonstrated, together with its proposed role
Fungus . | Plant . | Type of interaction* . | NO level (technique) . | Time scale . | NO source . | Gene expression . | Pharmacological approach . | Genetic approach . | Suggested function . | Reference . |
---|---|---|---|---|---|---|---|---|---|---|
Blumeria graminis | Hordeum vulgare (leaf) | Path | DAF-2DA | 6–24 h | – | – | cPTIO (0.25 mM), SNP (0.05 mM), L-NAME (1 mM) | – | NO contributes to HR and cell death, leading to infection being halted. NO also contributes to papilla formation. | Prats et al. (2005) |
Botrytis cinerea | Arabidopsis thaliana (leaf) | Path | DAF-2DA | 6 d | – | PR1/LOX2/LOX3/AOS/OPR3/VSP2/GDSL/ERF2 + array | N-isobutyl decanamide (60 μM) | Jar1/Coi1/Eds16/Mpk6 | Alkamides are involved in induction of plant immunity and change NO levels. | Méndez-Bravo et al. (2011) |
B. cinerea | Arabidopsis thaliana (leaf) | Path | DAF-2DA | 30 min–6 h | NR, Arg | – | OG, L-NAME (5 mM), cPTIO (500 µM), Tungstate (µM) | nia1nia2/cngc2/per4-1/per4-2/glu/RBOH-D | NO participates in the regulation of OG-responsive genes (PER4/a b-1,3-glucanase). Plants treated with cPTIO are more susceptible to the fungus. | Rasul et al. (2012) |
B. cinerea (PebC1) | Arabidopsis thaliana (leaf/cells) | Path | Greiss regeant | 3–6 h | – | PR1/BGL-2/PR4/PDF1.2/This2.1 | – | Ein2/Coi1/Npr1/NahG | PebC1 protein promotes resistance to infection by rapid increase of NO. | Zhang et al. (2014) |
B. cinerea | Nicotiana benthamiana (leaf) | Path | DAF-2DA | 2–12 d | NOS, NR | NbPR-1/NbLOX/NbGST/NbCAT1 | DPI (50 μM), L-NAME (5 mM), D-NAME (50 μM), cPTIO (500 μM) | NbNOA1/NbRBOHB VIGS | NO contributes to resistance | Asai and Yoshioka (2009) |
B. cinerea | Pelargonium peltatum (leaf) | Path | DAF-2DA/PGSTAT 30 | 5 min–3 d | – | – | – | – | An early NO burst and a later wave of NO generation enhances resistance. | Floryszak-Wieczorek et al. (2007) |
B. cinerea | Solanum lycopersicum, Nicotiana tabacum, Arabidopsis thaliana (leaf) | Path | DAF-2DA | 1–4 d | – | – | c-PTIO (0.25 mM), L-NAME (5 mM) | – | A NO concentration threshold triggers plant cell death. Below the threshold, NO acts as a signalling molecule to activate diverse plant defence systems. | Turrion-Gomez and Benito (2011) |
B. cinerea | Solanum lycopersicum (leaf) | Path | Quantum cascade laser | 30 min–24 h | NR | – | L-NAME (5 mM), SNP (0.1 mM) | ABA mutant sitiens | ABA can decrease resistance via reduction of NO production. | Sivakumaran et al. (2016) |
B. cinerea | Solanum tuberosum cv. Bintje/Bzura (leaf) | Path | Electrochemical method | 0–24 h | – | PR-1/PR-2/PR-3 | – | – | Infection triggers huge NO over-production. | Floryszak-Wieczorek and Arasimowicz-Jelonek (2016) |
Colletotrichum orbiculare | Nicotiana. benthamiama (leaf) | Path | DAF-2DA | 4–6 d | NR, NOS non-enzymatic | – | Tungstate (100 mM) | NOA1-silenced plants (VIGS) | NO helps to defend the plant. Post-transcriptional control of NOA1 influences NO production and is affected through the MEK2 SIPK/NTF4 cascade. | Asai et al. (2008) |
chitiosan (fungal elicitor) | Pisum. sativum (leaf) | Path | DAF-2DA | 10–20 min | NR, NOS | – | cPTIO (0.2 mM), L-NAME (0.1 mM), Tungstate (0.1 mM) | – | NO production may be responsive to fungal PAMPs. | Srivastava et al. (2009) |
Funneliformis mosseae (AMF) | Trifolium repens (root) | Benef | DAF-FM DA | 5–9 weeks | – | PAL/CHS | – | – | AMF increases NO levels in roots, independently of the mycorrhization time. | Zhang et al. (2013) |
F. mosseae (AMF) | Trifolium repens(root) | Benef | DAF-FM DA | 5–9 weeks | – | PAL/CHS | – | – | AMF increases NO in roots, but not systemically to non-mycorrhizal roots in a split-root system. | Zhu et al. (2015) |
Fusarium oxysporum/ Trichoderma asperelloides | Arabidopsis thaliana (root) | Path, Benef | DAF-2DA | 10–120 min | – | 78 NO-modulated genes | cPTIO (100 µM), L-NAME (2.5 mM) | nia1nia2 | T. asperelloides suppresses NO generation elicited by F. oxysporum. | Gupta et al. (2014) |
F. oxysporum (fusaric acid, FA) | Nicotiana tabacum (cells) | Path | DAF-2, DAF-FM DA | 15–90 min | – | PAL/Hsr203J | cPTIO (100 mM), L-NMMA (100 mM) | – | FA can induce programmed cell death in tobacco suspension cells in a NO-dependent way. | Jiao et al. (2013) |
F. oxysporum | Solanum lycopersicum (root) | Path | DAF-2DA, Haemoglobin assay | 48 h | NR | PRs/PAL/ProtIn/PO/GST/CAM/NR | SNP (100 µM), cPTIO (100 µM), L-NAME (10 µM) | – | Ca-treated plants show higher NO production than control. Disease incidence is reduced in Ca-treated plants, which may be due to the higher NO concentration. | Chakraborty et al. (2017) |
F. oxysporum (fungal elicitor) | Trifolium chinensis (cells) | Path | DAF-2 DA | 0–12 h | NOS | PAL | SNP (10 µM), L-NNA (100 µM), PTIO (100 µM) | – | NO activates fungal elicitor-induced responses involving secondary metabolism. | Wang and Wu (2004) |
Gigaspora margarita (exudates) | Medicago truncatula (root) | Benef (symbiosis) | DAF-2DA | 0–15 min | NR | NR/NiR | cPTIO (1 mM) | Transgenic roots, (DMI1-1, DMI2-2, and DMI3-1) | There is a NO specific signature related to AM interactions and a different NO signature when plants are exposed to a general elicitor such as bacterial lipopolysaccharides extract. | Calcagno et al. (2012) |
Magnaporthe grisea (cell wall) | Oriza sativa (leaf/cells) | Path | Greiss regeant | 30 min; 12 h | NOS | PAL/PR-1/CHI | NO acts as a signal mediating the HR induced by the fungus and it is also necessary for the induction of cell death in combination with H2O2. | Hu et al. (2003) | ||
M. oryzae (Nep1Mo) | Arabidopsis thaliana (leaf) | Path | DAF-2DA | 3 h | – | AtERF1/AtLOX3 | SNP (25 mM), cPTIO (400 µM) | AtALY4 | AtAlY4–H2O2–NO pathway mediates multiple Nep1Mo-triggered responses, including stomatal closure, hypersensitive cell death, and defence-related gene expression. | Teng et al. (2014) |
M. oryzae | Hordeum vulgare, Oriza sativa (leaf) | Path | – | – | – | – | PTIO (250–500 µM) | – | Removal of NO delays fungal germination and reduces disease lesion numbers. | Samalova et al. (2013) |
Macrophomina phaseolina and xylanase | Corchorus capsularis (leaf) | Path | DAF-FM DA | 8 h | – | – | cPTIO (200 mM) | – | Low NO concentration functions as a signalling molecule. High NO concentrations facilitate fungal infection by triggering programmed cell death. M. phaseolina can enhance the infection of plant cells through its own production of NO. | Sarkar et al. (2014) |
Oidium neolycopersici | Solanum lycopersicum cv. Amateur/chmielewskii/hirsutum f. glabratum (leaf) | Path | Oxyhemoglobin method, DAF-FM DA | 0–216 h | NOS | – | cPTIO (0.1 mM), L-NAME (10 mM), AMG (10 mM) | – | NO levels are higher in resistant varieties, leading to plant resistance. | Piterková et al. (2009) |
O. neolycopersici | Solanum lycopersicum/chmielewskii/habrochaites f. glabratum (leaf/disc) | Path | DAF-FM DA | 8–72 h | NOS | – | SNP (0.1 mM), L-NAME (1 mM), PTIO (0.1 mM) | – | In a moderately susceptible genotype the disease rate is diminished if NO production by NOS is reduced. NO activates defences in resistant genotype. With cPTIO, the fungus germinates better on the leaves. | Piterková et al. (2011) |
Puccinia striiformis CY22- 2/CY29-1 | Triticum aestivum cv. Lovrin10 (leaf) | Path | Electron spin resonance | 0–120 h | – | – | SNP (0.5; 2.5 mM) | – | There is a general correlation of NO formation and race-specific resistance. | Guo et al. (2004) |
P. coronata f.sp. avenae | Avena sativa (leaf) | Path | DAF | 12–60 h | – | – | cPTIO (500 µM) | – | Simultaneous generation of NO and H2O2 might be associated with the death of cells adjacent to those infected by the avirulent fungal isolate. | Tada et al. (2004) |
P. triticina | Arabidopsis thaliana, Triticum aestivum (leaf) | Path | DAF-DA | 24 h | – | – | – | atrbohD/atrbohF/atrbohD+F/A. thaliana (natural variation) | Identification of loci controlling non-host disease resistance and changes in NO levels. | Shafiei et al. (2007) |
P. triticina | Triticum aestivum (leaf) | Path | DAF-FM DA | 4–72 h | NR, NOS | – | Na2WO4 (100 μM), c-PTIO (200 μM), L-NAME (100 μM) | – | In this incompatible combination NO acts as an important signalling molecule and mediates HR. | Qiao et al. (2015) |
Trichoderma brevicompactum | Arabidopsis thaliana (leaf) | Path | DAF-DA | 2 h | – | – | Alamethicin (50 µM) | – | rRNA cleavage is suppressed by NO. | Rippa et al. (2007) |
Verticillium dahliae (VD-toxins) | Arabidopsis thaliana (leaf) | Path | DAF-2-DA | 45 min | – | PR-1 | Tungstate (100 µM), cPTIO (100 µM) | Atnoa1 | Cortical microtubule dynamics are mediated by NO-dependent signalling. | Shi et al. (2009) |
V. dahliae (VD-toxins) | Arabidopsis thaliana (leaf) | Path | DAF-2-DA | 60 min | NR | – | Tungstate, cPTIO | nia1nia2 | VD toxin-induced NO accumulation is H2O2 dependent, and H2O2 acts synergistically with NO to modulate the dynamic microtubule cytoskeleton responses to VD toxins. | Yao et al. (2012) |
V. dahliae/Rhizophagus irregularis | Olea europaea (root) | Path, Benef | DAF-2DA | 1–24 h | – | – | PTIO (400 mM) | – | NO may be a key in the establishment of symbiosis and the defence response to pathogens. | Espinosa et al. (2014) |
V. dahliae | Arabidopsis thaliana (leaf) | Path | DAF2-DA | 60 min | – | – | SNP (400 µM) | GhHb1-transgenic Arabidopsis | GhHb1 proteins play a role in the defence responses against pathogenic invasions, possibly by modulating the NO level and the ratio of H2O2/NO in the defence process. | Qu et al. (2006) |
V. dahliae | Arabidopsis thaliana (leaf) | Path | DAF-2-DA | 50–60 min | NR | NIA1 | Tungstate (100 µM), L-NNA (100 µM), cPTIO (100 µM) | Atnoa1/nia1/nia2 | NO is induced in response to VD toxins. | Shi and Li (2008) |
V. dahliae | Helianthus annuus (root) | Path | – | – | – | – | SNP (20 µM), GSNO (50 µM) | – | NO pre-treatments do not reduce wilt symptoms. NO donors appear to promote fungal infection. | Monzón et al. (2015) |
V. longisporum | Arabidopsis thaliana (root/leaf) | Path | DAF-2 | 50–80 min | – | Genes analysis at NO peak | – | – | NO may regulate 732 genes in the roots and 474 genes in the shoot. | Tischner et al. (2010) |
Fungus . | Plant . | Type of interaction* . | NO level (technique) . | Time scale . | NO source . | Gene expression . | Pharmacological approach . | Genetic approach . | Suggested function . | Reference . |
---|---|---|---|---|---|---|---|---|---|---|
Blumeria graminis | Hordeum vulgare (leaf) | Path | DAF-2DA | 6–24 h | – | – | cPTIO (0.25 mM), SNP (0.05 mM), L-NAME (1 mM) | – | NO contributes to HR and cell death, leading to infection being halted. NO also contributes to papilla formation. | Prats et al. (2005) |
Botrytis cinerea | Arabidopsis thaliana (leaf) | Path | DAF-2DA | 6 d | – | PR1/LOX2/LOX3/AOS/OPR3/VSP2/GDSL/ERF2 + array | N-isobutyl decanamide (60 μM) | Jar1/Coi1/Eds16/Mpk6 | Alkamides are involved in induction of plant immunity and change NO levels. | Méndez-Bravo et al. (2011) |
B. cinerea | Arabidopsis thaliana (leaf) | Path | DAF-2DA | 30 min–6 h | NR, Arg | – | OG, L-NAME (5 mM), cPTIO (500 µM), Tungstate (µM) | nia1nia2/cngc2/per4-1/per4-2/glu/RBOH-D | NO participates in the regulation of OG-responsive genes (PER4/a b-1,3-glucanase). Plants treated with cPTIO are more susceptible to the fungus. | Rasul et al. (2012) |
B. cinerea (PebC1) | Arabidopsis thaliana (leaf/cells) | Path | Greiss regeant | 3–6 h | – | PR1/BGL-2/PR4/PDF1.2/This2.1 | – | Ein2/Coi1/Npr1/NahG | PebC1 protein promotes resistance to infection by rapid increase of NO. | Zhang et al. (2014) |
B. cinerea | Nicotiana benthamiana (leaf) | Path | DAF-2DA | 2–12 d | NOS, NR | NbPR-1/NbLOX/NbGST/NbCAT1 | DPI (50 μM), L-NAME (5 mM), D-NAME (50 μM), cPTIO (500 μM) | NbNOA1/NbRBOHB VIGS | NO contributes to resistance | Asai and Yoshioka (2009) |
B. cinerea | Pelargonium peltatum (leaf) | Path | DAF-2DA/PGSTAT 30 | 5 min–3 d | – | – | – | – | An early NO burst and a later wave of NO generation enhances resistance. | Floryszak-Wieczorek et al. (2007) |
B. cinerea | Solanum lycopersicum, Nicotiana tabacum, Arabidopsis thaliana (leaf) | Path | DAF-2DA | 1–4 d | – | – | c-PTIO (0.25 mM), L-NAME (5 mM) | – | A NO concentration threshold triggers plant cell death. Below the threshold, NO acts as a signalling molecule to activate diverse plant defence systems. | Turrion-Gomez and Benito (2011) |
B. cinerea | Solanum lycopersicum (leaf) | Path | Quantum cascade laser | 30 min–24 h | NR | – | L-NAME (5 mM), SNP (0.1 mM) | ABA mutant sitiens | ABA can decrease resistance via reduction of NO production. | Sivakumaran et al. (2016) |
B. cinerea | Solanum tuberosum cv. Bintje/Bzura (leaf) | Path | Electrochemical method | 0–24 h | – | PR-1/PR-2/PR-3 | – | – | Infection triggers huge NO over-production. | Floryszak-Wieczorek and Arasimowicz-Jelonek (2016) |
Colletotrichum orbiculare | Nicotiana. benthamiama (leaf) | Path | DAF-2DA | 4–6 d | NR, NOS non-enzymatic | – | Tungstate (100 mM) | NOA1-silenced plants (VIGS) | NO helps to defend the plant. Post-transcriptional control of NOA1 influences NO production and is affected through the MEK2 SIPK/NTF4 cascade. | Asai et al. (2008) |
chitiosan (fungal elicitor) | Pisum. sativum (leaf) | Path | DAF-2DA | 10–20 min | NR, NOS | – | cPTIO (0.2 mM), L-NAME (0.1 mM), Tungstate (0.1 mM) | – | NO production may be responsive to fungal PAMPs. | Srivastava et al. (2009) |
Funneliformis mosseae (AMF) | Trifolium repens (root) | Benef | DAF-FM DA | 5–9 weeks | – | PAL/CHS | – | – | AMF increases NO levels in roots, independently of the mycorrhization time. | Zhang et al. (2013) |
F. mosseae (AMF) | Trifolium repens(root) | Benef | DAF-FM DA | 5–9 weeks | – | PAL/CHS | – | – | AMF increases NO in roots, but not systemically to non-mycorrhizal roots in a split-root system. | Zhu et al. (2015) |
Fusarium oxysporum/ Trichoderma asperelloides | Arabidopsis thaliana (root) | Path, Benef | DAF-2DA | 10–120 min | – | 78 NO-modulated genes | cPTIO (100 µM), L-NAME (2.5 mM) | nia1nia2 | T. asperelloides suppresses NO generation elicited by F. oxysporum. | Gupta et al. (2014) |
F. oxysporum (fusaric acid, FA) | Nicotiana tabacum (cells) | Path | DAF-2, DAF-FM DA | 15–90 min | – | PAL/Hsr203J | cPTIO (100 mM), L-NMMA (100 mM) | – | FA can induce programmed cell death in tobacco suspension cells in a NO-dependent way. | Jiao et al. (2013) |
F. oxysporum | Solanum lycopersicum (root) | Path | DAF-2DA, Haemoglobin assay | 48 h | NR | PRs/PAL/ProtIn/PO/GST/CAM/NR | SNP (100 µM), cPTIO (100 µM), L-NAME (10 µM) | – | Ca-treated plants show higher NO production than control. Disease incidence is reduced in Ca-treated plants, which may be due to the higher NO concentration. | Chakraborty et al. (2017) |
F. oxysporum (fungal elicitor) | Trifolium chinensis (cells) | Path | DAF-2 DA | 0–12 h | NOS | PAL | SNP (10 µM), L-NNA (100 µM), PTIO (100 µM) | – | NO activates fungal elicitor-induced responses involving secondary metabolism. | Wang and Wu (2004) |
Gigaspora margarita (exudates) | Medicago truncatula (root) | Benef (symbiosis) | DAF-2DA | 0–15 min | NR | NR/NiR | cPTIO (1 mM) | Transgenic roots, (DMI1-1, DMI2-2, and DMI3-1) | There is a NO specific signature related to AM interactions and a different NO signature when plants are exposed to a general elicitor such as bacterial lipopolysaccharides extract. | Calcagno et al. (2012) |
Magnaporthe grisea (cell wall) | Oriza sativa (leaf/cells) | Path | Greiss regeant | 30 min; 12 h | NOS | PAL/PR-1/CHI | NO acts as a signal mediating the HR induced by the fungus and it is also necessary for the induction of cell death in combination with H2O2. | Hu et al. (2003) | ||
M. oryzae (Nep1Mo) | Arabidopsis thaliana (leaf) | Path | DAF-2DA | 3 h | – | AtERF1/AtLOX3 | SNP (25 mM), cPTIO (400 µM) | AtALY4 | AtAlY4–H2O2–NO pathway mediates multiple Nep1Mo-triggered responses, including stomatal closure, hypersensitive cell death, and defence-related gene expression. | Teng et al. (2014) |
M. oryzae | Hordeum vulgare, Oriza sativa (leaf) | Path | – | – | – | – | PTIO (250–500 µM) | – | Removal of NO delays fungal germination and reduces disease lesion numbers. | Samalova et al. (2013) |
Macrophomina phaseolina and xylanase | Corchorus capsularis (leaf) | Path | DAF-FM DA | 8 h | – | – | cPTIO (200 mM) | – | Low NO concentration functions as a signalling molecule. High NO concentrations facilitate fungal infection by triggering programmed cell death. M. phaseolina can enhance the infection of plant cells through its own production of NO. | Sarkar et al. (2014) |
Oidium neolycopersici | Solanum lycopersicum cv. Amateur/chmielewskii/hirsutum f. glabratum (leaf) | Path | Oxyhemoglobin method, DAF-FM DA | 0–216 h | NOS | – | cPTIO (0.1 mM), L-NAME (10 mM), AMG (10 mM) | – | NO levels are higher in resistant varieties, leading to plant resistance. | Piterková et al. (2009) |
O. neolycopersici | Solanum lycopersicum/chmielewskii/habrochaites f. glabratum (leaf/disc) | Path | DAF-FM DA | 8–72 h | NOS | – | SNP (0.1 mM), L-NAME (1 mM), PTIO (0.1 mM) | – | In a moderately susceptible genotype the disease rate is diminished if NO production by NOS is reduced. NO activates defences in resistant genotype. With cPTIO, the fungus germinates better on the leaves. | Piterková et al. (2011) |
Puccinia striiformis CY22- 2/CY29-1 | Triticum aestivum cv. Lovrin10 (leaf) | Path | Electron spin resonance | 0–120 h | – | – | SNP (0.5; 2.5 mM) | – | There is a general correlation of NO formation and race-specific resistance. | Guo et al. (2004) |
P. coronata f.sp. avenae | Avena sativa (leaf) | Path | DAF | 12–60 h | – | – | cPTIO (500 µM) | – | Simultaneous generation of NO and H2O2 might be associated with the death of cells adjacent to those infected by the avirulent fungal isolate. | Tada et al. (2004) |
P. triticina | Arabidopsis thaliana, Triticum aestivum (leaf) | Path | DAF-DA | 24 h | – | – | – | atrbohD/atrbohF/atrbohD+F/A. thaliana (natural variation) | Identification of loci controlling non-host disease resistance and changes in NO levels. | Shafiei et al. (2007) |
P. triticina | Triticum aestivum (leaf) | Path | DAF-FM DA | 4–72 h | NR, NOS | – | Na2WO4 (100 μM), c-PTIO (200 μM), L-NAME (100 μM) | – | In this incompatible combination NO acts as an important signalling molecule and mediates HR. | Qiao et al. (2015) |
Trichoderma brevicompactum | Arabidopsis thaliana (leaf) | Path | DAF-DA | 2 h | – | – | Alamethicin (50 µM) | – | rRNA cleavage is suppressed by NO. | Rippa et al. (2007) |
Verticillium dahliae (VD-toxins) | Arabidopsis thaliana (leaf) | Path | DAF-2-DA | 45 min | – | PR-1 | Tungstate (100 µM), cPTIO (100 µM) | Atnoa1 | Cortical microtubule dynamics are mediated by NO-dependent signalling. | Shi et al. (2009) |
V. dahliae (VD-toxins) | Arabidopsis thaliana (leaf) | Path | DAF-2-DA | 60 min | NR | – | Tungstate, cPTIO | nia1nia2 | VD toxin-induced NO accumulation is H2O2 dependent, and H2O2 acts synergistically with NO to modulate the dynamic microtubule cytoskeleton responses to VD toxins. | Yao et al. (2012) |
V. dahliae/Rhizophagus irregularis | Olea europaea (root) | Path, Benef | DAF-2DA | 1–24 h | – | – | PTIO (400 mM) | – | NO may be a key in the establishment of symbiosis and the defence response to pathogens. | Espinosa et al. (2014) |
V. dahliae | Arabidopsis thaliana (leaf) | Path | DAF2-DA | 60 min | – | – | SNP (400 µM) | GhHb1-transgenic Arabidopsis | GhHb1 proteins play a role in the defence responses against pathogenic invasions, possibly by modulating the NO level and the ratio of H2O2/NO in the defence process. | Qu et al. (2006) |
V. dahliae | Arabidopsis thaliana (leaf) | Path | DAF-2-DA | 50–60 min | NR | NIA1 | Tungstate (100 µM), L-NNA (100 µM), cPTIO (100 µM) | Atnoa1/nia1/nia2 | NO is induced in response to VD toxins. | Shi and Li (2008) |
V. dahliae | Helianthus annuus (root) | Path | – | – | – | – | SNP (20 µM), GSNO (50 µM) | – | NO pre-treatments do not reduce wilt symptoms. NO donors appear to promote fungal infection. | Monzón et al. (2015) |
V. longisporum | Arabidopsis thaliana (root/leaf) | Path | DAF-2 | 50–80 min | – | Genes analysis at NO peak | – | – | NO may regulate 732 genes in the roots and 474 genes in the shoot. | Tischner et al. (2010) |
* Path, pathological; Benef, beneficial.
Necrotrophic fungi
The well-characterized necrotrophic foliar pathogen Botrytis cinerea has been used to demonstrate the importance of NO in the onset of the plant immune response against shoot-associated necrotrophic fungi in different plant species. For example, B. cinerea infection of tobacco (Nicotiana benthamiana) triggers an increase in NO levels in cells adjacent to invaded areas, which is concomitant with the activation of the salicylic acid -regulated defence pathway (Asai and Yoshioka, 2009). By using a pharmacological approach, these authors also showed that NO plays a pivotal role in the basal defence against B. cinerea, and in pathogen-triggered expression of PR-1. Similarly, an increase in NO was observed in cells infected with B. cinerea and in surrounding uninfected cells in Arabidopsis (van Baarlen et al., 2007). The critical role of NO in Arabidopsis resistance to B. cinerea was later confirmed by manipulation of NO levels through a genetic approach (Mur et al., 2012), with mutant lines that displayed increased NO levels (due to a mutation in the Phytogb1 gene) showing increased levels of the defence-related plant hormones jasmonic acid and ethylene together with an increased resistance to B. cinerea infection, whilst decreased NO levels in Phytogb1-overexpressing lines resulted in an opposite phenotype. Pharmacological approaches have also revealed the importance of the NO burst in plant resistance against B. cinerea in tomato (Solanum lycopersicum; Sivakumaran et al., 2016). Taken together, these studies demonstrate a key role of pathogen-triggered NO in plant immunity against B. cinerea in different plant species. A similar role for NO has been suggested for plant immune responses against other leaf-associated necrotrophic fungi such as Colletotrichum orbiculare (Asai et al., 2008) and Sclerotinia sclerotiorum (Perchepied et al., 2010).
Strikingly, a study by Turrion-Gomez and Benito (2011) indicated that B. cinerea may use NO signalling to enhance its spreading within plant cells. Although the authors mostly focused on NO produced by the fungus, they hypothesized that the plant cell death mediated by the NO-triggered HR might favour the growth of the necrotrophic fungus within plant tissues. It is notable that we recently found that B. cinerea triggered the down-regulation of Phytogb1 in tomato leaves, most likely to increase NO levels and to enhance cell death (Martínez-Medina et al., 2019a). This offers an apparently contradictory scenario where NO is being used by the host plant for defence and by the pathogenic fungus to promote virulence. Understanding this disparate data will require careful spatio-temporal measurement of NO concentrations (Box 1), as the relative concentration of NO during the different stages of the infection process could play a key role in governing its action. Indeed, Turrion-Gomez and Benito (2011) hypothesized that above a certain threshold NO triggers plant cell death, which would favour the infection, while below this threshold NO would act as a key signalling molecule in the onset of the plant immune response. In line with this hypothesis, Floryszak-Wieczorek et al. (2007) found uncontrolled generation of NO in tissues of susceptible Pelargonium peltatum infected with B. cinerea. This was accompanied by very intensive synthesis of H2O2 and ethylene. Moreover, when the pathogen colonized susceptible cells it further produced considerable amounts of NO, which enhanced the nitrosative and oxidative stress in host tissues. By contrast, a more controlled burst of NO was observed in the incompatible interaction of B. cinerea with a resistant Pelargonium genotype. In this case, the resistance response was accompanied by a strong first burst of NO followed by a controlled secondary wave of NO generation, which was co-expressed with the activation of plant defences. This response triggered a resistance that was not associated with cell death but which did have an enhanced pool of antioxidants, which ultimately favoured the maintenance of homeostasis of the surrounding cells. According to these findings, in susceptible interactions, necrotrophic fungi may exploit the NO-related plant defence system in order to expand the infection. However, in incompatible interactions, NO would be mostly acting as a key signal in the onset of the plant immune response.
The role of NO in plant–fungal interactions is of considerable complexity, as it has a regulatory role in both plant defence responses and in the process of pathogenicity and/or the proper establishment of beneficial interactions. Accordingly, we need a more accurate understanding of NO dynamics, distribution, and function in specific plant–fungal interactions. Such knowledge should contribute to the improvement of biotechnological applications for crop resistance through the identification of key regulation points that determine pathogenicity or beneficial effects of microbial inoculants.
We consider that the following technical and experimental challenges need to be addressed.
Development of appropriate NO sensors to allow monitoring of levels in vivo in order to follow the spatial and temporal dynamics and to identify the source of NO production during plant–fungal interactions.
Functional studies need to be conducted in which plant or fungal NO levels are manipulated at specific sites or time-points in order to determine their impact on the interaction and on plant health (for example, overexpression of phytoglobins in an inducible way, within specific tissues by using appropriate promoters).
Identification of targets of NO bioactivity during plant–fungal interactions would help to reveal the molecular mechanisms that underly NO functioning in these interactions.
Further studies are required that include plant species from diverse families in order to identify possible general patterns in NO regulation and potential family- or species-specific aspects of the plant responses and their impact on pathogenic and beneficial interactions.
Biotrophic fungi
In contrast to necrotrophic pathogens that feed on dead tissue and are thus not deterred by plant cell death, biotrophs require compounds from living host cells. Thus, HR-triggered cell death is probably one of the most important strategies in impeding the growth of biotrophic fungi (Govrin and Levine, 2000) and, accordingly, it is a likely hypothesis that NO-triggered HR would restrict the spread of biotrophic fungi. Indeed, Prats et al. (2005) found NO as one of the first responses of barley epidermal cells against Blumeria graminis. However, the role of NO in plant interactions with biotrophic fungal pathogens has not been thoroughly studied. It appears to have an important role in plant resistance to powdery mildew, as Schlicht and Kombrink (2013) found that Arabidopsis responded to both compatible (Golovinomyces orontii) and incompatible (Erysiphe pisi) interactions with powdery mildew with a rapid and transient accumulation of NO; however, there were significant differences in the patterns of NO accumulation. In leaves infected with G. orontii, the NO level rapidly declined after the initial burst and the authors suggested that this was most likely a consequence of an active effector-mediated defence suppression by the fungus. In contrast, NO levels remained high for an extended period of time during the incompatible interaction with E. pisi, indicating a correlation between the resistance phenotype and the amount and duration of NO production. Piterková et al. (2009) also found significant differences in the extent and timing of the increase in NO production triggered by Oidium neolycopersici between susceptible and resistant tomato genotypes. In the susceptible genotype, elevated NO production was observed only during the early moments following inoculation, whilst a two-phase increase in production was detected in the resistant genotypes. Similarly, a study by Qiao et al. (2015) suggested the importance of the intensity and duration of the NO burst in wheat immunity against the biotrophic fungus Puccinia triticina. In the incompatible plant–fungal interaction, a continuous and sustained increase in NO was found in the stomatal guard cells at the infection site. This burst primarily occurred in the cells undergoing a hypersensitive response. For the compatible interaction, a smaller and transient accumulation of NO was found. Taken together, these data suggest that the ability of the plant to rapidly and continuously increase NO production forms part of the molecular basis of plant resistance to biotrophic fungi.
Root fungal pathogens
The role of NO in plant interactions with root fungal pathogens has been relatively poorly studied, most likely because of the challenges involved in examining interactions below ground (Shelef et al., 2019). By using an in vitro system, we recently found that the compatible interaction of tomato with the necrotrophic pathogen F. oxysporum is associated with an early strong and transient burst of NO in tomato roots (Martínez-Medina et al., 2019a). This first burst is followed by a sustained and uncontrolled accumulation of NO that is concomitant with cell death. Moreover, as the infection progressed a down-regulation of Phytogb1 in infected tomato roots occurred, most likely in order to further increase NO levels and to promote cell death. By manipulating NO levels through a genetic approach, we were able to demonstrate the important role of NO in tomato susceptibility to F. oxysporum. Higher biomass of F. oxysporum and greater host cell death were observed in tomato lines displaying increased NO levels. By contrast, a decreased susceptibility to the pathogen was found in Phytogb1-overexpressing plants that displayed decreased NO levels. An increase in NO levels has also been found within the first hour after F. oxysporum infection of Arabidopsis roots (Gupta et al., 2014). Furthermore, Espinosa et al. (2014) found a strong increase in NO in roots of olive seedlings 1 h after contact with the necrotrophic fungus Verticillium dahliae. NO was spread across cell walls and in the cytoplasm of epidermal and cortical cells, and a concomitant increase in phenolic compounds was observed. Although the authors did not study the temporal dynamics of the NO burst and of the infection, they suggested that the burst was related to the activation of the plant immune response to the pathogen. Application of the NO-donor sodium nitroprusside (SNP) reduces the disease caused by Rhizoctonia solani in resistant and susceptible tomato cultivars via involvement of both the octadecanoid and phenylpropanoid pathways (Noorbakhsh and Taheri, 2016). These studies may suggest that, similar to the observations from above-ground plant parts, NO might play a dual role in root interactions with necrotrophic fungi. NO might act as a signal to initiate a defence response in incompatible interactions, while the NO signal might also be exploited by the pathogen to spread lesions in compatible interactions.
The rapid induction kinetics of the first NO burst and the lack of specificity of this early response during plant–fungal pathogenic interactions may indicate that NO accumulation is part of the plant response to fungal PAMPs. Indeed, the application of chitosan, a mycelial fungal elicitor of cell walls from F. oxysporum, triggers a rapid burst of NO (Wang and Wu, 2004; Srivastava et al., 2009; Martínez-Medina et al., 2019a). In accordance with this, we propose the following model. The interaction of the plant with necrotrophic pathogenic fungi results in a rapid and unspecific PAMP-triggered burst of NO that activates the plant response at the early stages. NO is massively produced after the first peak with the advance of the infection, and the associated cell death can be exploited by the pathogen to further expand lesions at later stages (Fig. 1A). In the case of plant interactions with biotrophic fungal pathogens, it seems that there is a correlation between the concentration and duration of the NO burst with plant resistance (Fig. 1B), although experimental data are scarce.
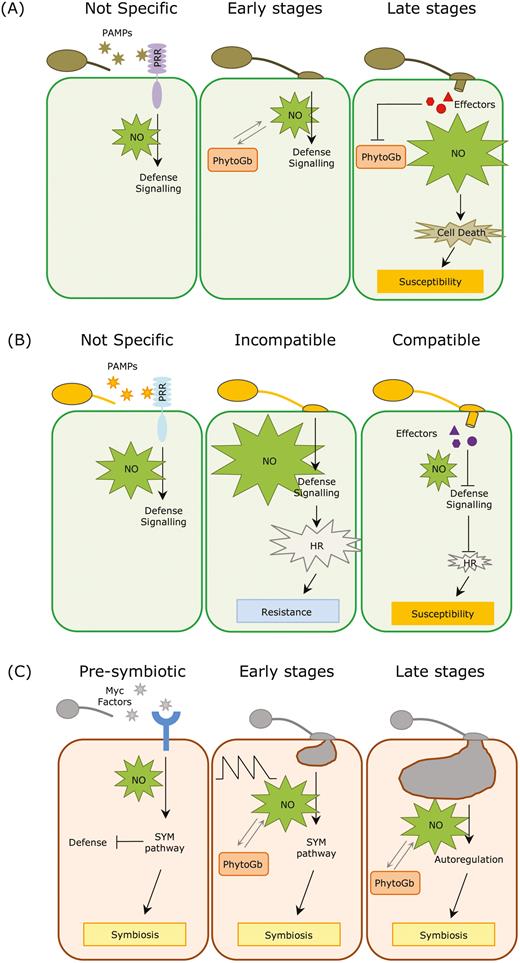
Model of NO functioning in plant–fungal interactions. (A) During interactions with necrotrophic fungi, the perception of fungal pathogen-associated molecular patterns (PAMPs) by plant pattern-recognition receptors (PRRs) triggers a rapid and unspecific burst of NO, which activates the plant response at the early stages. At later stages, NO is massively produced with the advance of the infection, and the associated cell death can be exploited by the pathogen to further expand the lesions (Floryszak-Wieczorek et al., 2007; Turrion-Gomez and Benito, 2011; Martínez-Medina et al., 2019a). (B) In interactions with biotrophic pathogens, plant perception of fungal PAMPs also triggers a rapid and unspecific burst of NO to activate the plant response. During incompatible interactions a second burst of NO leads to the hypersensitive response (HR) and cell death, which prevents the pathogen from spreading along the tissue as biotrophs only thrive in living cells. By contrast, in compatible interactions the NO level rapidly decreases after the initial burst, most likely due to active effector-mediated suppression of defences by the fungus, which leads to susceptibility (Piterková et al., 2009; Schlicht and Kombrink, 2013; Qiao et al., 2015). (C) During the pre-symbiotic stages of mycorrhizal symbiosis, Myc factors released by the fungus are perceived by plant receptors, triggering a burst of NO that is linked with the activation of the symbiotic regulatory (SYM) pathway. The activation of this pathway partially suppresses the host immune responses and prepares the plant for the forthcoming fungal colonization. After hyphal contact, the level of NO in the root cells spikes in a controlled manner thanks to the action of phytoglobins. This specific NO pattern may function as a regulatory element in the establishment of the symbiosis. In later stages, when the symbiosis is well established, NO is further controlled by the action of the phytoglobins, and is involved in the autoregulation of the symbiosis (Calcagno et al., 2012; Espinosa et al., 2014; Zou et al., 2017; Martínez-Medina et al., 2019a).
NO in plant–fungal mutualistic interactions
Interactions between plants and mutualistic fungi are ubiquitous and diverse, and often result in the improvement of plant growth and stress tolerance. In return, plants deliver carbohydrates and an ecological niche to their fungal associates, thus contributing to a stable association between the interacting partners (Zeilinger et al., 2016). Intimate mutualistic plant–fungal interactions include those between plants and foliar and root mutualistic endophytes, and mycorrhizal symbioses. The establishment and maintenance of intimate mutualistic interactions requires mutual recognition and substantial coordination of the plant and fungal responses. This coordination is based on finely regulated molecular dialogue between the partners, in which the host immune responses are tightly controlled to enable successful colonization and to maintain the balance of mutual benefits (Zipfel and Oldroyd, 2017; Plett and Martin, 2018). Given the crucial role of NO in plant immunity (as discussed above), it might be speculated that NO operates in the establishment and maintenance of mutualistic plant–fungal interactions. Remarkably, we could not find any reports related to NO signalling during plant interactions with fungal endophytes in leaves, despite their well-recognized benefits in plant health (Porras-Alfaro and Bayman, 2011). However, we found several studies on the specific roles of NO in endophyte-induced secondary metabolites in plants (Ren and Dai, 2013; Fan et al., 2014; Cui et al., 2017). The only reports regarding plant-produced NO during beneficial plant–fungal interactions concern root colonizers. A few recent studies report the occurrence of a burst of NO during the early steps of arbuscular mycorrhizal (AM) symbiosis and during the early interaction of roots with mutualistic fungal endophytes (Calcagno et al., 2012; Espinosa et al., 2014; Gupta et al., 2014; Zou et al., 2017; Martínez-Medina et al., 2019a). However, the specific role(s) of NO in plant–fungal mutualistic interactions remain unknown.
The first experimental data demonstrating the occurrence of a NO burst in mycorrhizal symbiosis were reported by Calcagno et al. (2012), who found that NO increased in the roots of Medicago truncatula within minutes following treatment with exudates of germinating spores of the AM fungus Gigaspora margarita. The authors suggested that this increase was mediated by the activity of nitrate reductase, and that was associated with the activation of the symbiotic regulatory (SYM) pathway. In agreement with these findings, we recently found a similar response in roots of tomato after treatment with exudates from germinating spores of the AM fungus Rhizoglomus irregularis (Martínez-Medina et al., 2019a). This response was specific for the AM fungus, as exudates from germinating spores of pathogenic F. oxysporum did not trigger NO accumulation. These findings indicate that the perception by the plant of bioactive molecules present in the exudates of germinating AM fungal spores triggers a NO-related response. It is notable that the chemical communication between the host plant and the AM fungus is initiated prior to physical contact between the symbionts (Buee et al., 2000; Chabaud et al., 2011). Plant perception of diffusible fungal signals (termed Myc factors) is translated into a transcriptional response that prepares the plant for the forthcoming fungal colonization (Maillet et al., 2011; Genre et al., 2013). It seems that NO is a component of the SYM pathway that is triggered in the host plants after the perception of Myc factors during the pre-symbiotic stage of the AM symbiosis.
Besides the pre-symbiotic stage, NO also accumulates in root cells shortly after contact with the mycelium of AM fungi. For example, NO increases in roots of olive seedlings (Espinosa et al., 2014) and tomato plants (Martínez-Medina et al., 2019a) within hours of contact with the mycelium of R. irregularis. Both sets of authors suggested that NO may function as a signalling component in regulating some key processes in the early stages of the AM interaction, such as cell wall remodelling, lateral root development, and controlling host defence. In addition, an increased NO level is observed in roots of seedlings of trifoliate orange (Citrus trifoliata) 21 d after inoculation with the AM fungus Diversispora versiformis (Zou et al., 2017), suggesting that NO might further function as a regulatory component in the maintenance of well-established AM symbioses (Fig. 1C). By manipulating the levels of NO in tomato roots using a genetic approach we have shown that NO appears to be a regulatory component of the establishment of AM symbiosis (Martínez-Medina et al., 2019a). Tomato roots displaying increased NO levels (through silencing of Phytogb1) or decreased NO levels (through the overexpression of Phytogb1) display increased mycorrhizal colonization, suggesting a role for NO in the tight regulation of the symbiosis.
Similar to mycorrhizal symbiosis, an increase of NO is observed in roots of Arabidopsis within minutes following contact with the mycelium of the mutualistic endosymbiotic fungus Trichoderma asperelloides (Gupta et al., 2014). The increase of NO is mediated by the activity of nitrate reductase, and is restricted to discrete root cells. Trichoderma harzianum also induced an early and transient burst of NO in tomato roots during the first hours after the interaction in parallel with the upregulation of PHYTOGB1, which was then maintained (Martínez-Medina et al., 2019b). These findings may suggest that NO is a common component of the plant signalling pathways that regulate the establishment of different plant–fungal mutualistic symbioses. It is notable that in the case of the Trichoderma symbiosis the increase in NO triggered by the fungus is limited to the early steps of the interaction (Gupta et al., 2014; Martínez-Medina et al., 2019b). This contrasts with the temporal organization displayed by NO accumulation during the AM interaction, in which NO levels peak in the host roots during the first days following contact with the AM fungal mycelia (Martínez-Medina et al., 2019a). These differences in the patterns of NO accumulation may highlight the different colonization strategies followed by these different mutualistic fungal symbionts. In the case of AM symbiosis, the plant actively accommodates the fungal partner in specialized host-membrane compartments in the root cortical cells to form arbuscules (Bonfante and Genre, 2010). This relies on continual signalling between the symbionts and in the activation of an extensive genetic and developmental program in both partners during the entire colonization process (MacLean et al., 2017). In contrast, the strategy followed by Trichoderma to colonize roots is mostly based on the early repression of plant immune responses to escape the plant defences (Brotman et al., 2013). These findings suggest that although NO is a common component of the plant signalling pathways that regulate the establishment of different plant–fungal mutualistic interactions, the patterns of NO and possibly its particular role(s) might be specific for every type of mutualistic association. However, experimental data on NO signalling during mutualistic plant–fungal interactions are still too scarce to develop accurate models.
Differential roles of NO in pathogenic and mutualistic plant–fungal interactions
As discussed above, it seems that NO is a common component of the plant signalling pathways that control both immunity against fungal pathogens and the establishment of symbioses with fungal mutualists. However, the spatio-temporal kinetics of NO accumulation in the two scenarios seem to differ widely. We found remarkable differences when comparing NO accumulation triggered in tomato roots by the AM fungus R. irregularis and triggered by the necrotrophic pathogen F. oxysporum (Martínez-Medina et al., 2019a). After an initial rapid and unspecific burst of NO, the pathogen triggered a massive accumulation of NO through the entire root, which was concomitant with a strong down-regulation of Phytogb1 and with the progression of cell death. In contrast, the AM mutualistic interaction triggered a series of more controlled oscillations of NO accumulation, which overlapped with the regulation of Phytogb1. In the case of the mutualistic association, the accumulation of NO was further restricted to the outer cell layers and root hairs. It is notable that these specific root zones are associated with Ca2+ signalling during early stages of the mycorrhizal process (Genre et al., 2013), perhaps suggesting an interplay between Ca2+ and NO in the onset of the AM symbiosis. Similarly, Espinosa et al. (2014) found that R. irregularis triggers a controlled burst of NO that is localized in the external cell layers. By contrast, the NO burst triggered by the pathogen V. dahliae is stronger and spreads not only to external cell layers, but also to cortical cells. A similar pattern has been observed when comparing NO accumulation triggered by T. asperelloides and F. oxysporum in Arabidopsis roots (Gupta et al., 2014): while the accumulation of NO that is triggered during the mutualistic interaction is weak and restricted to discrete root cells, accumulation triggered by the pathogen is stronger and is spread over wide portions of the roots. Thus, it seems that although NO-related signalling is a common regulatory component in mutualistic and pathogenic plant–fungal interactions, the NO-related signature that is triggered and probably also the specific NO functions differ widely. We envisage that future studies that compare pathogenic and mutualistic interactions within the same plant system will allow the specific regulatory role(s) of NO to be deciphered.
Concluding remarks
The information currently available on NO regulation during plant–fungal interactions allows to conclude that it is a key signal in the establishment and the fine-tuning of both mutualistic and pathogenic interactions. Although NO production is a common feature of both, the signature that is triggered seems to differ quantitatively and in its spatio-temporal distribution in the two types of interactions. These differences most likely determine the specific NO functions that can shape the final outcome of the interaction. Based on our current knowledge, we propose a model for NO regulation and function in the different types of interactions (Fig. 1), but this identifies important gaps in the available information. Comparative studies among different mutualistic and pathogenic interactions, using similar methodologies and across multiple plant systems are required in order to identify common patterns and major regulatory nodes. Moreover, studies devoted to examining the role of NO as a cue in the plant defence signalling network are required to explore the specific functions of NO in mutualistic and pathogenic interactions. This review highlights the importance of the spatio-temporal dynamics in NO production, and the need of precise and sensitive methods to measure it and to determine its sources and metabolism. Thus, important technical challenges remain ahead, as described in Box 1, but carefully designed new experiments together with the technical progress already taking place should result in great advances being made in the coming years. Such research will boost our knowledge of NO functions and the regulation of plant–fungal interactions, and potentially lead to biotechnological applications for plant health in agricultural systems.
Acknowledgements
We apologize to any colleagues whose studies have not been cited due to space limitations. This research was supported by grants EX12-BIO296 (from the Junta de Andalucía), and PGC2018-098372 and RTI2018-094350-B-C31 (from the Spanish Ministry of Science, Innovation and University). LP-A and LT-C were supported by University Staff Training (FPU) fellowships from the Spanish Ministry of Education, Culture and Sports. AMM further acknowledges funding from the program for attracting talent to Salamanca from the Fundación Salamanca Ciudad de Cultura y Saberes.
Comments