-
PDF
- Split View
-
Views
-
Cite
Cite
Hong Zhang, Yanpei Liu, Feng Wen, Dongmei Yao, Lu Wang, Jin Guo, Lan Ni, Aying Zhang, Mingpu Tan, Mingyi Jiang, A novel rice C2H2-type zinc finger protein, ZFP36, is a key player involved in abscisic acid-induced antioxidant defence and oxidative stress tolerance in rice, Journal of Experimental Botany, Volume 65, Issue 20, November 2014, Pages 5795–5809, https://doi.org/10.1093/jxb/eru313
- Share Icon Share
Abstract
C2H2-type zinc finger proteins (ZFPs) have been shown to play important roles in the responses of plants to oxidative and abiotic stresses, and different members of this family might have different roles during stresses. Here a novel abscisic acid (ABA)- and hydrogen peroxide (H2O2)-responsive C2H2-type ZFP gene, ZFP36, is identified in rice. The analyses of ZFP36-overexpressing and silenced transgenic rice plants showed that ZFP36 is involved in ABA-induced up-regulation of the expression and the activities of superoxide dismutase (SOD) and ascorbate peroxidase (APX). Overexpression of ZFP36 in rice plants was found to elevate the activities of antioxidant enzymes and to enhance the tolerance of rice plants to water stress and oxidative stress. In contrast, an RNA interference (RNAi) mutant of ZFP36 had lower activities of antioxidant enzymes and was more sensitive to water stress and oxidative stress. ABA-induced H2O2 production and ABA-activated mitogen-activated protein kinases (MAPKs) were shown to regulate the expression of ZFP36 in ABA signalling. On the other hand, ZFP36 also regulated the expression of NADPH oxidase genes, the production of H2O2, and the expression of OsMPK genes in ABA signalling. These results indicate that ZFP36 is required for ABA-induced antioxidant defence, for the tolerance of rice plants to water stress and oxidative stress, and for the regulation of the cross-talk between NADPH oxidase, H2O2, and MAPK in ABA signalling.
Introduction
Water stress, including drought and salinity, is one of the most important environmental factors that adversely affect plant growth and crop production. Plant adaptation to water stress is dependent on the activation of cascades of molecular networks involved in stress perception, signal transduction, and the expression of specific stress-related genes and metabolites (Huang et al., 2012). The plant hormone abscisic acid (ABA), accumulated in plant cells exposed to water stress, is the central regulator of water stress resistance in plants, and coordinates a complex regulatory network enabling plants to cope with water stress conditions (Cutler et al., 2010; Hubbard et al., 2010; Umezawa et al., 2010; Fujita et al., 2011). One mode of ABA-enhanced water stress tolerance is associated with the induction of antioxidant defence systems, including enzymatic and non-enzymatic constituents, which protect plant cells against oxidative damage. In ABA signal transduction, several signal molecules such as calcium ion (Ca2+), reactive oxygen species (ROS), and nitric oxide (NO), and protein kinases such as mitogen-activated protein kinase (MAPK), calcium-dependent protein kinase (CDPK), and calcium/calmodulin-dependent protein kinase (CCaMK) have been shown to play important roles in the regulation of antioxidant defence systems (Jiang and Zhang, 2001, 2002a, b, 2003; Zhang et al., 2006, 2007; Neill et al., 2008; Xing et al., 2008; Ye et al., 2011; Ma et al., 2012; Shi et al., 2012, 2014; Ding et al., 2013). However, the mechanisms by which these components regulate antioxidant defence in ABA signalling remain to be determined.
Transcriptional modulation is thought to be one of the most important ways in which plants respond and adapt to stress conditions (Yamaguchi-Shinozaki and Shinozaki, 2006; Nakashima et al. 2009; Kodaira et al., 2011). A number of genes have been reported to be induced or repressed in plants under water stress conditions (Yamaguchi-Shinozaki and Shinozaki, 2006; Nakashima et al. 2009; Golldack et al., 2011; Lindemose et al., 2013). Various transcription factors have been shown to be involved in ABA and water stress responses, such as those from the APETALA2/ethylene-responsive element-binding factor (AP2/ERF), NAM/ATAF1/CUC2 (NAC), WRKY, MYB, Cys2(C2)His2(H2)-type zinc finger protein (ZFP), basic helix–loop–helix (bHLH), and basic leucine zipper (bZIP) families (Yamaguchi- Shinozaki and Shinozaki, 2006; Nakashima et al. 2009; Fujita et al., 2011; Golldack et al., 2011; Lindemose et al., 2013). These transcription factors function as transcriptional activators or repressors and control downstream gene expression in ABA and stress signal transduction pathways.
The Cys2/His2 (C2H2)-type zinc finger proteins (ZFPs), with 176 members in Arabidopsis and 189 members in rice, constitute one of the largest families of transcriptional regulators in plants (Agarwal et al., 2007; Ciftci-Yilmaz and Mittler, 2008). It has been shown that C2H2-type ZFPs are important components in the regulation of plant growth, development, hormone responses, and tolerance to biotic and abiotic stresses (reviewed in Ciftci-Yilmaz and Mittler, 2008; Miller et al., 2008; Kielbowicz-Matuk, 2012). In Arabidopsis, DNA chip analysis revealed that the transcript levels of several members of the C1-2i subclass (Zat family) in C2H2-type ZFPs were up-regulated by various biotic and abiotic stresses (Mittler et al., 2006; Ciftci-Yilmaz and Mittler, 2008). Zat10 (STZ) and Zat12, two widely studied members of the Zat family, have been shown to be induced by drought, salinity, osmotic stress, temperature stress, wounding, oxidative stress, and high-light stress (Rizhsky et al., 2004; Sakamoto et al., 2004; Davletova et al., 2005a, b; Vogel et al., 2005; Mittler et al., 2006; Koussevitzky et al., 2007; Rossel et al., 2007; Ciftci-Yilmaz and Mittler, 2008; Miller et al., 2008; Kielbowicz-Matuk, 2012). Genetic analysis revealed that Zat10 and Zat12 are required for the expression of ROS-scavenging genes and tolerance to drought, salinity, and oxidative stress (Rizhsky et al., 2004; Sakamoto et al., 2004; Mittler et al., 2006; Rossel et al., 2007; Ciftci-Yilmaz and Mittler, 2008; Miller et al., 2008). In rice, several members of the C2H2-type ZFPs, such as ZFP182, ZFP245, ZFP252, and ZFP179, have also been shown to be involved in the responses of rice to drought, salinity, and oxidative stress (J. Huang et al., 2007, 2009, 2012; Xu et al., 2008; Sun et al., 2010). These results suggest that some members of the C2H2-type ZFPs are important regulators of ROS signalling under abiotic stresses. Until recently, however, it has not been clear whether these members of C2H2-type ZFPs are involved in ABA-induced antioxidant defence. By screening the homologous genes of Zat12 in rice, an ABA- and H2O2-responsive ZFP gene, ZFP182, was identified in rice and was shown to be involved in ABA-induced antioxidant defence (Zhang et al., 2012). In this study, by screening the homologous genes of Zat10 in rice, a novel ABA- and H2O2-responsive C2H2-type ZFP gene, ZFP36, was identified in rice. By combining pharmacological, biochemical, and molecular biology analyses with genetic approaches, evidence is provided to show that ZFP36 plays a key role in ABA-induced antioxidant defence and tolerance of rice to water stress and oxidative stress. Moreover, it is revealed that ZFP36 is an important regulator of the cross-talk involving NADPH oxidase, H2O2, and MAPK in ABA signalling.
Materials and methods
Plant material and treatments
Seeds of rice (Oryza sativa L. sub. japonica cv. Nipponbare) were grown hydroponically with a nutrient solution in a light chamber at a temperature of 22 °C (night) to 28 °C (day), photosynthetic active radiation of 200 μmol m–2 s–1, and a photoperiod of 14/10h (day/night). When the second leaves were fully expanded, they were collected and used for investigations.
The plants were placed in beakers wrapped with aluminium foil with nutrient solution containing 100 μM ABA or 10mM H2O2 for the indicated time, with a continuous light intensity of 200 μmol m–2 s–1. To study the effects of inhibitors, the plants were pre-treated with 100 μM diphenylene iodonium (DPI) and 5mM dimethylthiourea (DMTU) for 2h, and then exposed to 100 μM ABA treatment under the same conditions as described above. Seedlings were treated with the nutrient solution alone under the same conditions for the whole period and served as controls for the above. After treatments of rice plants, the leaves were sampled and immediately frozen under liquid N2 for further analysis.
Isolation of total RNA and semi-quantitative reverse transcription–polymerase chain reaction (RT–PCR)
Total RNA was isolated from leaves using RNAiso Reagent (TaKaRa, China) according to the manufacturer’s instructions. DNase treatment was included in the isolation step using RNase-free DNase (TaKaRa, China). Approximately 2 μg of total RNA were reverse transcribed using oligo d(T)18 primer and M-MLV reverse transcriptase (TaKaRa, China) at 42 °C for 90min and 75 °C for 15min. cDNA was amplified by PCR using the following primers: ZFP36, forward TTTTACGACGACGGCAACGG and reverse AGCGGCATCAGGTTGAGGTC; OsMPK1, forward AGAT ACATTCGCCAACTTCC and reverse TCTTAGAACAACACC TTCAGC; OsMPK4, forward CGCACAAACAACACTAAAGG and reverse GAGGTCCACAGGAAGAACAA; OsMPK5, forward GGGATCGTCTGCTCCGTGATGA and reverse AAGATGCAG CCGACGGACCA; OsMPK7, forward GGTGACTCCAGCCGA TA and reverse AGATACCTCCTTGCCTTGT; OsMPK14, forward TTGCTCTGCTTTGGACACTC and reverse CGTCTTGCC TTCTCATTTCTAA; and GAPDH, forward ACCACAAA CTGCCTTGCTCC and reverse ATGCTCGACCTGCTGTCACC. To standardize the results, the relative abundance of the glyceraldehyde-3-phosphate dehydrogenase gene (GAPDH) was also determined and used as the internal standard.
The cycle number of the PCRs was adjusted for each gene to obtain barely visible bands in agarose gels. Aliquots of the PCRs were loaded on agarose gels and stained with ethidium bromide.
Cloning of ZFP36
According to the predicted sequence of ZFP36, two primers (forward 5′-CAATCCCATCAAATCATCCACCGC-3′ and reverse 5′-CACCAGCTTAGCTTGTTGCATACTCG-3′) were designed. The PCR conditions are as follows: 0.8 μl of reverse transcription product was amplified in a 20 μl volume containing 10 μl of 2×Taq Master Mix (Biodee, Nanjing, China), 0.4 μl of 10mM of each primer, and 8.4 μl of double-distilled water. PCR was performed on a DNA amplification machine (Dongsheng, China) for an initial denaturation at 94 °C for 5min; 35 cycles at 94 °C for 20 s, 58 °C for 20 s, 72 °C for 30 s; and a final step of 72 °C for 10min. The PCR products were run on an 1% agarose gel and purified with a Gel Extraction Kit (Generay, Shanghai, China) according to the manufacturer’s protocol. The purified product was then cloned into the pMD19-T vector (TaKaRa, China) and sequenced (BGI, Shenzhen, China).
Real-time quantitative RT–PCR expression analysis
Real-time quantitative RT–PCRs were performed on a Bio-RAD MyiQ™ Real-time PCR Detection System (Bio-Rad, USA) using the SYBR® Premix Ex Taq™ (TaKaRa, China) according to the manufacturer’s instructions. cDNA was amplified by PCR using the following primers: ZFP36, forward AACTAATTCATCATACGCCATC and reverse CAAAGAATAGACTCTGTTCAATAG; OsMPK1, forward CTGAAGGTGTTGTTCTAAGAGTAG and reverse TGATGAT AACCGCAGAATTTAGG; OsMPK4, forward AACCAAGGG AAGCATTACTAC and reverse GAGCAAACTATTCCATAAGCC; OsMPK5, forward CCGCTGCAGAGAATCACGTTG and reverse TCCTCGTTTAGAGCCTTCTGCTC; OsMPK7, forward TGTTT CTCTTCCAAGGGCTA and reverse ATCGGATTCCT CACCACC; OsMPK14, forward TGCTTTGGACACTCACACCG and reverse CCCCTTGATGGAGGAAGTAGAATA; OsrbohB, forward TGCTCTTTGTCCATGGAACGTG and reverse ACAG CGAGGTACATCCATGTCG; OsrbohE, forward TGGTCTTGG AATTGGTGCTACTCC and reverse ACCATGTATGCTTT CCACCTCTTC; SodCc2, forward GGAGAAGATGGTGTTGCTA and reverse GCCTTGAAGTCCGATGAT; cAPX, forward TGTCC TTGTCACTCAAACCCATC and reverse GACCAACTTCCCAT CCTCTCCTA; anbd Osactin, forward CTTCATAGGAAT GGAAGCTGCGGGTA and reverse CGACCACCTTGATCTT CATGCTGCTA. Each PCR (20 μl) contained 10 μl of 2× real-time PCR Mix (containing SYBR Green I), 0.4 μl of each primer, and appropriately diluted cDNA. The thermal cycling conditions were 95 °C for 30 s followed by 40 cycles of 95 °C for 10 s, 60 °C for 20 s, and 72 °C for 20 s. To standardize the data, the ratio of the absolute transcript level of the target genes to the absolute transcript level of Osactin was calculated for each sample. The relative expression levels of the target genes were calculated as x-fold changes relative to the appropriate control experiment for the different treatments.
Protoplast isolation
Rice plants were grown in the dark at 28 °C for 1–2 weeks. When plants were ~4–8 inches tall, the protoplasts from the leaf and stem tissue were isolated according to the method described by Zhang et al. (2012).
Double-stranded (ds) RNA synthesis and transfection
dsRNA was produced by in vitro transcription of a PCR-generated DNA template using the following primer pairs containing the T7 promoter sequence on both ends: dsZFP36, forward TAATACGACTCACTATAGGGAGACTA ATTCATCATACGCCATC and reverse TAATACGACTCACTATA GGGAGATGAATCAACACTCCTAGAACC (the underlined part indicates the T7 promoter sequence); dsMPK5, forward TAATA CGACTCACTATAGGGAGACCGCTGCAGAGAATCA CAGTTG and reverse TAATACGACTCACTATAGGGA GATCCTCGTTTAGAGCCTTCTGCTC; dsMPK1, forward TAA TACGACTCACTATAGGGAGAAGATACATTCGC CAACTTCC and reverse TAATACGACTCACTATAGGGA GATCTTAGAACAACACCTTCAGC; dsMPK4, forward TAATACGACTCACTATAGGGCCGCAAGCACATCCTCTT and reverse TAATACGACTCACTATAGGGCCTGCCACAT CATCTCCC; dsMPK7, forward TAATACGACTCACTA TAGGGTACGGTCTTCACAATACTACTT and reverse TAAT ACGACTCACTATAGGGATTCCCATCTTGCTCATC; and dsMPK14, forward TAATACGACTCACTATAGGGTAC GGTGAGGGAAACAGGT and reverse TAATACGACTCACTA TAGGGGTCGCAGGAGTCTAAGCAA. The PCR products were recovered and the concentrations were measured. dsRNAs were synthesized in vitro using the Promega Ribomax Large Scale RNA Production System T7 (Promega). The dsRNAs were purified by phenol–chloroform–isopropanol extraction, dissolved in RNase-free water, and quantified by UV spectrophotometry.
The dsRNAs were delivered into protoplasts using a polyethylene glycol (PEG)–calcium-mediated method described previously (Shi et al., 2012).
Generation of ZFP36 transgenic rice
To obtain the transgenic plants with overexpression of ZFP36, the full-length open reading frame (ORF) of ZFP36 was inserted into the plant binary vector pCAMBIA1301 to construct pCAMBIA1301-ZFP36. Then the ZFP36 gene under the control of the Cauliflower mosaic virus (CaMV) 35S promoter was transformed into rice (O. sativa sub. japonica cv. Nipponbare) by the Agrobacterium-mediated transformation method (Hiei et al., 1994).
To generate ZFP36-RNAi (RNA interference) plants, the transcription region of the ZFP36 gene (from +116bp to +366bp) was amplified with primers containing the following restriction enzyme sites: the 5′ most primer with NcoI and BamHI sites, and the 3′-most primer with XhoI and SpeI sites. The resulting PCR product was digested first with NcoI and XhoI and ligated into a NcoI–XhoI-cleaved pGSA1285 vector (template plasmid). For the second PCR fragment for the inverted repeat construct, the same PCR product was digested with BamHI and SpeI and inserted into the BamHI–SpeI sites of the template plasmid. The ZFP36-RNAi plasmid was introduced into rice (O. sativa sub. japonica cv. Nipponbare) by the Agrobacterium-mediated transformation method (Hiei et al., 1994).
Tolerance of transgenic rice plants to PEG and H2O2 stresses
To evaluate the performance of the transgenic rice plants under abiotic stresses, the seeds of T2 transgenic lines (ZFP36-OE and ZFP36-RNAi) and the wild type (WT) were germinated and grown in a light chamber as described above. For the analysis of survival rate, the rice seedlings were treated with 18% PEG 4000, 100mM H2O2 for the indicated time, and the survival rates of the rice plants were counted after recovery by re-watering for 14 d. For the analysis of growth, the rice seedlings were treated with 18% PEG 4000, 100mM H2O2 for 15 d, and the shoot length and fresh weight, and root length were measured. For the analysis of oxidative damage to lipids and plasma membranes, the rice seedlings were treated with 15% PEG 4000, 100mM H2O2 for 2 d, and the content of malondialdehyde (MDA) and the percentage leakage of electrolyte were determined. For the analysis of antioxidant enzymes, the rice seedlings were treated with 15% PEG 4000, 100mM H2O2 for 12h, and the activities of superoxide dismutase (SOD) and ascorbate peroxidase (APX) were measured.
Determination of lipid peroxidation and electrolyte leakage
Oxidative damage to lipids was estimated by measuring the content of MDA in leaf segment homogenates, prepared in 10% trichloroacetic acid containing 0.65% 2-thiobarbituric acid (TBA), and heated at 95 °C for 25min, as in Hodges et al. (1999). The percentage leakage of electrolyte was determined as described by Jiang and Zhang (2001).
Enzyme assays
Frozen protoplasts or leaves were homogenized in a solution of 50mM potassium phosphate buffer (pH 7.0) containing 1mM EDTA and 1% polyvinylpyrrolidone. The homogenate was centrifuged at 12 000 g for 20min at 4 °C and the supernatant was immediately used for the antioxidant enzyme assays. The total activities of SOD and APX were determined as described previously (Jiang and Zhang, 2001).
H2O2 detection by confocal laser scanning microscopy
H2O2 production in protoplasts was monitored using the H2O2-sensitive fluorescent probe 2′,7′-dichlorofluorescein diacetate (H2DCF-DA; Molecular Probes, Leiden, The Netherlands) using the method described by Bright et al. (2006). The specificity of the H2O2-mediated fluorescence was proved by the application of catalase (CAT) (Ding et al., 2013). Images acquired were analysed using Leica IMAGE software (Leica Microsystems, Heerbrugge, Switzerland). Data are presented as mean pixel intensities. A total of 120 protoplasts are observed per treatment for three independent replicates.
Determination of H2O2 content in leaf extracts
H2O2 from leaves was extracted according to a previously described method (Rao et al., 2000). The content of H2O2 in leaf extracts was determined using the Hydrogen Peroxide Assay Kit (Beyotime Institute of Biotechnology, Shanghai, China) according to the manufacturer’s instructions. Briefly, test tubes containing 50 μl of supernatants and 100 μl of test solutions were placed at 30 °C for 30min and measured immediately with a spectrometer at a wavelength of 560nm. Absorbance values were calibrated to a standard curve generated with known concentrations of H2O2.
Results
Cloning and sequence analysis of ZFP36
The ZFP36 gene containing a complete ORF of 663bp was cloned by RT–PCR from total RNA prepared from rice seedlings. The predicted protein product of ZFP36 comprises 220 amino acids with the calculated molecular mass of 22.804kDa. The ZFP36 protein contains two C2H2-type zinc fingers, with a plant-specific QALGGH motif in each zinc finger domain (Fig. 1A). A homology search against the GenBank database showed that ZFP36 was homologous to many C2H2-type ZFPs in plants, especially in the finger domains (Fig. 1A). Like most reported C2H2-type ZFPs, ZFP36 contains a DLN-box/EAR-motif with a consensus of DLN at the C-terminus and a putative nuclear localization signal (NLS) (Fig. 1A). Unlike most zinc finger proteins reported, however, the NLS of ZFP36 locates at the C-terminus.
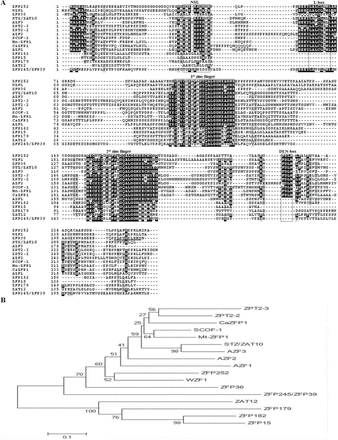
Alignment and phylogenetic relationship of ZFP36 with the other stress-responsive C2H2-type ZFPs. (A) Alignment of ZFP36 with other plant stress-responsive C2H2-type ZFPs. Characteristic amino acid sequences (NLS, L-box, two zinc fingers, and DLN-box) are boxed, and the putative NLS is underlined. Positions containing identical residues are shaded in black, while conservative residues are in grey. (B) The phylogenetic tree of plant stress-responsive C2H2-type ZFPs. Alignment of conserved ZFP sequences and phylogenetic analysis were performed by the program ClustalX 1.83 and MEGA 4, respectively. A distance tree was calculated using the Neighbor–Joining method. The lengths of the branches are proportional to the degree of divergence. Species designations and corresponding GenBank accession numbers are as follows: A. thaliana: AZF1 (BAA85108), AZF2 (BAB02542), AZF3 (AB030732), ZAT12 (AAM65582), STZ/ZAT10 (NP-174094); C. annuum: CaZFP1 (CAF74935); G. max: SCOF-1 (AAB39638); M. truncatula: Mt-ZFP1 (CAB77055); O. sativa: ZFP15 (AAP42460), ZFP36 (AAP51130.1), ZFP179 (AAL76091.1), ZFP182 (NP001051718.1), ZFP245 (AAQ95583), ZFP252 (AAO46041.1); P. hybrida: ZPT2-3 (BAA05079), ZPT2-2 (BAA05077.1); T. aestivum: WZF1 (BAA03902).
To investigate the evolutionary relationship among plant C2H2-type ZFPs involved in stress responses, a phylogenetic tree was constructed using the Neighbor–Joining method with the full-length amino acid sequences (Fig. 1B). The result revealed that ZFP36 was clustered with Arabidopsis Zat10.
ABA and H2O2 induce the expression of ZFP36, and H2O2 is required for the ABA-induced gene expression
To investigate the effects of ABA and H2O2 on the expression of ZFP36 in leaves of rice seedlings, relative quantitative real-time PCR analysis was performed on total RNA isolated from rice leaves treated with ABA or H2O2. The results showed that the treatments with ABA and H2O2 induced a biphasic response in the expression of ZFP36 in rice leaves (Fig. 2A, B). For ABA treatment, the first peak (phase I) in the expression of ZFP36 occurred within 20min of ABA treatment, and the second peak (phase II) appeared after 4h of ABA treatment (Fig. 2A). For H2O2 treatment, phase I occurred within 10min of H2O2 treatment and phase II peaked after 6h of H2O2 treatment (Fig. 2B).
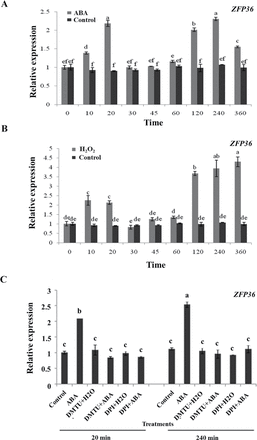
ABA and H2O2 induce the expression of ZFP36 in rice leaves. (A) Expression analysis of ZFP36 in leaves of rice plants exposed to ABA treatment. (B) Expression analysis of ZFP36 in leaves of rice plants exposed to H2O2 treatment. (C) Effects of pre-treatments with dimethylthiourea (DMTU) and diphenylene iodonium (DPI) on the expression of ZFP36 in rice leaves exposed to ABA treatment. In (A, B), the rice seedlings were treated with 100 μM ABA (A) or 10mM H2O2 (B) for various times as indicated. In (C), the rice seedlings were pre-treated with 5mM DMTU or 100 μM DPI for 2h, and then exposed to 100 μM ABA for 20min and 240min, respectively. Relative expression levels of the ZFP36 gene were analysed by real-time quantitative RT–PCR. Values are means ±SE of three independent experiments. Means denoted by the same letter did not differ significantly at P<0.05 according to Duncan’s multiple range test.
To establish a link between the production of H2O2 and the expression of ZFP36 in ABA signalling, rice plants were pre-treated with the two ROS manipulators, DMTU, a scavenger for H2O2, and DPI, an inhibitor of NADPH oxidase, respectively, and then exposed to ABA treatment. Experimental results showed that pre-treatments with the two H2O2 manipulators dramatically abolished the phase I and phase II induced by ABA (Fig. 2C), suggesting that H2O2 is required for ABA-induced up-regulation in the expression of ZFP36.
ZFP36 is involved in ABA-induced antioxidant defence and enhances the tolerance of rice to water stress and oxidative stress
To investigate whether ZFP36 is involved in ABA-induced antioxidant defence, ZFP36-overexpressing (ZFP36-OE) and silenced (ZFP36-RNAi) transgenic rice plants were generated. As shown in Fig. 3A, the expression of ZFP36 in ZFP36-OE plants was significantly higher than in WT plants, and the expression of ZFP36 in ZFP36-RNAi plants was obviously lower than that in WT plants. Under the control conditions, the expression of the antixiodant genes SodCc2, encoding a cytosolic Cu/Zn-SOD, and cAPX, encoding a cytosolic APX, and the activities of SOD and APX were higher in the leaves of the ZFP36-OE plants than in those in the WT plants, and the expression and the activities of these antioxidant enzymes were lower in the ZFP36-RNAi plants than in the WT plants (Fig. 3B, C). ABA treatment induced significant increases in the expression of SodCc2 and cAPX and the activities of SOD and APX in leaves of WT plants, and the increases induced by ABA were further enhanced in the leaves of the ZFP36-OE plants, but were inhibited in the ZFP36-RNAi plants (Fig. 3B, C). These results indicate that ZFP36 is required for ABA-induced increases in the expression and the activities of SOD and APX.
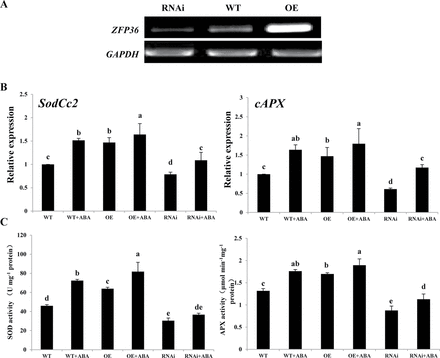
ZFP36 is required for ABA-induced up-regulation of the expression and the activities of antioxidant enzymes in rice leaves. (A) The expression of ZFP36 analysed by RT–PCR in ZFP36-OE plants, ZFP36-RNAi plantsm and WT plants grown under control conditions. The glyceraldehyde-3-phosphate dehydrogenase gene (GAPDH) was amplified as a control for the amount of template. (B, C) The expression of SodCc2 and cAPX (B) and the activities of SOD and APX (C) in the leaves of ZFP36-OE plants, ZFP36-RNAi plants, and WT plants. The rice seedlings were treated with 100 μM ABA for 12h, and the relative expression levels of SodCc2 and cAPX and the activities of SOD and APX were analysed. In (A), experiments were repeated at least three times with similar results. In (B, C), values are means ±SE of three independent experiments. Means denoted by the same letter did not differ significantly at P<0.05 according to Duncan’s multiple range test.
To test the role of ZFP36 in the tolerance of water stress and oxidative stress in plants, the rice seedlings of the WT, ZFP36-OE, and ZFP36-RNAi were treated with PEG (simulation of water stress) and H2O2. Under the control conditions, there were no significant differences in plant height (Fig. 4A, C), shoot fresh weight (Fig. 4D), and root length (Fig. 4E) between the ZFP36-OE line or ZFP36-RNAi line and WT plants. Under water stress induced by 18% PEG 4000 and oxidative stress induced by 100mM H2O2, however, the ZFP36-OE plants had longer shoot and root lengths, greater fresh weight, and higher survival rate than WT plants (Fig. 4A–E). In contrast, the ZFP36-RNAi plants exhibited shorter shoot and root lengths, lower fresh weight, and lower survival rate than WT plants under the stressed conditions (Fig. 4A–E). These results indicate that ZFP36 is required for the tolerance of rice to water stress and oxidative stress.
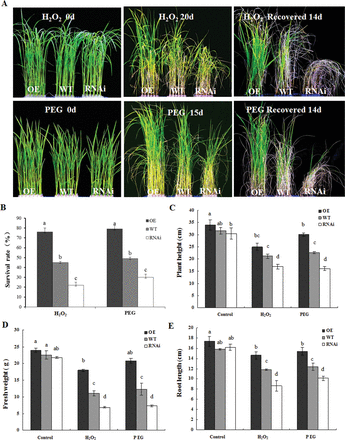
ZFP36 enhances the tolerance of rice plants to water stress and oxidative stress. (A) Photographs of ZFP36-OE plants, ZFP36-RNAi plants, and WT plants grown under water stress and oxidative stress. Ten-day-old rice seedlings were treated with 18% PEG 4000 for 15 d or 2-week-old plants were treated with 100mM H2O2 for 20 d, and then left to recover for 14 d. (B) The survival rate (%) of the rice plants after recovery by re-watering for 14 d shown in (A). (C–E) The growth analysis of ZFP36-OE plants, ZFP36-RNAi plants, and WT plants under water stress and oxidative stress. Ten-day-old rice seedlings were treated with 18% PEG 4000, 100mM H2O2 for 15 d, and the lengths of shoots (C) and roots (E) and shoot fresh weight (D) were measured. In (A), experiments were repeated at least three times with similar results. In (B–E), values are means ±SE of three independent experiments. Means denoted by the same letter did not differ significantly at P<0.05 according to Duncan’s multiple range test.
To investigate the role of ZFP36 in antioxidant defence under stressed conditions, the content of MDA and the percentage of electrolyte leakage, which are indications of oxidative stress, and the activities of the antioxidant enzymes SOD and APX were analysed in gain- and loss-of-fuction ZFP36 rice plants exposed to water stress and oxidative stress. The content of MDA (Fig. 5A) and the percentage of electrolyte leakage (Fig. 5B) were lower in the leaves of the ZFP36-OE plants exposed to 15% PEG and 100mM H2O2 treatment than in those of the WT plants, and the activities of SOD (Fig. 5C) and APX (Fig. 5D) in the ZFP36-OE plants were higher than the WT plants under the stressed conditions. In contrast, under water stress and oxidative stress, the content of MDA (Fig. 5A) and the percentage of electrolyte leakage (Fig. 5B) were higher in the leaves of the ZFP36-RNAi plants than in those of the WT plants, and the activities of SOD (Fig. 5C) and APX (Fig. 5D) in the ZFP36-RNAi plants were lower than in the WT plants. These results suggest that the expression of ZFP36 can enhance the ability of rice plants to scavenge ROS, thus resulting in the reduction of oxidative damage caused by water stress and oxidative stress.
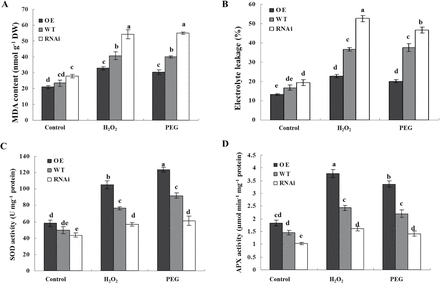
ZFP36 up-regulates the activities of antioxidant enzymes and reduces oxidative damage under water stress and oxidative stress. (A, B) The content of MDA (A) and the percentage leakage of electrolyte (B) in the leaves of ZFP36-OE plants, ZFP36-RNAi plants, and WT plants. Ten-day-old rice seedlings were treated with 15% PEG 4000, 100mM H2O2 for 2 d, and then leaves were sampled for the determination of MDA content and electrolyte leakage (%).(C, D) The activities of SOD (C) and APX (D) in the leaves of ZFP36-OE plants, ZFP36-RNAi plants, and WT plants. Ten-day-old rice seedlings were treated with 15% PEG 4000, 100mM H2O2 for 12h, and then leaves were sampled for the determination of the activities of SOD and APX. Values are means ±SE of three independent experiments. Means denoted by the same letter did not differ significantly at P<0.05 according to Duncan’s multiple range test.
ZFP36 regulates the expression of NADPH oxidase and MAPK genes and the production of H2O2 in ABA signalling
Previous studies have shown that NADPH oxidase, H2O2, and MAPK are important components in ABA-induced antioxidant defence and that there is a positive feedback loop involving NADPH oxidase, H2O2, and MAPK in ABA signalling (Zhang et al., 2006; Lin et al., 2009). To investigate whether ZFP36 regulates the expression of NADPH oxidase genes and the production of H2O2 in ABA signalling, ZFP36-OE and ZFP36-RNAi transgenic rice plants and WT plants were used. In the rice genome, there are nine NADPH oxidase (rboh) genes (OsrbohA–OsrbohI; Wong et al., 2007). ABA treatment only induced increased expression of OsrbohB and OsrbohE in leaves of rice plants (Fig. 6A; data not shown). Under the control conditions, the expression of OsrbohB and OsrbohE was higher in the leaves of the ZFP36-OE plants than that in the WT plants, and the expression of these genes was lower in the ZFP36-RNAi plants than in the WT (Fig. 6B). ABA treatment induced significant increases in the expression of OsrbohB and OsrbohE in leaves of WT plants, and the increases were further enhanced in the leaves of the ZFP36-OE plants, but were inhibited in the ZFP36-RNAi plants (Fig. 6B). The changes in the production of H2O2, detected by confocal laser scanning microscopy in rice protoplasts (Fig. 6C) and by spectrophotometry in leaf extracts (Fig. 6D), in the transgenic rice plants and WT plants with or without ABA were similar to the changes in the expression of OsrbohB and OsrbohE (Fig. 6B).
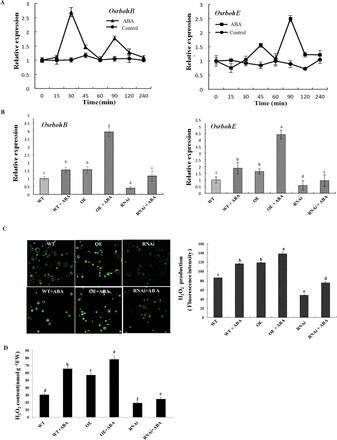
ZFP36 regulates the expression of NADPH oxidase genes and the production of H2O2 in ABA signalling. (A) Time course of changes in the expression of OsrbohB and OsrbohE in leaves of rice plants exposed to ABA treatment. The rice seedlings were treated with 100 μM ABA for various times as indicated, and the relative expression levels of OsrbohB and OsrbohE were analysed by real-time quantitative RT–PCR. (B) The expression of OsrbohB and OsrbohE in the leaves of ZFP36-OE plants, ZFP36-RNAi plants, and WT plants. The rice seedlings were treated with 100 μM ABA for 30min (OsrbohB) or 90min (OsrbohE), and the relative expression levels of OsrbohB and OsrbohE were analysed by real-time quantitative RT–PCR. (C) The production of H2O2 in the protoplasts from ZFP36-OE plants, ZFP36-RNAi plants, and WT plants. The protoplasts were treated with 10 μM ABA (+ABA) or the incubation medium (–ABA) for 5min, and then loaded with H2DCF-DA for 10min. H2O2 was visualized by confocal microscopy (left), and the fluorescence intensity was analysed by Leica IMAGE software (right). (D) The content of H2O2 in the leaves of ZFP36-OE plants, ZFP36-RNAi plants, and WT plants. The rice seedlings were treated with 100 μM ABA for 2h, and the content of H2O2 in leaves was analysed. Values are means ±SE of three independent experiments. Means denoted by the same letter did not differ significantly at P<0.05 according to Duncan’s multiple range test.
In the rice genome, there are 17 MAPK genes (OsMPK genes; Reyna and Yang, 2006). Previous studies have shown that OsMPK1 and OsMPK5 are important components of ABA signalling (Zhang et al., 2012; Shi et al., 2014). Here another three MAPK genes were identified, OsMPK4, OsMPK7, and OsMPK14, which were also induced by ABA treatment (Supplementary Fig. S1 available at JXB online). To investigate whether ZFP36 regulates the expression of these MAPK genes in ABA signalling, ZFP36-OE and ZFP36-RNAi transgenic rice plants and WT plants were used. The changes in the expression of OsMPK genes in the transgenic rice plants and WT plants with or without ABA (Fig. 7) were similar to those in the expression of OsrbohB and OsrbohE (Fig. 6B) and the production of H2O2 (Fig. 6C, D).
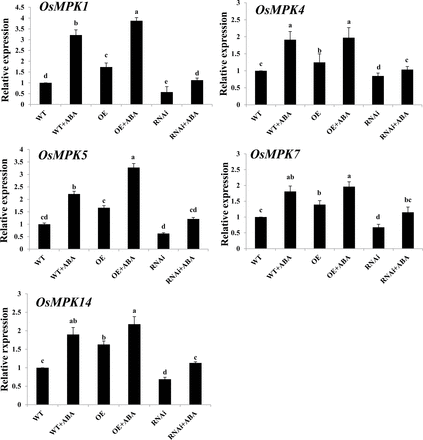
The expression of OsMPK genes in leaves of ZFP36-OE plants, ZFP36-RNAi plants, and WT plants. The rice seedlings were treated with 100 μM ABA for 30min (for OsMPK4, OsMPK5, OsMPK7, and OsMPK14) or 60min (for OsMPK1), and the relative expression levels of OsMPK genes were analysed by real-time quantitative RT–PCR. Values are means ±SE of three independent experiments. Means denoted by the same letter did not differ significantly at P<0.05 according to Duncan’s multiple range test.
The expression of ZFP36 is regulated by OsMPKs in ABA signalling
To investigate whether OsMPKs are also involved in the ABA-induced up-regulation of the expression of ZFP36, a transient RNAi analysis in protoplasts (Zhai et al., 2009), which has been proven to be gene specific (Zhai et al. 2009; Zhang et al. 2012; Cao et al. 2014), was used. In rice protoplasts, RNAi-mediated silencing of OsMPK1, OsMPK4, OsMPK5, OsMPK7, and OsMPK14 substantially decreased the expression of these genes (Fig. 8A), and also decreased the expression of ZFP36 (Fig. 8B–F). Further, the ABA-induced increase in the expression of ZFP36 in the control protoplasts was inhibited by the RNAi silencing of these OsMPK genes (Fig. 8B–F). These results indicate that these OsMPKs are required for ABA-induced up-regulation in the expression of ZFP36.
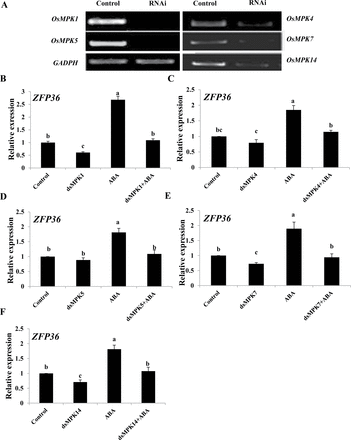
OsMPK genes regulate the expression of ZFP36 in rice protoplasts. (A) The transient RNA interference (RNAi) silencing of OsMPK1, OsMPK4, OsMPK5, OsMPK7, and OsMPK14 in rice protoplasts. The protoplasts were transfected with dsRNAs against OsMPK genes or with water (control) and incubated for 24h. The transient RNAi silencing of OsMPK genes was analysed by semi-quantitative RT–PCR. The glyceraldehyde-3-phosphate dehydrogenase gene (GAPDH) was amplified as a control for amount of a template. (B–F) The transient RNAi silencing of OsMPK1 (B), OsMPK4 (C), OsMPK5 (D), OsMPK7 (E), and OsMPK14 (F) reduces the ABA-induced expression of ZFP36 in rice protoplasts. The protoplasts were treated with 10 μM ABA for 5min, and the relative expression levels of ZFP36 were analysed by real-time quantitative RT–PCR. In (A), experiments were repeated at least three times with similar results. In (B–F), values are means ±SE of three independent experiments. Means denoted by the same letter did not differ significantly at P<0.05 according to Duncan’s multiple range test.
Discussion
Recent studies have revealed that some members of C2H2-type ZFPs in Arabidopsis, such as Zat10, Zat12, Zat7, Zat6, AZF1, AZF2, and AZF3, are involved in the responses of plants to various abiotic stresses (Ciftci-Yilmaz and Mittler, 2008; Miller et al., 2008; Kielbowicz-Matuk, 2012). Zat10 and Zat12, the two widely studied members of the Zat family, have been shown to be induced by multiple abiotic stresses and oxidative stress (Rizhsky et al., 2004; Sakamoto et al., 2004; Davletova et al., 2005a, b; Vogel et al., 2005; Mittler et al., 2006; Koussevitzky et al., 2007; Rossel et al., 2007; Ciftci-Yilmaz and Mittler, 2008; Miller et al., 2008; Kielbowicz-Matuk, 2012). Zat12-overexpressing Arabidopsis plants showed higher tolerance to cold, oxidative, osmotic, and high-light stresses, and knockout Zat12 Arabidopsis seedlings suffered increased sensitivity to these stresses (Iida et al., 2000; Rizhsky et al., 2004; Davletova et al., 2005b; Vogel et al. 2005). Similarly, transgenic plants overexpressing Zat10 were found to be more tolerant to drought, salinity, osmotic stress, heat stress, and high-light stress (Sakamoto et al., 2004; Mittler et al., 2006; Rossel et al., 2007), but, surprisingly, Zat10 knockout and RNAi lines were also more tolerant to osmotic stress and salinity (Mittler et al., 2006). These results suggest that the role of these ZFPs in tolerance of plants to abiotic stresses is complex. This is further supported by AZF1 and AZF2, which are closely related to Zat10 in structure, and were found to regulate salt tolerance negatively in Arabidopsis (Kodaira et al., 2011). In rice, overexpression of the C2H2-type ZFP genes, ZFP252, ZFP245, and ZFP179, increased the tolerance of rice seedlings to drought, salinity, cold, and oxidative stress (Xu et al., 2008; J. Huang et al., 2009; Sun et al., 2010). However, DST (drought and salt tolerance), a C2H2-type ZFP in rice, negatively regulates rice tolerance to drought and salt stresses (X.Y. Huang et al., 2009). In the present study, evidence is provided to show that ZFP36, a novel rice C2H2-type ZFP, is a positive regulator of rice tolerance to water stress and oxidative stress. Transgenic rice plants with overexpression of ZFP36 showed a better phenotype in the lengths of the shoot and root, the shoot fresh weight, and the survival rate under water stress and oxidative stress than the WT plants, and the ZFP36-RNAi plants were more sensitive to water stress and oxidative stress than the WT (Fig. 4). These results indicate that ZFP36 is an important player in the response of rice to water stress and oxidative stress.
It has been shown that Zat10- and Zat12-dependent increased tolerance to abiotic stresses is associated with the up-regulation of antioxidant defence systems. APX1, APX2, and FeSOD1 transcripts were constantly elevated in Zat10 transgenic plants and showed suppressed expression in knockout Zat10 plants during high-light stress (Mittler et al., 2006). Zat12 was shown to be required for APX1 expression during oxidative stress (Davletova et al., 2005a), although overexpression of Zat12 did not induce the expression of APX1 (Davletova et al., 2005b). In rice, overexpression of ZFP245 and ZFP179 enhanced the activities of SOD and peroxidase (POD) under drought, cold, and salt stresses, and increased the tolerance of rice seedlings to oxidative stress (J. Huang et al., 2009; Sun et al., 2010). DST was shown to bind directly to the promoter sequence of peroxidase 24 precursor to regulate the expression of the gene, thus resulting in the reduction of H2O2 accumulation (X.Y. Huang et al., 2009). In the present study, the results showed that under the control conditions, the activities of SOD and APX were enhanced in ZFP36-OE transgenic plants and were decreased in ZFP36-RNAi plants (Fig. 5). Under water stress and oxidative stress, higher activities of SOD and APX were accompanied by lower oxidative damage in ZFP36-OE transgenic plants, and lower activities of SOD and APX were accompanied by higher oxidative damage in ZFP36-RNAi plants (Fig. 5). These results suggest that ZFP36-enhanced tolerance of rice plants to water stress and oxidative stress is related to the increase in the activities of antioxidant enzymes.
ABA, as a stress hormone, plays a key role in the regulation of plant water balance and water stress tolerance under drought and salt stress conditions (Cutler et al., 2010; Hubbard et al., 2010; Umezawa et al., 2010; Fujita et al., 2011). Accumulating evidence indicates that ABA-enhanced water stress tolerance results, at least in part, from the induction of antioxidant defence systems (Jiang and Zhang, 2002a, b, 2004; Miao et al., 2006; Zhang et al., 2006, 2007; Xing et al., 2007, 2008; Neill et al., 2008; Miller et al., 2010; Ye et al., 2011). It was also shown that ABA treatment induced the expression of Zat10, Zat12, AZF1, and AZF2 in Arabidopsis (Sakamoto et al. 2004; Davletova et al. 2005b; Kodaira et al. 2011; Kielbowicz-Matuk, 2012), and ZFP182 and ZFP179 in rice (Huang et al. 2007; Sun et al. 2010; Zhang et al., 2012), suggesting that there might be a link between ABA-induced ZFPs and ABA-induced antioxidant defence under water stress. However, overexpression of AZF1 and AZF2 did not alter the expression of APX1, APX2, and FeSOD1 in Arabidopsis (Kodaira et al. 2011). DST was shown to regulate stomatal closure negatively by direct modulation of genes related to H2O2 homeostasis, but the pathway was ABA independent (X.Y. Huang et al., 2009). Using a transient gene expression analysis and a transient RNAi analysis in protoplasts, a recent study showed that ZFP182 is involved in ABA-induced up-regulation of the activities of antioxidant enzymes in rice (Zhang et al., 2012). Here, another rice C2H2-type ZFP, ZFP36, is reported which is also involved in the regulation of antioxidant defence in ABA signalling. Treatments with ABA and H2O2 induced the expression of ZFP36 (Fig. 2), and H2O2 is required for the ABA-induced up-regulation of the expression of ZFP36 (Fig. 2). Overexpression of ZFP36 in transgenic rice plants enhanced the expression of the antioxidant genes SodCc2 and cAPX and the activities of SOD and APX, and RNAi silencing of ZFP36 in transgenic plants decreased the expression of SodCc2 and cAPX and the activities of SOD and APX (Figs 3, 5). Further, ABA-induced increases in the expression of SodCc2 and cAPX and the activities of SOD and APX were further enhanced in the leaves of the ZFP36-OE plants, but were inhibited in the ZFP36-RNAi plants (Fig. 3). These results indicate that ZFP36 is required for ABA-induced up-regulation in the expression and the activities of antioxidant enzymes.
Previous studies have shown that NADPH oxidase, H2O2, and MAPK are important players in ABA-induced antioxidant defence and that there is a cross-talk between NADPH oxidase, H2O2, and MAPK in ABA signalling (Zhang et al., 2006; Lin et al., 2009). ABA-induced H2O2 production activates MAPKs, which amplify the H2O2 signal by regulating the activity of NADPH oxidase in ABA signalling. However, it is not clear whether C2H2-type ZFPs are involved in the regulation of the cross-talk in ABA signalling. It was reported that the expression of ZFP182 was regulated by OsMPK1 and OsMPK5 in ABA signalling (Zhang et al., 2012). However, ZFP182 did not regulate the expression of OsMPK1 and OsMPK5 induced by ABA. These results suggest that ZFP182 might not be involved in the regulation of the cross-talk involving NADPH oxidase, H2O2, and MAPK in ABA signalling. In the present study, it is shown that ZFP36 is a key component in the regulation of the cross-talk in ABA signalling. On the one hand, the expression of ZFP36 was regulated by H2O2, OsMPK1, OsMPK4, OsMPK5, OsMPK7, and OsMPK14 in ABA signalling (Figs 2, 8), and, on the other hand, ZFP36 regulated the expression of the NADPH oxidase genes OsrbohB and OsrbohE and the production of H2O2, and the expression of OsMPK1, OsMPK4, OsMPK5, OsMPK7, and OsMPK14 in ABA signalling (Figs 6, 7). These results suggest that ZFP36 is a key regulator of ROS signalling in ABA signal transduction. However, it may be asked where the early H2O2 source for the expression of ZFP36 in ABA signalling comes from. In Arabidopsis, it was shown that NADPH oxidases mediate ABA-induced ROS production in guard cells, and AtrbohD and AtrbohF are the major catalytic subunits in this process (Kwak et al., 2003). SNF1-related protein kinase 2 (SnRK2) has been shown to be a positive regulator of the early ABA signalling module (Hubbard et al., 2010; Umezawa et al., 2010). OST1 (Open Stomata 1), a member of the SnRK2 family, was shown to be involved in ABA-induced ROS production, and to function upstream of ROS production (Mustilli et al., 2002). A recent study further showed that AtrbohF interacts with and is phosphorylated by OST1, suggesting that OST1 regulates AtrbohF activity (Sirichandra et al., 2009). However, the physiological importance of the phosphorylation of AtrbohF by OST1 in ABA signalling remains to be determined.
The mechanisms by which ZFP36 regulates the expression of these genes involved in ABA signalling are still undetermined. ZFP36 contains two typical C2H2 zinc finger domains and a DLN-box/EAR-motif located at its C-terminus. Several zinc finger proteins containing a DLN-box/EAR-motif have exhibited transcription-repressive activities in plants, such as petunia ZPT2-3 (Sugano et al., 2003) and Arabidopsis Zat7 (Ciftci-Yilmaz et al., 2007), STZ/Zat10, AZF1, and AZF2 (Sakamoto et al., 2004). Overexpression of AZF1 and AZF2 in Arabidopsis repressed the expression of various genes, including osmotic stress and ABA-repressive genes and auxin-inducible genes (Kodaira et al., 2011). However, the rice ZFP179 (Sun et al., 2010), the chrysanthemum CgZFP1 (Gao et al., 2012), the salt cress ThZF1 (Xu et al., 2007), and the chickpea CaZF (Jain et al., 2009) also have a DLN-box/EAR-motif in their C-terminal end and function as transcriptional activators in yeast. Moreover, both Zat10 overexpressors and loss-of-function mutants display enhanced tolerance to drought and salinity stresses, suggesting that Zat10 plays a dual role as both an activator and a repressor of stress response genes (Mittler et al., 2006). These contrasting data suggest the existence of complex networks of interactions between ZFPs and different partners to regulate the tolerance of plants to water stress and oxidative stress. The exact mechanisms of how ZFP36 up-regulates the expression of the ABA-inducible genes in ABA signalling are under investigation in the authors’ laboratory.
In conclusion, the present data indicate that ZFP36 is required for ABA-induced antioxidant defence and for tolerance of rice plants to water stress and oxidative stress. ABA-induced H2O2 production and ABA-induced activation of OsMPKs up-regulate the expression of ZFP36 in ABA signalling, and the expression of ZFP36 also up-regulates the expression of NADPH oxidase and MAPK genes and the production of H2O2 in ABA signalling, indicating that ZFP36 is an important player in the regulation of the cross-talk involving NADPH oxidase, H2O2, and MAPK in ABA signalling. The results also suggest that ZFP36 is a key regulator of ROS signalling in the signal transduction of ABA, water stress, and oxidative stress.
Acknowledgements
This work was supported by the National Basic Research Program of China (grant no. 2012CB114306), the Fundamental Research Funds for the Central Universities (grant nos KYZ201157 and KYTZ201402), the National Natural Science Foundation of China (grant nos 31070254 and 31271631), the Project Funded by the Priority Academic Program Development of Jiangsu Higher Education Institutions, and the Research Fund for the Doctoral Program of Higher Education of China (grant no. 20130097110025).
References
Comments